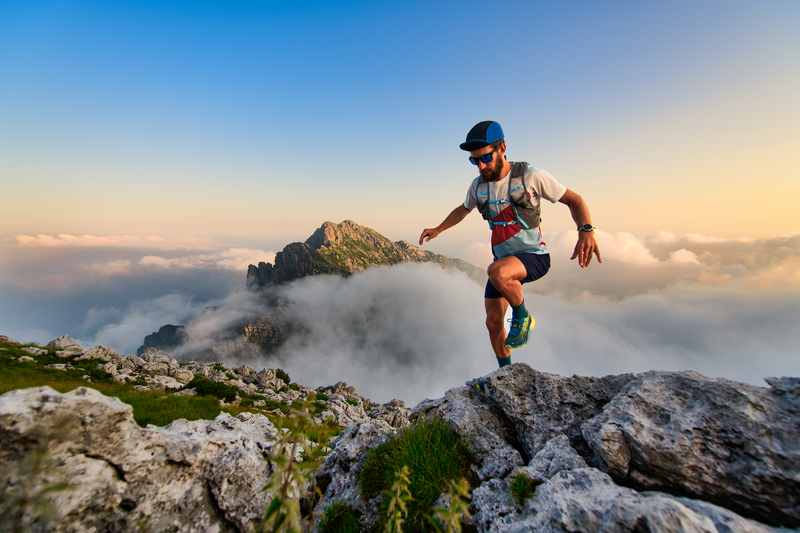
95% of researchers rate our articles as excellent or good
Learn more about the work of our research integrity team to safeguard the quality of each article we publish.
Find out more
REVIEW article
Front. Bee Sci. , 12 October 2023
Sec. Bee Protection and Health
Volume 1 - 2023 | https://doi.org/10.3389/frbee.2023.1272937
This article is part of the Research Topic Horizons in Bee Science View all 10 articles
Varroa destructor is considered one of the greatest threats to the health of the honey bee, A. mellifera. In recent years, there has been a considerable increase in the number of studies on the mite and its interaction with the bee at a cellular and molecular level. However, these studies have also revealed just how complex the interaction is. A significant factor in the virulence of V. destructor is the proteins secreted in its saliva, but only a fraction of these have yet been examined. These proteins can negatively affect the bee’s immune system and promote viruses associated with mite parasitism. Initially, studies on parasitized bees concentrated on immune-related genes, but as more genes of the bees have been examined, it is clear that many other aspects of the bee are affected, such as metabolism and neural functioning. Some of those could be responsible for the detrimental changes in certain behaviors of parasitized bees, which compromises the health of the entire colony. Several viruses are associated with V. destructor parasitism, but it remains difficult to distinguish the effects of the viruses from those of the mite. Reduced immunity in parasitized bees also opens the possibility of secondary microbial infections, adding complexity to the mite-bee interaction. Further complicating studies is the impact of other factors, like agrochemicals, which can alter how V. destructor parasitism affects bee immunity, metabolism, and neural functioning. In addition, differences due to age and sex of the bee being parasitized is a factor that needs to be considered in all studies. While much has been learned in recent years about this complex interaction, the number of unanswered questions only increases.
Graphical Abstract The mechanisms by which Varroa destructor affects honey bee health are not trivial; they are associated with complex interactions with other stressors, including pathogens and agrochemicals, which may impact behaviors, gene expression, and immune responses, compromising the survival and performance of honey bee colonies.
Among the seven to 11 species of Apis, the most important economically managed pollinator worldwide is the western honey bee, A. mellifera, followed much less by the Asian honey bee, A. cerana, which is primarily found in East Asia (Caron and Connor, 2013). Insect pollination is estimated to increase global food production by 15-30%, which can be up to 6,700% compared to self-pollination (Verma and Partap, 1993; Papa et al., 2022). In the USA, honey bee pollination has a value of approx. 11 billion USD (Khalifa et al., 2021) and approx. €153 billion worldwide (Gallai et al., 2009). Pollination increases plant yield and quality (Papa et al., 2022). Vegetables and fruits are the leading types of crops utilizing insect pollination, followed by edible oil crops, stimulants, nuts, and spices (Gallai et al., 2009). Also, honey bees are essential for pollinating wild plants, helping to maintain biodiversity (Papa et al., 2022).
Starting in the winter of 2006-2007, there has been a notable increase in A. mellifera colony losses, particularly in the USA and Europe (vanEngelsdorp et al., 2010). An overwintering loss of colonies of approx. 10% was considered normal, but in the winter of 2006-2007, losses in the USA reached 32% (vanEngelsdorp et al., 2009). Additionally, researchers from other parts of the world, including the Middle East and parts of Asia, reported high rates of colony losses for the same period, in some cases reaching more than 50% (Neumann and Carreck, 2010). More recent losses of colonies have been reported from Spain (36%), Mexico (27.65%), Slovenia (28.9%) (Medina-Flores et al., 2021; Gray et al., 2023), and the USA (51%) (Bruckner et al., 2023a). These high rates of colony losses have been described as Colony Collapse Disorder (CCD) (vanEngelsdorp et al., 2009). With CCD, most worker bees in a colony disappear and leave behind a queen, plenty of food and a few nurse bees to care for the remaining immature bees and the queen (https://www.epa.gov/pollinator-protection/colony-collapse-disorder). CCD is likely due to multiple factors, including arthropod parasites, such as Varroa destructor (Genersch, 2010; Guzman-Novoa et al., 2010), Acarapis woodi (Oldroyd, 2007), and Aethina tumida (Morawetz et al., 2019), and pathogens, such as RNA viruses, like Deformed wing virus (DWV) and Acute bee paralysis virus (APBV), microsporidians, such as Vairimorpha (Nosema) ceranae, and bacteria, such as Mellisococcus plutonius and Paenibacillus larvae (Genersch, 2010; Hristov et al., 2020). In addition, queen failure (Genersch, 2010), weak colonies in autumn (Genersch, 2010; Guzman-Novoa et al., 2010), synthetic acaricides (vanEngelsdorp et al., 2009), deforestation and habitat loss, agrochemicals, and climate change have been reported as contributing factors (Oldroyd, 2007; Khalifa et al., 2021). Among the causes of CCD, V. destructor is often cited as the most important. For example, in the winter of 2008-2009 in Canada, V. destructor was associated with >85% of colony deaths (Guzman-Novoa et al., 2010) between 2012 and 2015 in the Netherlands, 83% of colonies not treated for V. destructor died (van Dooremalen and van Langevelde, 2021), in the winter of 2015-2016 in Austria, losses were 54.6% when V. destructor levels were at 30% (Morawetz et al., 2019).
Originally, Varroa jacobsoni was described as a brood parasite of A. mellifera that had switched host from A. cerana (Oldroyd, 1999). However, V. jacobsoni was a complex of at least two species, V. jacobsoni and a new species, V. destructor (Anderson and Trueman, 2000). After switching hosts, V. destructor spread relatively rapidly, reaching Europe by the early 1970s, and worldwide by approx. 2000 (Traynor et al., 2020). One reason for its rapid spread is that A. mellifera has lower individual behavioral defenses against the mite than its original host, A. cerana. These defenses include grooming, hygienic behavior, varroa sensitive hygiene (uncapping and removal of infested brood), entombing of infested brood, and inhibition of mite fertility (Rath, 1999; Traynor et al., 2020; Grindrod and Martin, 2023).
Both V. jacobsoni and V. destructor can be divided into several variants. Based on the sequence of the mitochondrial DNA cytochrome oxidase subunit I (COI/CO-I/COXI), there were 12 haplotypes of V. jacobsoni and four of V. destructor in A. cerana. In contrast, there were two haplotypes (Korea and Japan-Thailand) of V. destructor in A. mellifera (Anderson and Trueman, 2000). The Korea haplotype is the most virulent and common worldwide, while the less virulent Japan-Thailand haplotype is limited to Japan, Thailand, French Guyana, Chile, and Brazil (Traynor et al., 2020). However, more haplotypes or sub-haplotypes have been found by analyzing additional mitochondrial sequences. By examining sequence variation in cytochrome B (CytB) in addition to COXI, Lin et al. (2021) identified two variants for the Korea haplotype in China with A. mellifera, and Gajić et al. (2019), found five variants of the Korea haplotype in Serbia with A. mellifera. By examining sequence variation in cytochrome c oxidase subunit III (COX3), ATP synthase subunit 6 (ATP6), CytB, and COX1, Navajas et al. (2010) found three variants of the Korea haplotype and four variants of the Japan-Thailand haplotype, both in northeast Asia with A. mellifera. However, with that same set of genes, Ogihara et al. (2020) did not find any variation within the Korea haplotype in Japan.
The amount of sequence differences between the genes used to define V. destructor haplotypes can vary considerably. Navajas et al. (2010) failed to detect any single nucleotide polymorphisms (SNPs) in CytB within the Korea haplotype of V. destructor parasitizing A. mellifera in Asia, and all the variation was detected with COXI. For the Korea haplotype in Turkey, the genetic distance between V. destructor samples was greatest for COX3 (0.55%), followed by CytB (0.06%), and none for ATP6 (0.00%) (Koç et al., 2021). For V. destructor in Serbia, COXI and CytB revealed SNPs in 51.8 and 16.3% of the samples, respectively (Gajić et al., 2019). For the Korea haplotype in Japan, Ogihara et al. (2020) found no variation in ATP6, COXIII, and CytB among 15 apiaries. Thus, it appears that COX1 and COXIII are generally better for distinguishing haplotypes/sub-haplotypes. However, an examination of V. destructor from Argentina with COX1, NADH dehydrogenase subunit 4 (ND4), subunit 4 L (ND4L), and subunit 5 (ND5) showed that the ND4 was best as it could reveal two Korea sub-haplotypes (Muntaabski et al., 2020). Thus, there may be other sequences that can provide better differentiation of V. destructor haplotypes.
Another approach to determining variation among V. destructor is to examine polymorphisms in microsatellites. Analysis of the V. destructor transcriptome revealed 27,775 potential microsatellite loci, and among 60 randomly selected microsatellite loci, six were confirmed to be polymorphic in V. destructor from five locations in China (Duan et al., 2020). Thus, there are many possible microsatellites in V. destructor, but only a fraction may show polymorphisms. For samples of the Korea haplotype from France, Chile, USA, and Philippines, only two of 20 microsatellites revealed variability (Solignac et al., 2005). For samples from Madagascar, three of 11 microsatellites showed polymorphisms, one with two alleles, another with three, and the last one with four alleles (Rasolofoarivao et al., 2017). For samples from China, five of six microsatellites were polymorphic, and four showed polymorphisms with nine to 16 alleles within two Korea sub-haplotypes (Lin et al., 2021). Greater success can be obtained if V. destructor microsatellites are chosen that were previously shown to be polymorphic. Dynes et al. (2016) used 10 microsatellites showing polymorphisms in earlier papers and found all showed polymorphism at the apiary and colony level in the USA. The same approach was used to select six microsatellites, all of which were highly polymorphic for Philippine and Vietnam samples belonging to the Korea haplotype (Beaurepaire et al., 2015). However, Strapazzon et al. (2009) used some of the same microsatellites as Dynes et al. (2016) and Beaurepaire et al. (2015), and none of the four chosen showed polymorphisms within the Korea or Japan haplotypes in two locations in Brazil.
Genetic variability of V. destructor has been compared in a variety of ways, mostly by sequencing mitochondrial genes or microsatellite length polymorphisms. Ultimately, the best approach is full genome sequence comparisons. The genome of V. destructor is approx. 565 Mbp, like many other Acari (Cornman et al., 2010). As sequencing technology continues to advance, it should become more feasible to compare complete V. destructor genomes (Hasegawa et al., 2021). Even a limited comparison between complete genomes of samples could be useful in identifying better markers, such as sequences with the highest number of SNPs or microsatellites with the most significant length variation. As more markers are developed to distinguish sub-haplotypes, like those within the Korea haplotype, it will also be important to compare their virulence to better understand how that varies within a haplotype. Also, one factor that has not been considered much in these studies is sampling time. It appears that mite population can shift considerably over time. The number of alleles varied over a brood season in Germany when examined with seven microsatellites (Beaurepaire et al., 2015).
The life cycle of V. destructor can be divided into two phases, the dispersal or phoretic phase and the reproductive phase. In the phoretic phase, V. destructor females adhere and parasitize adult bees, which enable them to be transported to worker or drone brood cells where they reproduce, and to spread between colonies on parasitized robber or drifting bees (Boecking and Genersch, 2008). The reproductive phase involves V. destructor females entering cells of bee larvae before they are capped (5-day-old instar bee larvae). Once inside the cell, the female mite hides underneath the larva´s food until the cell is capped (De Jong et al., 1982). Drone cells appear to be preferred as they were invaded 11.6 times more frequently than worker cells (Boot et al., 1995). During the molt of the bee prepupa (48 h after cell capping), the mother mite, also known as the foundress mite, prepares a feeding site for her daughters on the bee pupa by puncturing the prepupa’s cuticle at the sternite of the second abdominal segment of drone pupae or in the mesothorax of worker pupae. Following penetration by the mite’s toothed chelicerae and serrated-edged corniculi to form a channel in the bee’s cuticle, a pharyngeal pump is used to feed on the bee’s internal fluids at 4.5 cycles per second with each feeding event lasting approx. 10 seconds separated by approx. 2 minutes (Li et al., 2019). Bee fluid is extracted from the feeding site by the foundress mite and her progeny throughout the bee’s pupal phase (Kanbar and Engels, 2003). Also, the foundress mite establishes a fecal accumulation site on the wall of the cell, where the mating of her offspring also takes place (Donzé and Guerin, 1994).
Approximately 60 to 70 h after the cell is capped, the foundress lays her first egg, which is unfertilized and develops into a haploid male (Rehm and Ritter, 1989). After this, the foundress lays 2 to 5 fertilized eggs at about 30 h intervals between them, and these eggs become females. The developmental period of V. destructor from egg to adult is 5.8 days for females and 6.6 days for males. Mating starts approximately 230-280 h post cell capping with female mites reaching sexual maturity 10 to 20 h after the males (Donzé and Guerin, 1994). Males fertilize a sister or sexually mature female mite from a different foundress mite, and the female stores the semen in her spermatheca. A female has to mate at least four times to obtain sufficient spermatozoa to achieve 1.6 to 1.7 reproductive cycles (Ifantidis, 1983). A male can fertilize an average of 3.75 females in a worker cell but 7.5 females in a drone cell since the metamorphosis period is longer in drone cells (Donzé et al., 1996). When workers or drones hatch from their cells, V. destructor female progeny leave the cells attached to their hosts. In contrast, progeny males remain in the cell where they starve to death since their mouthparts (chelicerae) are modified to perform sperm transportation into the female receptacle (oviduct II) and thus are unable to feed (Alberti and Hänel, 1986).
A key element of the virulence of V. destructor to bees is its saliva. Typically, a damaged cuticle and epidermis of an insect will heal preventing loss of hemolymph, but puncture wounds caused by V. destructor remain open (Kanbar and Engels, 2003). There were at least 15 different proteins detected by SDS-PAGE and electroblotting in the saliva of V. destructor, and when incubated with hemocytes of the caterpillar, Lacanobia oleracea, the saliva damaged the hemocytes and suppressed their ability to extend pseudopods and form aggregates, which are needed for wound healing (Richards et al., 2011). However, a much greater number (356 proteins) were detected in the mite saliva by SDS-PAGE, gel sectioning and nano-liquid chromatography coupled to mass spectrometry (Zhang and Han, 2019). Saliva was toxic to the larvae of A. cerana workers, and some of the proteins in it were potential virulence factors, such as lysophospholipase for membrane destabilization, antimicrobial factors, such as lysozyme for bacterial cell wall degradation, nutrient utilization factors, such as dipeptidyl peptidase III for ingesting host erythrocytes, antioxidant/oxidation–reduction factors, such as thioredoxin peroxidase for hydrogen peroxide breakdown, and detoxification factors, such as sulfotransferase for xenobiotic elimination. Another protein in the mite’s saliva is a chitinase (Vd-CHIsal) related to chitinases of parasitic arthropods (Becchimanzi et al., 2020). The gene encoding it was highly expressed in mite salivary glands and silencing it reduced mite survival. It may be essential for hydrolysis of the bee’s chitin keeping the wound site open and anti-microbial activity attacking the cell wall of any opportunistic bacteria near the wound site. Enzyme activities of cholinesterases, carboxylesterases and phosphatases were also detected in the secretion products of V. destructor, presumably in the saliva, that could enhance virulence by causing cell lysis and degrading bee tissues (Dmitryjuk et al., 2014). Perhaps the clearest example of a saliva protein being involved in mite virulence is Varroa toxic protein, which is lethal to A. cerana worker larvae and pupae, but not A. mellifera worker larvae and pupae. Also it was not toxic to A. cerana worker adults and drones (Zhang and Han, 2018). Considering the significant number of proteins, in the saliva, it will be important to conduct more studies involving gene silencing to assess their importance.
Much of the literature about factors secreted by V. destructor is related to honey bee-associated viruses. In V. destructor, peptides were detected from Varroa destructor Macula-like virus (VdMLV), DWV and ABPV (Erban et al., 2015). Lack of detection of non-structural proteins compared with high amounts of structural proteins suggested that the viruses did not replicate in the mite but more likely accumulated in the mite gut from hemolymph feeding. Santillan-Galicia et al. (2008) also did not find that DWV replicated in V. destructor since there was no specific antibody binding to DWV in any mite tissues, and DWV was only detected in the mite’s midgut lumen in structures resembling fecal pellets. However, the variant DWV-B was able to infect the mite’s intestinal epithelium and salivary glands, while variant DWV-A could not infect the mite (Gisder and Genersch, 2021). DWV was detected among the mite’s saliva proteins, but other viruses, APBV, Black queen cell virus (BQCV), Chronic bee paralysis virus (CBPV), Israeli acute paralysis virus (IAPV) and Sacbrood virus (SBV), were not (Zhang and Han, 2019). However, another study reported SBV and Kashmir bee virus (KBV) in mite saliva (Shen et al., 2005). Therefore, at least DWV-B, and perhaps other viruses, can be transmitted in mite saliva.
In addition to transmission in saliva, DWV in bees can be affected by V. destructor parasitism. Mite parasitized brood can have overt DWV infections with deformed wings, general paralysis, discoloration, and bloated abdomen compared to covert DWV infections where none of those symptoms appear (Martin and Brettell, 2019). In workers, DWV was found in the thorax and abdomen, but not the head with covert infections, whereas the virus was spread throughout the body in overt infections at elevated levels (Yue and Genersch, 2005). The saliva of V. destructor may be a factor in triggering overt infections as it stimulated the appearance of deformed wings in A. mellifera adults in the presence of DWV (Zhang and Han, 2019). Mite parasitism suppressed bee immunity based on reduced expression of genes for antimicrobial peptides and immunity-related enzymes allowing for increased DWV replication (Yang and Cox-Foster, 2005). One explanation for this could be Varroa toxic protein in the saliva, which was not toxic to A. mellifera, but increased DWV levels and the subsequent development of deformed-wing symptoms in adults (Zhang and Han, 2018). Another explanation is that V. destructor suppresses the immune systems by feeding on the fat body of the bee mechanically removing a tissue that is responsible for immune responses (Galbraith et al., 2015; Ramsey et al., 2019). Mite reproduction was also increased by DWV infection as the virus adversely impacted the bee’s immune responses by interfering with NF-κB signaling (Di Prisco et al., 2016). However, proteome changes in the bee during interactions with V. destructor and DWV showed that they were mostly due to the mite rather than the virus (Erban et al., 2019). The effects of the mite and DWV were both cooperative and antagonistic. Opposite effects included the mite and DWV activating and suppressing NF-κB signaling, respectively, while cooperative effects included both the mite and DWV increasing p53-induced apoptosis, hyperactivation of the JAK/STAT pathway and disruption of p53-BCL-6 feedback. Another way that V. destructor could affect viruses in bees is through hemolymph removal during feeding as loss of increasing volumes of hemolymph from bees increased DWV densities by destabilizing viral immune control (Annoscia et al., 2019). Hemolymph removal favors the extraction of antiviral molecules, triggering greater viral replication. While some of the studies do conflict, it is clear that the relationship between the virulence of the mite and honey bee-associated viruses is more driven by the mite and that possibly both mite saliva proteins and physical removal of hemolymph are involved.
The above review of the life cycle and virulence of V. destructor shows that it is highly damaging to bees both directly and indirectly. Effects on individual bees include decreased body weight, lifespan, water content, immune gene expression, neural processes, learning and behaviors, such as flying and orientation (Noël et al., 2020). One of the most common measurements in studies of the effects of the mite on individual bees is the bee’s weight, and thus can be compared between multiple studies. For newly emerged bees without deformed wing symptoms, Bowen-Walker and Gunn (2001) found that non-parasitized bees weighed 5% more than parasitized bees inoculated with one mite per capped brood, but this increased to 7% more than bees with three mites per capped brood. For newly emerged bees with deformed wing symptoms, the impact was greater with 12% more weight for non-parasitized bees than those with one mite per capped brood, and 17% more than those with three mites per capped brood. Similar results were obtained by Yang and Cox-Foster (2007) where newly emerged non-parasitized bees weighed 5 and 8% more than those with one or three mites per capped brood, respectively, without deformed wing symptoms, and 11, 21 and 27% more with one, three or 10 mites per capped brood with deformed wing symptoms. Annoscia et al. (2012) results without deformed wing symptoms were similar with non-parasitized newly emerged bees weighing 8 and 12% more than those with one or three mites per capped brood, respectively. However, with deformed wing symptoms, the weight of non-parasitized newly emerged bees was 4% and 13% higher than those with one or three mites per capped brood, respectively, indicating that deformed wing symptoms did not greatly affect weight reductions. Other studies have only examined parasitized bee weight without deformed wing symptoms. These include van Dooremalen et al. (2013) with non-parasitized bees at seven days of post emergence having 5% more weight than those with one or two mites per capped brood; Strauss et al. (2016) with non-parasitized bees at emergence having 7 and 15% more weight than those with one or with two to three mites per capped brood, respectively; Morfin et al. (2020a) with non-parasitized bees at emergence having 8% more weight than those with one mite per capped brood; and Yang et al. (2021) with non-parasitized bees at emergence having 17% greater weight that those with two mites per capped brood. A summary of studies of the effect of V. destructor parasitism of brood on weight of the emerged adult indicates that weight loss always increased with more mites per capped brood, which was relatively consistent, mostly with non-parasitized bees being 5-8% heavier than those with one mite and 15-20% than those with two to three mites. However, more studies are needed to compare the effect of DWV infections on weight loss, particularly by quantifying the amount of DWV. In contrast to brood parasitism, only Morfin et al. (2020b) examined weight loss where mites were placed on adults, showing that non-parasitized bees weighed 7% more than those with one mite per adult bee at 21 days of parasitism with no deformed wing symptoms.
Another factor commonly measured for the effect of V. destructor on individual bees is bee survival, and thus can be compared between studies. The median lifespan of bees without deformed wing symptoms after emergence was 13.6 d and 8.9 d with one or two mites per brood, respectively, compared to 27.6 d for non-parasitized bees (De Jong and De Jong, 1983). The time of 75% cumulative dead after emergence with one mite per capped brood was 18.5 d without deformed wing symptoms and 1.1 d with deformed wing symptoms compared to 20.7 d for non-parasitized bees (Yang and Cox-Foster, 2007). The average lifespan of emerged bees with one and three mites per capped brood was 16 or 8.5 d, respectively, without deformed wing symptoms, and 7.5 or 4 d, respectively, with deformed wing symptoms compared to 19 d for non-parasitized bees (Annoscia et al., 2012). The average lifespan of emerged bees with two mites per capped brood was 8.5 d without deformed wing symptoms compared to 14.4 d for non-parasitized bees (Reyes-Quintana et al., 2019). Similar to studies on weight, there has been little examination of the effects of mite parasitism on adult bee lifespans. Morfin et al. (2020b) did not calculate the average lifespan but showed that 20% of adult bees with one mite per bee survived by 21 days compared to 83% for non-parasitized adult bees. All these studies show that mite parasitism of brood reduced the lifespan of the bee with much greater effects with DWV symptoms, although the effect on adults appears much more limited. Also, the degree of impact of V. destructor parasitism on bee lifespan is much greater than that on weight.
In the above studies, weight reductions due to mite parasitism was hypothesized to be due to hemolymph loss (Bowen-Walker and Gunn, 2001; Annoscia et al., 2012; Morfin et al., 2020a), fat body consumption (Morfin et al., 2020a), DWV symptoms (Bowen-Walker and Gunn, 2001; Morfin et al., 2020a), reduced bee water content (Annoscia et al., 2012; Strauss et al., 2016), and reduced bee protein content (van Dooremalen et al., 2013). Reduced lifespan due to mite parasitism were proposed to be due to piercing of cuticle and membranes (Morfin et al., 2020a), suppression of bee immunity (Yang and Cox-Foster, 2007; Reyes-Quintana et al., 2019; Morfin et al., 2020b), up-regulation of genes associated with cardiac pathology, and down-regulation of genes associated with glycolysis/glucogenesis (Morfin et al., 2020b), and DWV symptoms resulting in bees being unable to feed themselves (Yang and Cox-Foster, 2007; Annoscia et al., 2012; Reyes-Quintana et al., 2019).
Hemolymph is the circulating fluid of insects, composed mostly of water (approx. 20-50% of total water in an insect), but it also contains a variety of chemicals, such as inorganic compounds, low molecular weight organic compounds and proteins, as well as circulating cells called hemocytes (Kanost, 2009). All of these are important for the proper functioning of an insect’s metabolism, such as ions to help maintain pH, trehalose as an energy source, glycerol, and sorbitol to lower the freezing point for cold damage protection, and proteins to maintain osmotic pressure, transport lipids, provide food storage, and contribute to the immune response. Also, hemolymph transports nutrients from the digestive system, and removes cellular wastes (Caron and Connor, 2013). The physical loss of hemolymph during V. destructor parasitism could negatively affect any of these functions to varying extents. For example, body water content of bees was negatively correlated with the degree of mite parasitism (Bowen-Walker and Gunn, 2001), and total hemolymph basic proteins decreased 34% with one to three mites and 56% with four to six mites per larva (Glinski and Jarosz, 1984).
Insect hemocytes are divided into plasmatocytes, granulocytes and lamellocytes based on their morphology/presumed function with plasmatocytes (a pleiomorphic hemocyte) being the most abundant (Ling and Yu, 2006; Marmaras and Lampropoulou, 2009). Plasmatocytes, granulocytes and lamellocytes are part of the immune response involved in phagocytosis of microbes, nodule formation where multiple hemocytes aggregate to trap microbes, encapsulation where hemocytes attach to the surface of a larger parasite and form a multilayered capsule killing the parasite. In addition, plasmatocytes aggregate to seal wounds preventing hemolymph loss, and granuloctyes synthesize an extracellular matrix that covers tissues exposed to the hemolymph (Strand, 2008). Newly emerged bees with V. destructor had up to 90% reduction in the number of normal hemocytes compared to the non-parasitized control, but by 8 to 25 days post-emergence, there were no significance differences from the non-parasitized control (Amdam et al., 2004). Another study of newly emerged bees with V. destructor showed approx. 25% reduced hemocyte concentration when exposed to one mite per capped pupa (Morfin et al., 2020a). For nurse bees from colonies parasitized with V. destructor, there was an approx. 33% decrease in hemocyte concentration (Belaïd and Doumandji, 2010). For adult worker bees in cages with V. destructor, there was an approx. 50% reduction in total hemocyte concentrations by 12 hours of parasitism, although the numbers recovered by 24 and 48 hours (Koleoglu et al., 2018). For drones taken from infested colonies, hemocyte concentrations were decreased by 47%, 13% and 65% for infested larvae, pupae, and adults, respectively (Salem et al., 2006). While all these studies show that parasitism reduces hemocytes in bees, there is a considerable range in the level of reductions, and evidence that the reductions can be transient.
Reductions in hemocyte concentrations by V. destructor parasitism as well as down-regulated immune gene expression (Yang and Cox-Foster, 2005; Erban et al., 2019) may help explain the ability of pathogens or potential pathogens to invade the hemolymph of mite parasitized bees. The pathogen, V. ceranae, is normally restricted to the bee’s digestive system, but its DNA was found in 68% of the hemolymph samples of bees parasitized by V. destructor (Glavinić et al., 2014). Serratia marcescens was found in the hemolymph of more than 90% of dying worker bees in winter hives and about half of the V. destructor in those colonies (Burritt et al., 2016). The bacterium was not generally found on the surface or digestive tract of dying bees, and it was not found in healthy bees. Sequencing the genome showed that this was a novel strain with unique genes related to those of certain bacterial insect pathogens, and there was evidence that the bacterium could be reducing hemocyte populations and permeabilizing some bee organ membranes. Varroa toxic protein could be involved as injection of it into A. mellifera larvae resulted in the isolation of Stenotrophomonas maltophilia and Staphylococcus aureus, while bacterial colonies were not detected from the larval hemolymph with injection only (Balakrishnan et al., 2021). The authors suggested that those species of bacteria were present in the hemolymph of healthy larvae at undetectable levels and Varroa toxic protein stimulated their growth. While none of these studies have shown that the microbes detected are causing diseases in the hemolymph, they do indicate that microbes that normally would be controlled by the bee’s cellular immunity now have the opportunity to proliferate and spread in the hemolymph during V. destructor parasitism.
While it is generally assumed that mite uptake of hemolymph was related to consumption of hemocytes as their numbers declined with parasitism, there is evidence that fat body cells are fed upon. The mite preferred to feed on the ventral rather than the dorsal region of the bee’s metasoma providing access to the fat body, and degraded fat body cells were found directly underneath the intersegmental membrane at the feeding site, likely a result of extra-oral digestion by the mite (Ramsey et al., 2018; Ramsey et al., 2019). Marking hemolymph and fat body in honey bees with different fluorescent stains revealed that the guts of mites contained the stain fluorescence of the fat body rather than the hemolymph (Ramsey et al., 2019). Also, mites feeding on the fat body lived longer and produced more eggs than those feeding on the hemolymph. All of this indicated that fat body cells were being fed upon rather than hemolymph following extra-oral digestion by the mite creating dissolved semisolid fat body tissue in the bee. Ramsey et al. (2019) proposed that the diverse symptoms of V. destructor parasitism would be consistent with the loss of fat body tissue during feeding as the bee’s fat body is important for immunity, detoxification, nutrient storage, and other functions.
There are other bee organs affected by mite feeding, such as the hypopharyngeal gland. The size of the hypopharyngeal gland in parasitized nurse bees was reduced with fewer vacuoles suggesting decreased secretion by the gland, which could be detrimental to brood development and queen nutrition (Pinto et al., 2011). Similarly, newly emerged bees with mite parasitism of the brood showed reduced hypopharyngeal gland size, which could result in bees with less secretion of royal jelly and brood food as well as less secretion of antimicrobial enzymes reducing immunity (Bruckner et al., 2023b). Pinto et al. (2011) proposed that reduced hypopharyngeal gland size was due to direct parasitism and viral infections. Other evidence that DWV may be involved was the smaller hypopharyngeal glands of bees with deformed wings that also had few small vacuoles but many eosinophilic granules, suggesting increased production of serous secretion, more typical of foragers (Power et al., 2021). Power et al. (2021) proposed that this was an impact of DWV on the gland in both symptomatic as well as asymptomatic bees.
Other organs affected by mite parasitism are the mandibular glands and smaller reservoir glands (Zakaria and Abd El-Wahab, 2004), antennal sense organs (Abd El-Wahab et al., 2006), and flight muscles and mid-gut (Power et al., 2021). Mandibular and smaller reservoir glands were smaller in newly emerged bees with mite parasitism and more severe effects were observed with deformed bees, which could be related to inadequate protein levels during development (Zakaria and Abd El-Wahab, 2004). Mandibular glands are needed to produce pheromones for communication among colony members, and so reduced function would negatively affect the entire colony (Zakaria and Abd El-Wahab, 2004). Mite parasitized bees also have fewer sensilla trichodea and smaller antennal flagellum (Abd El-Wahab et al., 2006). Such a reduction in the antennal sense organs could adversely affect the bee’s behavior with a reduced sense of touch, smell and taste. In addition, mite parasitized bees showed an incomplete development of flight muscles and an inflammation of the midgut and hemocele (Power et al., 2021). There were increased numbers of inflammatory cells (plasmatocytes and granulocytes) and the accumulation of melanin between the midgut villi and the hemocele. These changes were observed in symptomatic and asymptomatic bees infected with DWV. While ABPV is typically associated with DWV in bees and was also found in all samples with altered morphology, it was believed to be less likely responsible as APBV does not trigger an immune response in the bee. The inflammation of the midgut could be related to reduced immunity against microbial infections, consistent with the finding of N. ceranae in the hemolymph samples of parasitized bees (Glavinić et al., 2014).
Finally, the brain of the bee appears to be negatively affected with V. destructor parasitism. The size of the total brain and specific brain regions were reduced by approx. 13% in workers that developed from mite parasitized brood (Lucas et al., 2006). Mite parasitism also dysregulated many brain metabolites, including fatty acids, amino acids, carboxylic acid, and phospholipids, with the greatest changes in linoleic acid, propanoate, glycine, serine, and threonine metabolism (Wu et al., 2017). The result would be reduced brain function negatively affecting the processing of sensory inputs as well as outputs like bee behaviors, which would adversely impact both individuals and colonies. Other evidence for reduced brain function is altered gene expression of parasitized capped brood related to decreased dopamine production and suppression of the prevention of neural degeneration, which could result in greater neuronal apoptosis during aging causing cognitive impairment (Navajas et al., 2008). Mite parasitism of adult bees also down-regulated expression of several neural genes indicating disruption of synaptic function and reduced ability to counteract neurodegeneration (Morfin et al., 2020b; Morfin et al., 2020c) These negative changes in the bee brain during mite parasitism may contribute to the behavioral changes in parasitized bees reviewed below.
One of the highest impacts of V. destructor on individual bees is the effect on the humoral immune system. Activation of humoral immunity relies on changes in protein levels regulated by intracellular signaling pathways (Morfin et al., 2021). These signaling pathways include Toll, Immune deficiency (Imd), Janus kinase-signal transducer and activator of transcription (JAK/STAT), and c-Jun N-terminal kinase (JNK). The activation of any of these pathways culminates in the synthesis of antimicrobial compounds, mainly antimicrobial peptides (AMP), defensive enzymes, and complement-like proteins (Morfin et al., 2021).
Several studies have found differential immune gene expression in developing and adult honey bees with mite parasitism, either showing or not showing symptoms of viral infection. For example, Yang and Cox-Foster (2005) found differences in gene expression between bees with no signs of wing deformity and no mites, mite-parasitized bees with normal wing development, and mite-parasitized bees with deformed wings due to DWV infection. When these bees were challenged with Escherichia coli, immunosuppression was detected by the downregulation of hymenoptaecin, which was not observed in non-parasitized control bees (Supplementary Table 1). Navajas et al. (2008) compared bee gene expression from colonies that were presumably susceptible or resistant to V. destructor and reported differences in transcriptomic profiles as a response to V. destructor parasitism during the pupal stage. Of the differentially expressed genes that were identified, 15 genes were upregulated and 17 were downregulated. The differentially expressed genes were linked to biological pathways related to embryonic development (perhaps explaining wing deformity), cell metabolism, and immune responses. Another study also found that V. destructor parasitism affected the expression of bee immune-related genes, including the downregulation of defensin and spaetzle in parasitized honey bee pupae (Khongphinitbunjong et al., 2015). Defensin and hymenoptaecin are AMPs synthesized after the activation of the Toll pathway by the protein spaetzle (Rolff and Reynolds, 2009), which suggests that all the proteins regulated by the Toll pathway are suppressed by the mite during bee development. However, in adult bees, Abbo et al. (2017) found an up-regulatory effect of mite parasitism on the humoral immunity genes, defensin and hymenoptaecin, and the cellular immunity gene, eater, indicating that the effects may be quite different between parasitism of developing and adult bees. They also reported a positive correlation between the expression of defensin and hymenoptaecin with levels of varroa mite parasitism. Barroso-Arévalo et al. (2019) found a negative correlation between mite loads and the downregulation of the defense gene defensin and the development gene dorsal, both related to the Toll pathway, but a positive correlation with the up-regulation of the humoral immunity regulator gene, relish. However, Gregory et al. (2005) found that pupae parasitized with one to four mites had lower expression levels of the immunity genes, abaecin and defensin, compared to non-parasitized pupae or pupae parasitized with five to six mites, suggesting a non-linear expression pattern with respect to the number of mites parasitizing the bees. However, not all studies have reported immunosuppression during parasitism. Kuster et al. (2014) found immunostimulatory effects of varroa parasitism in developing bees based on the expression of 10 immune related genes (including defensin-2 and hymenoptaecin) at 24, 72, 120, 192, and 240-hours post cell capping (hpc). However, the upregulation of immune-related genes only occurred at some hpc and with ≥3 mites per cell, indicating an inconsistent effect of V. destructor on immune responses in developing bees. Their study found that experimental wounding also increased the expression of immune genes, suggesting that the mechanical injury caused by the mite’s feeding is related to changes in immune gene expression. Likewise, Koleoglu et al. (2017) found that the effect of V. destructor parasitism and the injection of a saline buffer had similar effects, but in this case resulting in downregulation of the immune-related genes hymenoptaecin and defensin, in developing and adult honey bees, suggesting that the effects of the mite on immune responses could be, at least in part, related to the wounds caused by the mite or the needle used to inject the buffer. In contrast to all these studies, Aronstein et al. (2012) found no significant effects of the mite on the expression of the immune genes defensin-1, abaecin, and hymenoptaecin studied in parasitized bees at different stages of development.
In addition to cellular immune responses mediated by hemocytes that circulate in the hemolymph of bees, there are a number of genes related to cellular immunity (Morfin et al., 2021). Besides a reduction in hemocyte numbers, Koleoglu et al. (2018) found that the mite caused a downregulation of AmPPO, a prophenoloxidase gene whose expression was directly correlated with hemocyte count. Morfin et al. (2020a) found that a reduction of uncharacterized hemocytes in newly emerged bees parasitized with V. destructor during their pupal stage occurred along with 21 upregulated and 45 downregulated differentially expressed genes, that included biological pathways linked to leucocyte transendothelial migration and phagosomes, linking the effect of V. destructor on cellular immune responses. Furthermore, Zaobidna et al. (2015) found mostly down-regulatory effects of V. destructor parasitism on the expression of proPO, and a reduced enzymatic activity of prophenoloxidase in most of the developmental stages of parasitized honey bee workers and drones. The cleavage of prophenoloxidase by serine proteases leads to activation of phenoloxidase, which in turn allows the production of melanin. Melanin and the reactive oxygen and nitrogen species formed during its synthesis are toxic to parasites, bacteria, fungi, and viruses (Morfin et al., 2021). Thus, not only are hemocyte numbers reduced in response to V. destructor parasitism, gene expression and enzyme activities related to them are also suppressed. However, it is not yet clear if such reductions are simply a result of fewer hemocytes or whether haemocytes are not functioning normally. Studies on the effects of V. destructor on hemocytes need to identify gene expression in the different types of hemocytes as well.
Although it is evident that V. destructor alters gene expression in honey bees, there are clearly inconsistencies among the above studies in the way V. destructor dysregulates the expression of immune related genes. Differences between the studies could be related to the experimental setup, developing versus adult bees, or the levels of other stressors, like viruses. For example, Zaobidna et al. (2017) observed effects on 14 genes related to the Toll pathway in parasitized workers and drones during different developmental stages, finding significantly increased expression of 10 of the 14 genes, including defensin-1 and defensin-2, in larvae, with a slower increase in drones. However, expression was silenced in later life stages showing that the impact of mite parasitism on bee gene expression was sex and life-stage specific.
The effect of mite parasitism on honey bee gene expression in most of the studies above have been limited to three to 14 genes, but transcriptomic studies have allowed the examination of a much broader range of genes shedding light on the complex effects of V. destructor on honey bee health. For example, Doublet et al. (2017) identified 88 upregulated genes and 79 downregulated genes in bees parasitized by V. destructor, and a gene ontology (GO) analysis identified nutrient reservoir activity linked to the down-regulated genes. Differentially expressed immune related genes identified by Doublet et al. (2017) included iap2, rel, tube, and defensin-2, which are part of the Imd and Toll immunity pathways. The involvement of the aforementioned genes in anti-bacterial and anti-fungal mechanisms has been well defined, but most recently their activation in response to viral infections has also been described (McMenamin et al., 2018). However, Doublet et al. (2017) failed to identify transcripts related to the antiviral defense mechanism, RNAi, and as discussed by the authors, this was possibly due to the transient nature of the transcripts and the technical inability of RNA sequencing to detect them. However, they found that vitellogenin (Vg) was down-regulated and malvolio (Mvl) was up-regulated. These genes are involved in behavioral division of labor, indicating a potential effect of V. destructor on behavioral social responses (Alaux et al., 2012; Salmela and Sundström, 2018). Similarly, Amdam et al. (2012) showed lower titres of Vg protein in parasitized worker bees infested during their pupal stage that could be affecting the onset of foraging behavior in parasitized bees. Zanni et al. (2017) identified 1333 differentially expressed genes in honey bees highly parasitized by V. destructor in field and laboratory experiments, and apart from observing higher DWV levels in parasitized bees, they identified dysregulated genes linked to stress responses, immune responses, nervous system function, metabolism, and behavioral maturation. Dysregulated expression of immune genes included up-regulation of PGRP-2 and hymenoptaecin and down-regulation of UNC93, and up-regulation of the neural genes for nicotinic acetylcholine receptors (nAChRa9 and nAChRb2), indicating a possible impact of the mite on both immunity and b behavioral immune responses (discussed in section 11). Using a combined Omics approach (transcriptomics, proteomic, metabolomic, and functional analysis), Kunc et al. (2023) showed an activation of immune responses and sphingolipid metabolism in parasitized 10-day old worker bees, but an inhibition of olfactory recognition and oxidative stress. Their metabolome analysis indicated a decrease in nutrients and energy stores in parasitized bees, which agrees with the mite’s feeding behavior of consuming primarily fat body but also hemolymph (Annoscia et al., 2019; Ramsey et al., 2019). The up-regulated immune response genes by V. destructor identified by Kunc et al. (2023) included apidaecin 1, abaecin, hymenoptaecin, defensin 1, RISC-loading complex subunit TARBP2, dicer, argonaute-2, peptidoglycan recognition protein S2, and beta-1,3 glucan binding protein. However, no down-regulated genes linked to immune response. Down-regulated genes were involved in the maintenance of structural integrity and cell development and included cell wall integrity and stress response component 1, collagen alpha-2(IV) chain like, tubulin alpha chain, and the detoxification genes cytochrome P450 6A1 and cytochrome P450 9e2. Other down-regulated genes were related to metabolic processes including pancreatic triacylglycerol lipase-like, glucose dehydrogenase, and major royal jelly protein 6, and sensory recognition, such as carotenoid isomerooxygenase and chemosensory protein 6. Thus, a quite broad range of impacts were observed. These studies showed the complex effects of V. destructor on different biological pathways (immune and metabolic pathways) that could be interconnected.
Although V. destructor has been considered the main culprit of honey bee colony mortality in the last three decades, most of the conclusions regarding the effect of the mite on honey bee immune responses are based on the expression of a few genes used as molecular markers (Supplementary Table 1). However, this has started to change as more studies have examined the effect of the mite on the immunity and other biological pathways using high throughput transcriptomic, proteomic, and metabolomic studies (Duay et al., 2002; Navajas et al., 2008; Zanni et al., 2017; Morfin et al., 2019; Morfin et al., 2020a; Morfin et al., 2020b; Kunc et al., 2023). Nevertheless, it is apparent that the response of bees differs based on sex and age (Zaobidna et al., 2017), and many of the studies so far conducted have focused on worker bees, but little is known about the effect of V. destructor parasitism on immune mechanisms of queens and drones (Supplementary Table 1). Moreover, studies on relationships between V. destructor parasitism, energetic cost of immune responses, and consequences to other metabolic pathways (like neural processes and reproduction) are warranted. The crosstalk between immune and metabolic pathways, known as immunometabolism, is a new area of research in other animal models (i.e. Mus musculus) (Lercher et al., 2020), and needs to be further explored in bees. Lastly, there are difficulties in determining the sole effect of V. destructor as there are many factors, like viruses and altered neural and metabolic processes, that could be interacting with the effects of varroa mite parasitism. Addressing the sole effects of the mite as well as the synergistic effects from the interaction of the parasite with different stressors (biotic and abiotic) and their influence on different biological processes is not trivial (discussed in section 5). Hopefully the use of high throughput molecular techniques and studies on the description and quantification of biomolecules and their role on different biological pathways and networks will help understand the impact of V. destructor on honey bee health at the molecular level, as well as to help develop therapeutic strategies for varroosis control.
In addition to the host-parasite interaction between V. destructor and A. mellifera, there is also the interaction between the mite and viruses. Several studies have found an increase in viral levels (i.e. DWV and IAPV) in honey bees from V. destructor-parasitized colonies, and also an increase in bee mortality in parasitized and infected bees of those colonies, compared to colonies not parasitized by the mite (Dainat et al., 2012; Francis et al., 2013). Zhu et al. (2022) found that colonies that were treated with the synthetic acaricide Apivar® (containing the active ingredient amitraz) not only had lower mite loads and lower DWV levels compared to untreated colonies, but they also showed higher tolerance (lower mortality) to insecticides such as imidacloprid, cyhalothrin and oxamyl, indicating that V. destructor parasitism may affect tolerance to insecticide exposure. Several studies support the notion that viruses associated with V. destructor have detrimental effects on honey bee health, including immune responses. For example, a negative correlation between DWV levels and the cellular immune responses of melanization and encapsulation has been documented in honey bee brood artificially parasitized with V. destructor (Di Prisco et al., 2016). This correlation resulted from a down-regulatory effect of DWV on Amel\102, a gene involved in cellular immunity, which is regulated by the transcription factor NB-kB of the Toll signaling pathway. Di Prisco et al. (2016) also proposed that DWV increased V. destructor fitness because the proportion of reproducing mites increased as DWV levels in the pupae increased, up to a threshold of 108 DWV genome copies per bee. Conversely, higher DWV levels seemed to negatively impact V. destructor reproduction. Furthermore, mites showed twice the fertility rate (40%) when they parasitized larvae that later emerged with evident signs of wing deformity, compared to mites that parasitized asymptomatic bees (22% fertility rate), suggesting that DWV infections might favor V. destructor fitness. However, confirmation of the levels of DWV in the bees was lacking, and thus, further studies are needed to confirm the claims made by the authors of the study.
Three known variants of DWV that infect honey bees (DWV-A, DWV-B, and DWV-C) are mechanically vectored by V. destructor, but apparently only DWV-B can infect mite tissues (intestinal epithelium and salivary glands), which turns the parasite into a biological vector and a parasite of V. destructor (Gisder and Genersch, 2021). However, very few studies have been conducted on the relationship between DWV variants and V. destructor, and if the interaction with the vector (V. destructor) is affecting the mutation rate of DWV (Regoes et al., 2013). It is possible that variants of DWV will eventually appear that are primarily pathogens of the mite rather than the bee. Variants of Varroa destructor virus (VDV; Iflaviridae), including VDV-2, VDV-3, and VDV-5, seem to selectively infect mites but not bees (Levin et al., 2016; Herrero et al., 2019; Chen et al., 2021). Most of these studies have used RNA extraction and sequencing, which is a technique able to identify the presence of the virus but is not capable of determining if the virus is replicating and damaging tissues of either host. However, there are not many techniques available to confirm the infectivity of viruses in V. destructor and A. mellifera, as there are limited or no commercial cell lines. Identifying negative RNA strands, immunoassays, or in situ hybridization could be the first steps to study virus infectivity (Shen et al., 2005; Yue and Genersch, 2005; Shah et al., 2009). Moreover, the interactions between the different viruses may be a confounding factor. For example, Gregorc et al. (2012) found higher and lower DWV and BQCV transcripts, respectively, in mite parasitized bees, indicating a possible competition between viruses, which should be further explored. Moreover, there are less well studied viruses associated with honey bee and/or mite tissues, such as Bee Macula-like virus (BeeMLV), and De Miranda et al. (2015) suggested that mites are likely a biological vector of this virus based on the identification of sub-genomic RNA, which allowed the detection of actively replicating virus in bee and mite tissues. Other less studied viruses have been identified in V. destructor and bees using high throughput RNA sequencing, like Moku virus and Bee macula virus, and the implications of these viruses on V. destructor and bee health are unknown (Mordecai et al., 2016; Morfin et al., 2022).
Varroa destructor seems to interact with pathogens other than viruses, including the microsporidians Vairimorpha (Nosema) spp., as it appears that honey bee colonies parasitized by the mite tend to have higher Vairimorpha (Nosema) spp. loads (Bermejo and Fernández, 1997; Mariani et al., 2012; Little et al., 2015). Additionally, van Dooremalen et al. (2018) found that colonies parasitized by V. destructor that were exposed to imidacloprid, showed higher Vairimorpha (Nosema) spp. levels, but they found no interactions between the stressors on colony size or colony survival. Contrary to these findings, Ostermann (2003) found no effect of V. destructor on Vairimorpha (Nosema) apis levels, nor an effect of the mite and formic acid treatment on the prevalence of chalkbrood disease (Ascosphera apis) in their experimental colonies.
In recent years, there has been an increasing interest in understanding how the gut microbiome impacts bee health, as evidence suggests that it plays an important role in bee metabolism, immunity, and development (Raymann and Moran, 2018). Parasitized bees showed a reduction in the relative abundance of Bartonella apis and Lactobacillus apis, and an increase of Lactobacillus helsingborgensis, Lactobacillus mellis, and Commensalibacter intestini, in whole bodies compared to non-parasitized bees (Hubert et al., 2017). The effect of V. destructor on the bee gut was greater for bacteria than for fungi, such as V. apis and V. ceranae, or trypanosomes, such as Lotmaria passim, which is interesting because Vairimorpha (Nosema) spp. and L. passim infect the epithelial cells of the bees’ gut, and thus, a more pronounced effect on the microbiome would be expected from these pathogens than from V. destructor. Marche et al. (2019) also found that the abundance of bacterial community in whole bee bodies was altered by mite parasitism, with increased relative abundance of Gammaproteobacteria, Betaproteobacteria, Lactobacillus spp., Bifidobacterium spp., and Brevibacillus laterosporus.
There are a few reports on the interaction of V. destructor parasitism and multicellular organisms attacking bees. For example, a decrease in mite levels in apiaries infested with the small hive beetle (SHB), Aethina tumida, was reported, but no effects resulting from the interaction between V. destructor and SHB were observed on parameters associated to honey bee health like adult bee population, body mass, brood density, or colony weight (Delaplane et al., 2010). More studies are needed to confirm the nature of the interaction between V. destructor and SHB, and the possible consequences on honey bee health.
Abiotic stressors also interact with V. destructor parasitism, impacting honey bee health. Most studies have focused on analyzing the effect of the mite and insecticide exposure on different aspects of bee health, behavior, and neural gene expression. For behavior, negative effects on flight capacity (i.e. bees flying shorter distances) and homing success of forager bees from colonies exposed to neonicotinoid insecticides (i.e. imidacloprid or thiamethoxam) and parasitized by V. destructor have been reported (Blanken et al., 2015; Monchanin et al., 2019). Detrimental effects on memory retention and in the expression of neural related genes, like neurexin (AmNrx-1), in honey bees parasitized by V. destructor and exposed to sublethal doses of neonicotinoid insecticides, like imidacloprid and clothianidin, have been described, which could be responsible for the altered behaviors (Morfin et al., 2020b; Morfin et al., 2020c; Schwartz et al., 2021). The complex formed by pre and postsynaptic proteins regulated by neural genes such as neurexin and neuroligin is essential for neurotransmission and linked to learning processes and memory retention in bees (Biswas et al., 2010). Therefore, these effects of insecticide exposure and V. destructor parasitism affect the performance of behaviors that are essential for colony survival, such as foraging behavior and navigation. An effect of sublethal doses of clothianidin and V. destructor parasitism on the proportion of bees performing intense self-grooming and an effect of both stressors on genes linked to neurological dysfunction was reported by Morfin et al. (2019).
Exposure to imidacloprid and thiamethoxam plus V. destructor parasitism also dysregulated immune related genes in bees at different developmental stages, like a down-regulation of abaecin and defensin-1 in white-eyed pupa, and an up-regulation of PPOact and spaetzle in brown-eyed pupae (Tesovnik et al., 2017; Tesovnik et al., 2019). The mechanism by which neonicotinoid insecticides interact with V. destructor seems complex. Annoscia et al. (2020) found that bees exposed to sublethal doses of clothianidin had lower expression levels of immune related genes (Amel/102 and dorsal 1), higher DWV levels, and lower mite fertility (reproducing mites/total mites). Although the immunosuppressive effect of clothianidin could be exacerbating the effects of the other two biotic stressors (DWV and V. destructor), the nature of the interactions needs to be investigated.
The combined effects of V. destructor parasitism and neonicotinoid insecticide exposure may reduce the body mass of newly emerged bees as well as their subsequent longevity (Straub et al., 2019), and this could be related to the detrimental effect of the parasite and insecticide on hypopharyngeal gland size in workers, and on drone body mass at emergence (Bruckner et al., 2021; Bruckner et al., 2023b). Other studies have found that the interaction between V. destructor and neonicotinoid insecticides impact bee metabolism by dysregulating genes linked to the biosynthesis of secondary metabolites and amino acids, as well as genes involved in fat digestion and absorption (Morfin et al., 2020a; Morfin et al., 2020b).
Most of the studies conducted so far have analyzed the effect of V. destructor in combination with other stressors on worker bees, but it would be interesting to know more about the synergistic impact of these stressors on the reproductive casts and the possible consequences to colony health and fitness. Additionally, the effect of V. destructor in combination with stressors other than insecticides used in agriculture that honey bees are exposed to, like a variety of agrochemicals, such as herbicides, fungicides, and acaricides (including the ones used to treat varroosis), low or high temperatures, drought, etc, warrant investigation to find out if their interaction with the mite impacts honey bee health and colony fitness.
Relatively little is known about how V. destructor affects bee behavior. The best documented behaviors of honey bees in response to V. destructor parasitism are hygienic and grooming behavior, but other behaviors such as foraging and defensive behavior may also be affected by the mite.
Parasitism by V. destructor affects honey bee foraging behavior in different ways. Foragers infested by the mite perform longer foraging trips and many do not return to their hives at all. Parasitized bees also show impaired homing and orientation ability at the hive entrance (Kralj and Fuchs, 2006), which may limit the ability of colonies to collect resources. The fact that bees take long foraging trips or do not return to the hive could be an adaptive behavior of the insects to reduce parasitism load in the hive by leaving the hive or could be due to neurological damage caused by the mite, affecting recognition and responsiveness to environmental stimuli. This last hypothesis was tested by Kralj et al. (2007), who found that bees infested with V. destructor significantly decreased their responses to a reward stimulus (sucrose syrup) when a habituation test was conducted by repeatedly presenting the stimulus to the bees. The parasitized bees also showed a lower response to a scent stimulus than non-parasitized bees, suggesting that mites may interfere with neural transmission that enables learning and memory retention. Morfin et al. (2020c) also showed a detrimental impact of V. destructor on the ability of honey bees to respond to foraging-related stimuli, and possibly other behaviors that require cognitive processing. Studies have also found a down regulatory effect of V. destructor on neural related genes, like AmNrx-1, neuroligin (Am-Nlg-1) and acetylcholinesterase (AmAChE-2) (Morfin et al., 2020b; Morfin et al., 2020c). Likewise, Duay et al. (2002) found that V. destructor-parasitized drones showed decreased flight performance and lower ability to mate with queens compared to non-parasitized drones. Neural disorders because of V. destructor parasitism could also be due to infections caused by viruses transmitted by the mite. For example, DWV and IAPV affect the expression of neural genes and impair the homing ability and learning processes in honey bees (Iqbal and Mueller, 2007; Li et al., 2013). Therefore, the combination of mite parasitism and viral infections could be detrimental to the foraging activity and fitness of honey bee colonies.
Varroa destructor parasitism and reproduction in honey bee brood stimulates hygienic behavior by adult bees. Hygienic worker bees detect and uncap comb cells containing larvae infected with bacteria or fungi, as well as parasitized with V. destructor (Guzman-Novoa and Morfin, 2019). A parasitized larva may be removed from its cell (Boecking and Spivak, 1999; Arathi et al., 2000) or the cell may be posteriorly recapped (Hawkins and Martin, 2021), all of which interrupts the mite’s life cycle. It is well established that hygienic behavior is a heritable trait (Lapidge et al., 2002; Unger and Guzman-Novoa, 2010) and that some honey bee strains are more hygienic than others (Spivak and Reuter, 1998), particularly those expressing the varroa sensitive hygiene (VSH) trait, that allows them to identify cells infested with reproducing mites (Harbo and Harris, 2009). Worker bees that are hygienic can detect V. destructor-parasitized larvae by perceiving with their antennae chemical odorants from the brood such as hydrocarbons and oleic acid (Nazzi et al., 2004; Mondet et al., 2015; McAfee et al., 2018) that elicit hygienic responses in the bees. Some of these compounds have also been identified from V. destructor and DWV (Wagoner et al., 2019), which again, shows that the combination of both pathogens can influence several honey bee behaviors.
Grooming behavior of adult honey bees may also be triggered by V. destructor infestations. Parasitized workers use their legs and mandibles to remove mites from their bodies, sometimes biting and injuring them (Boecking and Spivak, 1999). The presence of V. destructor as well as the wounds inflicted by the mite while feeding irritate the bees, which elicits grooming responses (Morfin et al., 2019) that are governed by neural processes (Hamiduzzaman et al., 2017; Morfin et al., 2023). Grooming behavior is a heritable trait (Arechavaleta-Velasco et al., 2012; Morfin et al., 2020d), and the expression of this behavior varies among different genotypes and strains of honey bees (Arechavaleta-Velasco and Guzmán-Novoa, 2001; Rinderer et al., 2001; Guzman-Novoa et al., 2012; Bąk and Wilde, 2015; Invernizzi et al., 2015). Interestingly, younger daughter mites elicit stronger grooming responses by honey bees than older foundress mites (Kirrane et al., 2012). Perhaps these responses are related with the greater reproductive potential of younger mites or with different chemical signals produced by the mites.
Varroa destructor may also influence defensive behaviors of honey bees. De la Mora et al. (2021) measured the stinging response threshold of V. destructor-parasitized and non-parasitized worker bees by exposing them to a constant electric stimulus while measuring the time that the bees took to sting a leather patch. Parasitized bees stung significantly faster than non-parasitized bees. The authors concluded that the irritation caused by the parasite affected neural processes that made the bees more sensitive and prone to sting. The implications of these results are that colonies infested with the mite may be better adapted to protect their nests from predators, which would be beneficial for their ecological success, but they would also be more difficult to manage by beekeepers for production purposes.
While it is clear that V. destructor affects different honey bee behaviors, these could result in higher or lower mite infestation rates. More research, particularly related to breeding and selection for different behaviors are needed. In addition to better understanding the effects of the mite on bee behaviors, such studies could have practical outcomes for improved health and fitness of honey bee colonies.
Undoubtedly, V. destructor parasitism is one of the main impacts currently affecting A. mellifera worldwide. Although advancements have been made to understand the development of V. destructor parasitism, there is insufficient knowledge on the effects of the parasite on different immune responses in honey bees and the consequences (or interactions) on biological processes, like metabolic pathways. However, recent studies using more comprehensive methods to investigate the effect of V. destructor on molecular processes, like the use of ‘omic’ tools, can help understand this interaction. Nevertheless, they need to be applied to bees of different developmental stages and sexes rather than concentrating mostly on newly emerged bees parasitized as brood. They would also be useful to combine with studies showing behavioral changes. Complicating all studies of V. destructor parasitism is its interaction in different ways with many biotic and abiotic factors. Such interactions are not trivial, especially those with viruses, as separating the effects of viral diseases (e.g DWV) and V. destructor is challenging. While research on the effect of V. destructor on immune pathways, cellular immunity, and metabolic pathways may appear to have limited practical outcomes, it will hopefully lead to the development of therapies and bees that could better fight V. destructor, thus reducing economic losses, which is urgently needed.
NM: Conceptualization, Writing – original draft, Writing – review & editing. PG: Conceptualization, Writing – original draft, Writing – review & editing. EG: Conceptualization, Writing – original draft, Writing – review & editing.
The authors declare that no financial support was received for the research, authorship, and/or publication of this article.
The authors declare that the research was conducted in the absence of any commercial or financial relationships that could be construed as a potential conflict of interest.
The authors EG-N and NM declared that they were editorial board members of Frontiers, at the time of submission. This had no impact on the peer review process and the final decision.
All claims expressed in this article are solely those of the authors and do not necessarily represent those of their affiliated organizations, or those of the publisher, the editors and the reviewers. Any product that may be evaluated in this article, or claim that may be made by its manufacturer, is not guaranteed or endorsed by the publisher.
The Supplementary Material for this article can be found online at: https://www.frontiersin.org/articles/10.3389/frbee.2023.1272937/full#supplementary-material
Abbo P. M., Kawasaki J. K., Hamilton M., Cook S. C., DeGrandi-Hoffman G., Li W. F., et al. (2017). Effects of Imidacloprid and Varroa destructor on survival and health of European honey bees, Apis mellifera. Insect Sci. 24, 467–477. doi: 10.1111/1744-7917.12335
Abd El-Wahab T., Abd T., Zakaria M., Nour M. (2006). Influence of the infestation by varroa mite Varroa destructor on some antennal sense organs of the worker and drone honey bees Apis mellifera L. J. Appl. Sci. Res. 2, 80–85.
Alaux C., Kemper N., Kretzschmar A., Le Conte Y. (2012). Brain, physiological and behavioral modulation induced by immune stimulation in honeybees (Apis mellifera): A potential mediator of social immunity? Brain Behav. Imm 26, 1057–1060. doi: 10.1016/j.bbi.2012.04.004
Alberti G., Hänel H. (1986). Fine structure of the genital system in the bee parasite, Varroa jacobsoni (Gamasida: Dermanyssina) with remarks on spermiogenesis, spermatozoa and capacitation. Exp. Appl. Acarol. 2, 63–104. doi: 10.1007/BF01193355
Amdam G. V., Fennern E., Havukainen H. (2012). “Vitellogenin in honey bee behavior and lifespan,” in Honeybee Neurobiology and Behavior: A Tribute to Randolf Menzel. Eds. Galizia C. G., Eisenhardt D., Giurfa M. (Dordrecht: Springer Science & Business Media), 17–29. doi: 10.1007/978-94-007-2099-2_2
Amdam G. V., Hartfelder K., Norberg K., Hagen A., Omholt S. W. (2004). Altered physiology in worker honey bees (Hymenoptera: Apidae) infested with the mite Varroa destructor (Acari: Varroidae): A factor in colony loss during overwintering? J. Econ. Entomol. 97, 741–747. doi: 10.1093/jee/97.3.741
Anderson D. L., Trueman J. W. H. (2000). Varroa jacobsoni (Acari: Varroidae) is more than one species. Exp. Appl. Acarol. 24, 165–189. doi: 10.1023/A:1006456720416
Annoscia D., Brown S. P., Di Prisco G., De Paoli E., Del Fabbro S., Frizzera D., et al. (2019). Haemolymph removal by Varroa mite destabilizes the dynamical interaction between immune effectors and virus in bees, as predicted by Volterra’s model. Proc. R. Soc B: Biol. Sci. 286, 20190331. doi: 10.1098/rspb.2019.0331
Annoscia D., Del Piccolo F., Nazzi F. (2012). How does the mite Varroa destructor kill the honeybee Apis mellifera? Alteration of cuticular hydrocarbons and water loss in infested honeybees. J. Insect Physiol. 58, 1548–1555. doi: 10.1016/j.jinsphys.2012.09.008
Annoscia D., Di Prisco G., Becchimanzi A., Caprio E., Frizzera D., Linguadoca A., et al. (2020). Neonicotinoid Clothianidin reduces honey bee immune response and contributes to Varroa mite proliferation. Nat. Commun. 11, 5887. doi: 10.1038/s41467-020-19715-8
Arathi H. S., Burns I., Spivak M. (2000). Ethology of hygienic behaviour in the honey bee Apis mellifera L. (Hymenoptera: Apidae): Behavioural repertoire of hygienic bees. Ethology 106, 365–379. doi: 10.1046/j.1439-0310.2000.00556.x
Arechavaleta-Velasco M. E., Alcala-Escamilla K., Robles-Rios C., Tsuruda J. M., Hunt G. J. (2012). Fine-Scale linkage mapping reveals a small set of candidate genes influencing honey bee grooming behavior in Response to varroa mites. PloS One 7, e47269. doi: 10.1371/journal.pone.0047269
Arechavaleta-Velasco M. E., Guzmán-Novoa E. (2001). Relative effect of four characteristics that restrain the population growth of the mite Varroa destructor in honey bee (Apis mellifera) colonies. Apidologie 32, 157–174. doi: 10.1051/apido:2001121
Aronstein K. A., Saldivar E., Vega R., Westmiller S., Douglas A. E. (2012). How varroa parasitism affects the immunological and nutritional status of the honey bee, Apis mellifera. Insects 3, 601–615. doi: 10.3390/insects3030601
Bąk B., Wilde J. (2015). Grooming behavior by worker bees of various subspecies of honey bees to remove Varroa destructor mites. J. Apic. Res. 54, 207–215. doi: 10.1080/00218839.2016.1147791
Balakrishnan B., Wu H., Cao L., Zhang Y., Li W., Han R. (2021). Immune response and hemolymph microbiota of Apis mellifera and Apis cerana after the challenge with recombinant Varroa Toxic Protein. J. Econ. Entomol. 114, 1310–1320. doi: 10.1093/jee/toab047
Barroso-Arévalo S., Vicente-Rubiano M., Puerta F., Molero F., Sánchez-Vizcaíno J. M. (2019). Immune related genes as markers for monitoring health status of honey bee colonies. BMC Vet. Res. 15, 72. doi: 10.1186/s12917-019-1823-y
Beaurepaire A. L., Truong T. A., Fajardo A. C., Dinh T. Q., Cervancia C., Moritz R. F. A. (2015). Host specificity in the honeybee parasitic mite, Varroa spp. in Apis mellifera and Apis cerana. PloS One 10, e0135103. doi: 10.1371/journal.pone.0135103
Becchimanzi A., Tatè R., Campbell E. M., Gigliotti S., Bowman A. S., Pennacchio F. (2020). A salivary chitinase of Varroa destructor influences host immunity and mite’s survival. PloS Pathog. 16, e1009075. doi: 10.1371/journal.ppat.1009075
Belaïd M., Doumandji S. (2010). Effet du Varroa destructor sur la morphométrie alaire et sur les composants du système immunitaire de l’abeille ouvrière. Apis mellifera intermissa. LSJ 11 (1), 84–90.
Bermejo F. O., Fernández P. G. (1997). Nosema disease in the honey bee (Apis mellifera L) infested with varroa mites in southern Spain. Apidologie 28 (3-4), 105–112.
Biswas S., Reinhard J., Oakeshott J., Russell R., Srinivasan M. V., Claudianos C. (2010). Sensory regulation of neuroligins and neurexin I in the honeybee brain. PloS One 5, e9133. doi: 10.1371/journal.pone.0009133
Blanken L. J., van Langevelde F., van Dooremalen C. (2015). Interaction between Varroa destructor and imidacloprid reduces flight capacity of honeybees. Proc. Biol. Sci. 282, 20151738. doi: 10.1098/rspb.2015.1738
Boecking O., Genersch E. (2008). Varroosis – the ongoing crisis in beekeeping. J. Verbr. Lebensm. 3, 221–228. doi: 10.1007/s00003-008-0331-y
Boecking O., Spivak M. (1999). Behavioral defenses of honey bees against Varroa jacobsoni Oud. Apidologie 30, 141–158.
Boot W. J., Schoenmaker J., Calis J. N. M., Beetsma J. (1995). Invasion of Varroa jacobsoni into drone brood cells of the honey bee, Apis mellifera. Apidologie 26, 109–118. doi: 10.1051/apido:19950204
Bowen-Walker P. L., Gunn A. (2001). The effect of the ectoparasitic mite, Varroa destructor on adult worker honeybee (Apis mellifera) emergence weights, water, protein, carbohydrate, and lipid levels. Entomol. Exp. Appl. 3, 207–217. doi: 10.1023/A:1019254727879
Bruckner S., Straub L., Neumann P., Williams G. R. (2021). Synergistic and antagonistic interactions between Varroa destructor mites and neonicotinoid insecticides in male Apis mellifera honey bees. Front. Eco. Evol. 9. doi: 10.3389/fevo.2021.756027
Bruckner S., Straub L., Neumann P., Williams G. R. (2023b). Negative but antagonistic effects of neonicotinoid insecticides and ectoparasitic mites Varroa destructor on Apis mellifera honey bee food glands. Chemosphere 313, 137535. doi: 10.1016/j.chemosphere.2022.137535
Bruckner S., Wilson M., Aurell D., Rennich K., vanEngelsdorp D., Steinhauer N., et al. (2023a). A national survey of managed honey bee colony losses in the USA: results from the Bee Informed Partnership for 2017–18, 2018–19, and 2019–20. J. Apic. Res. 62, 429–443. doi: 10.1080/00218839.2022.2158586
Burritt N. L., Foss N. J., Neeno-Eckwall E. C., Church J. O., Hilger A. M., Hildebrand J. A., et al. (2016). Sepsis and hemocyte loss in honey bees (Apis mellifera) infected with Serratia marcescens strain Sicaria. PloS One 11, e0167752. doi: 10.1371/journal.pone.0167752
Caron D. M., Connor L. J. (2013). Honey Bee Biology and Beekeeping, Revised Edition (Kalamazoo: Wicwas Press).
Chen G., Wang S., Jia S., Feng Y., Hu F., Chen Y., et al. (2021). A new atrain of virus discovered in China specific to the parasitic mite Varroa destructor poses a potential threat to honey bees. Viruses 13, 679. doi: 10.3390/v13040679
Cornman S. R., Schatz M. C., Johnston S. J., Chen Y.-P., Pettis J., Hunt G., et al. (2010). Genomic survey of the ectoparasitic mite Varroa destructor, a major pest of the honey bee Apis mellifera. BMC Genomics 11, 602. doi: 10.1186/1471-2164-11-602
Dainat B., Evans J. D., Chen Y. P., Gauthier L., Neumann P. (2012). Dead or alive: Deformed wing virus and Varroa destructor reduce the life span of winter honeybees. Appl. Environ. Microbiol. 78, 981–987. doi: 10.1128/AEM.06537-11
De Jong D., De Jong P. H. (1983). Longevity of Africanized honey bees (Hymenoptera: Apidae) infested by Varroa jacobsoni (Parasitiformes: Varroidae). J. Econ. Entomol. 76, 766–768. doi: 10.1093/jee/76.4.766
De Jong D., Morse R. A., Eickwort G. C. (1982). Mite pests of honey bees. Ann. Rev. Entomol. 27, 229–252. doi: 10.1146/annurev.en.27.010182.001305
De la Mora A., Morfin N., Espinosa-Montaño L. G., Medina-Flores C. A., Guzman-Novoa E. (2021). The mite Varroa destructor lowers the stinging response threshold of honey bees (Apis mellifera). J. Apic. Res. 0, 1–5. doi: 10.1080/00218839.2021.1959754
Delaplane K. S., Ellis J. D., Hood W. M. (2010). A test for interactions between Varroa destructor (Acari: Varroidae) and Aethina tumida (Coleoptera: Nitidulidae) in colonies of Honey bees (Hymenoptera: Apidae). Ann. Entomol. Soc Am. 103, 711–715. doi: 10.1603/AN09169
De Miranda J. R., Cornman R. S., Evans J. D., Semberg E., Haddad N., Neumann P., et al. (2015). Genome characterization, prevalence and distribution of a Macula-Like Virus from Apis mellifera and Varroa destructor. Viruses 7, 3586–3602. doi: 10.3390/v7072789
Di Prisco G., Annoscia D., Margiotta M., Ferrara R., Varricchio P., Zanni V., et al. (2016). A mutualistic symbiosis between a parasitic mite and a pathogenic virus undermines honey bee immunity and health. PNAS 113, 3203–3208. doi: 10.1073/pnas.1523515113
Dmitryjuk M., Żółtowska K., Frączek R., Lipiński Z. (2014). Esterases of Varroa destructor (Acari: Varroidae), parasitic mite of the honeybee. Exp. Appl. Acarol. 62, 499–510. doi: 10.1007/s10493-013-9754-y
Donzé G., Guerin P. M. (1994). Behavioral attributes and parental care of varroa mites parasitizing honeybee brood. Behav. Ecol. Sociobio. 34, 305–319. doi: 10.1007/BF00197001
Donzé G., Herrmann M., Bachofen B., Guerin P. R. M. (1996). Effect of mating frequency and brood cell infestation rate on the reproductive success of the honeybee parasite. Varroa jacobsoni. Ecol. Entomol. 21, 17–26. doi: 10.1111/j.1365-2311.1996.tb00261.x
Doublet V., Poeschl Y., Gogol-Döring A., Alaux C., Annoscia D., Aurori C., et al. (2017). Unity in defence: honeybee workers exhibit conserved molecular responses to diverse pathogens. BMC Genomics 18, 207. doi: 10.1186/s12864-017-3597-6
Duan X.-L., Zhao B.-A., Liu Y., Xiong M.-Q., He N., Huang S.-K., et al. (2020). Development and characterization of six novel microsatellite markers for honey bee parasitic mite Varroa destructor (Mesostigmata: Varroidae). SAA 25, 1733–1744. doi: 10.11158/saa.25.10.2
Duay P., De Jong D., Engels W. (2002). Decreased flight performance and sperm production in drones of the honey bee (Apis mellifera) slightly infested by Varroa destructor mites during pupal development. Genet. Mol. Res. 1 (3), 227–232.
Dynes T. L., De Roode J. C., Lyons J. I., Berry J. A., Delaplane K. S., Brosi B. J. (2016). Fine scale population genetic structure of Varroa destructor, an ectoparasitic mite of the honey bee (Apis mellifera). Apidologie 2016, 1–9. doi: 10.1007/s13592-016-0453-7
Erban T., Harant K., Hubalek M., Vitamvas P., Kamler M., Poltronieri P., et al. (2015). In-depth proteomic analysis of Varroa destructor: Detection of DWV-complex, ABPV, VdMLV and honeybee proteins in the mite. Sci. Rep. 5, 13907. doi: 10.1038/srep13907
Erban T., Sopko B., Kadlikova K., Talacko P., Harant K. (2019). Varroa destructor parasitism has a greater effect on proteome changes than the deformed wing virus and activates TGF-β signaling pathways. Sci. Rep. 9, 9400. doi: 10.1038/s41598-019-45764-1
Francis R. M., Nielsen S. L., Kryger P. (2013). Varroa-virus interaction in collapsing honey bee colonies. PloS One 8, e57540. doi: 10.1371/journal.pone.0057540
Gajić B., Munoz I., de la Rua P., Stevanović J., Lakić N., Kulišić Z., et al. (2019). Coexistence of genetically different Varroa destructor in Apis mellifera colonies. Exp. Appl. Acarol. 78, 315–326. doi: 10.1007/s10493-019-00395-z
Galbraith D. A., Yang X., Nino E. L., Yi S., Grozinger C. (2015). Parallel epigenomic and transcriptomic responses to viral infection in honey bees (Apis mellifera). PloS Pathog. 11 (3), e1004713. doi: 10.1371/journal.ppat.1004713
Gallai N., Salles J.-M., Settele J., Vaissière B. E. (2009). Economic valuation of the vulnerability of world agriculture confronted with pollinator decline. Ecol. Econ. 68, 810–821. doi: 10.1016/j.ecolecon.2008.06.014
Genersch E. (2010). Honey bee pathology: current threats to honey bees and beekeeping. Appl. Microbiol. Biotechnol. 87, 87–97. doi: 10.1007/s00253-010-2573-8
Gisder S., Genersch E. (2021). Direct evidence for infection of Varroa destructor mites with the bee-pathogenic deformed wing virus variant B, but not variant Avia fluorescence in situ hybridization analysis. J. Virol. 95, e01786–e01720. doi: 10.1128/JVI.01786-20
Glavinić U., Stevanović J., Gajić B., Simeunović P., Đurić S., Vejnović B., et al. (2014). Nosema ceranae DNA in honey bee haemolymph and honey bee mite Varroa destructor. Acta Veterinaria-Beograd 64, 349–357. doi: 10.2478/acve-2014-0033
Glinski Z., Jarosz J. (1984). Alterations in haemolymph proteins of drone honey bee larvae parasitized by Varroa jacobsoni. Apidologie 15, 329–338. doi: 10.1051/apido:19840305
Gray A., Adjlane N., Arab A., Ballis A., Brusbardis V., Douglas B., et al. (2023). Honey bee colony loss rates in 37 countries using the COLOSS survey for winter 2019–2020: the combined effects of operation size, migration and queen replacement. J. Apicultural Res. 62, 204–210. doi: 10.1080/00218839.2022.2113329
Gregorc A., Evans J. D., Scharf M., Ellis J. D. (2012). Gene expression in honey bee (Apis mellifera) larvae exposed to pesticides and Varroa mites (Varroa destructor). J. Insect. Physiol. 58, 1042–1049. doi: 10.1016/j.jinsphys.2012.03.015
Gregory P. G., Evans J. D., Rinderer T., de Guzman L. (2005). Conditional immune-gene suppression of honeybees parasitized by Varroa mites. J. Insect. Sci. 5, 7.
Grindrod I., Martin S. J. (2023). Varroa resistance in Apis cerana: a review. Apidologie 54, 14. doi: 10.1007/s13592-022-00977-8
Guzman-Novoa E., Eccles L., Calvete Y., Mcgowan J., Kelly P. G., Correa-Benítez A. (2010). Varroa destructor is the main culprit for the death and reduced populations of overwintered honey bee (Apis mellifera) colonies in Ontario, Canada. Apidologie 41, 443–450. doi: 10.1051/apido/2009076
Guzman-Novoa E., Emsen B., Unger P., Espinosa-Montaño L. G., Petukhova T. (2012). Genotypic variability and relationships between mite infestation levels, mite damage, grooming intensity, and removal of Varroa destructor mites in selected strains of worker honey bees (Apis mellifera L.). J. Invertebr. Pathol. 110, 314–320. doi: 10.1016/j.jip.2012.03.020
Guzman-Novoa E., Morfin N. (2019). “Disease resistance in honey bees (Apis mellifera L.) at the colony and individual levels,” in Comprehensive Biotechnology, 3rd ed. Ed. Moo-Young M. (Oxford: Elsevier Pergamon), 811–817.
Hamiduzzaman M. M., Emsen B., Hunt G. J., Subramanyam S., Williams C. E., Tsuruda J. M., et al. (2017). Differential gene expression associated with honey bee grooming behavior in response to Varroa mites. Behav. Genet. 47, 335–344. doi: 10.1007/s10519-017-9834-6
Harbo J. R., Harris J. W. (2009). Responses to Varroa by honey bees with different levels of Varroa Sensitive Hygiene. J. Apic. Res. 48, 156–161. doi: 10.3896/IBRA.1.48.3.02
Hasegawa N., Techer M., Mikheyev A. S. (2021). A toolkit for studying Varroa genomics and transcriptomics: preservation, extraction, and sequencing library preparation. BMC Genomics 22, 54. doi: 10.1186/s12864-020-07363-7
Hawkins G. P., Martin S. J. (2021). Elevated recapping behaviour and reduced Varroa destructor reproduction in natural Varroa resistant Apis mellifera honey bees from the UK. Apidologie 52, 647–657. doi: 10.1007/s13592-021-00852-y
Herrero S., Millán-Leiva A., Coll S., González-Martínez R. M., Parenti S., González-Cabrera J. (2019). Identification of new viral variants specific to the honey bee mite Varroa destructor. Exp. Appl. Acarol. 79, 157–168. doi: 10.1007/s10493-019-00425-w
Hristov P., Shumkova R., Palova N., Neov B. (2020). Factors associated with honey bee colony losses: A mini-review. Vet. Sci. 7, 166. doi: 10.3390/vetsci7040166
Hubert J., Bicianova M., Ledvinka O., Kamler M., Lester P. J., Nesvorna M., et al. (2017). Changes in the bacteriome of honey bees associated with the parasite Varroa destructor, and pathogens Nosema and Lotmaria passim. Microb. Ecol. 73, 685–698. doi: 10.1007/s00248-016-0869-7
Ifantidis M. (1983). Ontogenesis of the mite Varroa jacobsoni in worker and drone honeybee brood cells. J. Apic. Res. 22, 200–206. doi: 10.1080/00218839.1983.11100588
Invernizzi C., Zefferino I., Santos E., Sánchez L., Mendoza Y. (2015). Multilevel assessment of grooming behavior against Varroa destructor in Italian and Africanized honey bees. J. Apic. Res. 54, 321–327. doi: 10.1080/00218839.2016.1159055
Iqbal J., Mueller U. (2007). Virus infection causes specific learning deficits in honeybee foragers. Proc. Biol. Sci. 274, 1517–1521. doi: 10.1098/rspb.2007.0022
Kanbar G., Engels W. (2003). Ultrastructure and bacterial infection of wounds in honey bee (Apis mellifera) pupae punctured by Varroa mites. Parasitol. Res. 90, 349–354. doi: 10.1007/s00436-003-0827-4
Kanost M. R. (2009). “Hemolymph,” in Encyclopedia of Insects. Eds. Resh V. H., Cardé R. T. (Amsterdam: Academic Press), 446–449.
Khalifa S. A. M., Elshafiey E. H., Shetaia A. A., El-Wahed A. A. A., Algethami A. F., Musharraf S. G., et al. (2021). Overview of bee pollination and its economic value for crop production. Insects 12, 688. doi: 10.3390/insects12080688
Khongphinitbunjong K., de Guzman L. I., Tarver M. R., Rinderer T. E., Chen Y., Chantawannakul P. (2015). Differential viral levels and immune gene expression in three stocks of Apis mellifera induced by different numbers of Varroa destructor. J. Insect. Physiol. 72, 28–34. doi: 10.1016/j.jinsphys.2014.11.005
Kirrane M. J., de Guzman L. I., Rinderer T. E., Frake A. M., Wagnitz J., Whelan P. M. (2012). Age and reproductive status of adult Varroa mites affect grooming success of honey bees. Exp. Appl. Acarol. 58, 423–430. doi: 10.1007/s10493-012-9591-4
Koç N., İnak E., Jonckheere W., Van Leeuwen T. (2021). Genetic analysis and screening of pyrethroid resistance mutations in Varroa destructor populations from Turkey. Exp. Appl. Acarol. 84 (2), 433–444. doi: 10.1007/s10493-021-00626-2
Koleoglu G., Goodwin P. H., Reyes-Quintana M., Hamiduzzaman M. M., Guzman-Novoa E. (2017). Effect of Varroa destructor, wounding and varroa homogenate on gene expression in brood and adult honey bees. PloS One 12, e0169669. doi: 10.1371/journal.pone.0169669
Koleoglu G., Goodwin P. H., Reyes-Quintana M., Hamiduzzaman M. M., Guzman-Novoa E. (2018). Varroa destructor parasitism reduces hemocyte concentrations and prophenol oxidase gene expression in bees from two populations. Parasitol. Res. 117, 1175–1183. doi: 10.1007/s00436-018-5796-8
Kralj J., Brockmann A., Fuchs S., Tautz J. (2007). The parasitic mite Varroa destructor affects non-associative learning in honey bee foragers, Apis mellifera L. J. Comp. Physiol. A. Neuroethol. Sens. Neural. Behav. Physiol. 193, 363–370. doi: 10.1007/s00359-006-0192-8
Kralj J., Fuchs S. (2006). Parasitic Varroa destructor mites influence flight duration and homing ability of infested Apis mellifera foragers. Apidologie 37, 577–587. doi: 10.1051/apido:2006040
Kunc M., Dobeš P., Ward R., Lee S., Čegan R., Dostálková S., et al. (2023). Omics-based analysis of honey bee (Apis mellifera) response to Varroa sp. parasitisation and associated factors reveals changes impairing winter bee generation. Insect Biochem. Mol. Biol. 152, 103877. doi: 10.1016/j.ibmb.2022.103877
Kuster R. D., Boncristiani H. F., Rueppell O. (2014). Immunogene and viral transcript dynamics during parasitic Varroa destructor mite infection of developing honey bee (Apis mellifera) pupae. J. Exp. Biol. 217, 1710–1718. doi: 10.1242/jeb.097766
Lapidge K. L., Oldroyd B. P., Spivak M. (2002). Seven suggestive quantitative trait loci influence hygienic behavior of honey bees. Naturwissenschaften 89, 565–568. doi: 10.1007/s00114-002-0371-6
Lercher A., Baazim H., Bergthaler A. (2020). Systemic immunometabolism: Challenges and opportunities. Immunity 53, 496–509. doi: 10.1016/j.immuni.2020.08.012
Levin S., Sela N., Chejanovsky N. (2016). Two novel viruses associated with the Apis mellifera pathogenic mite Varroa destructor. Sci. Rep. 6, 37710. doi: 10.1038/srep37710
Li Z., Chen Y., Zhang S., Chen S., Li W., Yan L., et al. (2013). Viral infection affects sucrose responsiveness and homing ability of forager honey bees, Apis mellifera L. PloS One 8, e77354. doi: 10.1371/journal.pone.0077354
Li A. Y., Cook S. C., Sonenshine D. E., Posada-Florez F., Noble N. I. I., Mowery J., et al. (2019). Insights into the feeding behaviors and biomechanics of Varroa destructor mites on honey bee pupae using electropenetrography and histology. J. Insect Physiol. 119, 103950. doi: 10.1016/j.jinsphys.2019.103950
Lin Z., Wang S., Neumann P., Chen G., Page P., Li L., et al. (2021). Population genetics and host specificity of Varroa destructor mites infesting eastern and western honeybees. J. Pest Sci. 94, 1487–1504. doi: 10.1007/s10340-020-01322-7
Ling E., Yu X.-Q. (2006). Cellular encapsulation and melanization are enhanced by immulectins, pattern recognition receptors from the tobacco hornworm Manduca sexta. Dev. Comp. Immunol. 30, 289–299. doi: 10.1016/j.dci.2005.05.005
Little C. M., Shutler D., Williams G. R. (2015). Associations among Nosema spp. fungi, Varroa destructor mites, and chemical treatments in honey bees, Apis mellifera. J. Apic. Res. 54, 378–385. doi: 10.1080/00218839.2016.1159068
Lucas T., DeGrandi-Hoffman G., Gronenberg W. (2006). Brain morphology and learning in the honey bee, Apis mellifera, parasitized during development by ectoparasitic Varroa mite, Varroa destructor. Entomological Society of America Annual Meeting, December 10-13, 2006.
Marche M. G., Satta A., Floris I., Pusceddu M., Buffa F., Ruiu L. (2019). Quantitative variation in the core bacterial community associated with honey bees from Varroa-infested colonies. J. Apic. Res. 58, 444–454. doi: 10.1080/00218839.2019.1589669
Mariani F., Maggi M., Porrini M., Fuselli S., Caraballo G., Brasesco C., et al. (2012). Parasitic interactions between Nosema spp. and Varroa destructor in Apis mellifera colonies. Zootec. Trop. 30, 081–090.
Marmaras V. J., Lampropoulou M. (2009). Regulators and signalling in insect haemocyte immunity. Cell Signal 21, 186–195. doi: 10.1016/j.cellsig.2008.08.014
Martin S. J., Brettell L. E. (2019). Deformed wing virus in honeybees and other insects. Annu. Rev. Virol. 6, 49–69. doi: 10.1146/annurev-virology-092818-015700
McAfee A., Chapman A., Iovinella I., Gallagher-Kurtzke Y., Collins T. F., Higo H., et al. (2018). A death pheromone, oleic acid, triggers hygienic behavior in honey bees (Apis mellifera L.). Sci. Rep. 8, 5719. doi: 10.1038/s41598-018-24054-2
McMenamin A. J., Daughenbaugh K. F., Parekh F., Pizzorno M. C., Flenniken M. L. (2018). Honey bee and bumble bee antiviral defense. Viruses 10, 395. doi: 10.3390/v10080395
Medina-Flores C. A., Macias-Macias J. O., Cárdenas A. R., Rivera A. S., Vasquez H. C., Carrillo-Muro O., et al. (2021). Pérdida de colonias de abejas melíferas y factores asociados en el centro-occidente de México en los inviernos del 2016 al 2019. Rev. Bio Cienc. 8, 11. doi: 10.15741/revbio.08.e1095
Monchanin C., Henry M., Decourtye A., Dalmon A., Fortini D., Bœuf E., et al. (2019). Hazard of a neonicotinoid insecticide on the homing flight of the honeybee depends on climatic conditions and Varroa infestation. Chemosphere 224, 360–368. doi: 10.1016/j.chemosphere.2019.02.129
Mondet F., Alaux C., Severac D., Rohmer M., Mercer A. R., Le Conte Y. (2015). Antennae hold a key to Varroa-sensitive hygiene behaviour in honey bees. Sci. Rep. 5, 10454. doi: 10.1038/srep10454
Morawetz L., Köglberger H., Griesbacher A., Derakhshifar I., Crailsheim K., Brodschneider R., et al. (2019). Health status of honey bee colonies (Apis mellifera) and disease-related risk factors for colony losses in Austria. PloS One 14, e0219293. doi: 10.1371/journal.pone.0219293
Mordecai G. J., Brettell L. E., Pachori P., Villalobos E. M., Martin S. J., Jones I. M., et al. (2016). Moku virus; a new Iflavirus found in wasps, honey bees and Varroa. Sci. Rep. 6, 34983. doi: 10.1038/srep34983
Morfin N., Anguiano-Baez R., Guzman-Novoa E. (2021). Honey bee (Apis mellifera) immunity. Vet. Clin. North Am. Food Anim. Pract. 37, 521–533. doi: 10.1016/j.cvfa.2021.06.007
Morfin N., Given K., Evans M., Guzman-Novoa E., Hunt G. J. (2020d). Grooming behavior and gene expression of the Indiana “mite-biter” honey bee stock. Apidologie 51, 267–275. doi: 10.1007/s13592-019-00710-y
Morfin N., Goodwin P. H., Guzman-Novoa E. (2020a). Interaction of Varroa destructor and sublethal clothianidin doses during the larval stage on subsequent adult honey bee (Apis mellifera L.) health, cellular immunity, Deformed wing virus levels and differential gene expression. Microorganisms 8, 858. doi: 10.3390/microorganisms8060858
Morfin N., Goodwin P. H., Guzman-Novoa E. (2020b). Interaction of field realistic doses of clothianidin and Varroa destructor parasitism on adult honey bee (Apis mellifera L.) health and neural gene expression, and antagonistic effects on differentially expressed genes. PloS One 15, e0229030. doi: 10.1371/journal.pone.0229030
Morfin N., Goodwin P. H., Guzman-Novoa E. (2020c). The Combined Effects of Varroa destructor parasitism and exposure to neonicotinoids affects honey bee (Apis mellifera L.) memory and gene expression. Biology 9, 237. doi: 10.3390/biology9090237
Morfin N., Goodwin P. H., Hunt G. J., Guzman-Novoa E. (2019). Effects of sublethal doses of clothianidin and/or V. destructor on honey bee (Apis mellifera) self-grooming behavior and associated gene expression. Sci. Rep. 9, 5196. doi: 10.1038/s41598-019-41365-0
Morfin N., Harpur B. A., de la Mora A., Guzman-Novoa E. (2023). Breeding honey bees (Apis mellifera L.) for low and high Varroa destructor population growth: Gene expression of bees performing grooming behavior. Front. Insect. Sci. 3. doi: 10.3389/finsc.2023.951447
Morfin N., Kozak P., Ledger L., You Q., Bell-Rogers P., Zechel J., et al. (2022). Surveillance of viruses in samples collected from honey bee colonies in Ontario, Canada, between 2015 and 2019. J. Apic. Sci. 66, 209–215. doi: 10.2478/jas-2022-0009
Muntaabski I., Russo R. M., Liendo M. C., Palacio M. A., Cladera J. L., Lanzavecchia S. B., et al. (2020). Genetic variation and heteroplasmy of Varroa destructor inferred from ND4 mtDNA sequences. Parasitol. Res. 119, 411–421. doi: 10.1007/s00436-019-06591-5
Navajas M., Anderson D. L., Guzman L. I., Huang Z. Y., Clement J., Zhou T., et al. (2010). New Asian types of Varroa destructor: a potential new threat for world apiculture. Apidologie 41, 181–193. doi: 10.1051/apido/2009068
Navajas M., Migeon A., Alaux C., Martin-Magniette M. L., Robinson G. E., Evans J. D., et al. (2008). Differential gene expression of the honey bee Apis mellifera associated with Varroa destructor infection. BMC Genomics 9, 1–11. doi: 10.1186/1471-2164-9-301
Nazzi F., Vedova G. D., D’Agaro M. (2004). A semiochemical from brood cells infested by Varroa destructor triggers hygienic behaviour in Apis mellifera. Apidologie 35, 65–70. doi: 10.1051/apido:2003065
Neumann P., Carreck N. L. (2010). Honey bee colony losses. J. Apicultural Res. 49, 1–6. doi: 10.3896/IBRA.1.49.1.01
Noël A., Le Conte Y., Mondet F. (2020). Varroa destructor: how does it harm Apis mellifera honey bees and what can be done about it? Emerg. Top. Life Sci. 4, 45–57. doi: 10.1042/ETLS20190125
Ogihara M. H., Yoshiyama M., Morimoto N., Kimura K. (2020). Dominant honeybee colony infestation by Varroa destructor (Acari: Varroidae) K haplotype in Japan. Appl. Entomol. Zool. 55, 189–197. doi: 10.1007/s13355-020-00667-w
Oldroyd B. P. (1999). Coevolution while you wait: Varroa jacobsoni, a new parasite of western honeybees. Trends Ecol. Evol. 14, 312–315. doi: 10.1016/S0169-5347(99)01613-4
Oldroyd B. P. (2007). What’s killing American honey bees? PloS Biol. 5, e168. doi: 10.1371/journal.pbio.0050168
Ostermann D. J. (2003). Interactions of varroa, Varroa destructor Anderson and Trueman, with chalkbrood, Ascosphaera apis (Maassen ex Claussen) Olive & Spiltoir, and nosema, Nosema apis Zander, in honey bee, Apis mellifera L., colonies treated with formic acid, and the influence of hive and ambient conditions on formic acid concentration in the hive (Winnipeg (MB: University of Manitoba).
Papa G., Maier R., Durazzo A., Lucarini M., Karabagias I. K., Plutino M., et al. (2022). The Honey bee Apis mellifera: An insect at the interface between human and ecosystem health. Biology 11, 233. doi: 10.3390/biology11020233
Pinto F., Souza G., Sanches M., Serrão J. (2011). Parasitic Effects of Varroa destructor (Acari: Varroidae) on hypopharyngeal glands of Africanized Apis mellifera (Hymenoptera: Apidae). Sociobiology 58, 769–778.
Power K., Martano M., Altamura G., Piscopo N., Maiolino P. (2021). Histopathological features of symptomatic and asymptomatic honeybees naturally infected by Deformed wing virus. Pathogens 10, 874. doi: 10.3390/pathogens10070874
Ramsey S., Gulbronson C. J., Mowery J., Ochoa R., vanEngelsdorp D., Bauchan G. (2018). A Multi-microscopy approach to discover the feeding site and host tissue consumed by Varroa destructor on host honey bees. Microsc. Microanal. 24, 1258–1259. doi: 10.1017/S1431927618006773
Ramsey S. D., Ochoa R., Bauchan G., Gulbronson C., Mowery J. D., Cohen A., et al. (2019). Varroa destructor feeds primarily on honey bee fat body tissue and not hemolymph. PNAS 116, 1792–1801. doi: 10.1073/pnas.1818371116
Rasolofoarivao H., Clémencet J., Speck A., Raveloson-Ravaomanarivo L. H., Reynaud Delatte B. H. (2017). Genetic diversity of Varroa destructor parasitizing Apis mellifera unicolor in Madagascar. Apidologie 48, 648–656. doi: 10.1007/s13592-017-0509-3
Rath W. (1999). Co-adaptation of Apis cerana Fabr. and Varroa jacobsoni Oud. Apidologie 30, 97–110. doi: 10.1051/apido:19990202
Raymann K., Moran N. A. (2018). The role of the gut microbiome in health and disease of adult honey bee workers. Curr. Opin. Insect. Sci. 26, 97–104. doi: 10.1016/j.cois.2018.02.012
Regoes R. R., Hamblin S., Tanaka M. M. (2013). Viral mutation rates: modelling the roles of within-host viral dynamics and the trade-off between replication fidelity and speed. Proc. R. Sco. B: Bio. Sci. 280, 20122047. doi: 10.1098/rspb.2012.2047
Rehm S.-M., Ritter W. (1989). Sequence of the sexes in the offspring of Varroa jacobsoni and the resulting consequences for the calculation of the developmental period. Apidologie 20, 339–343. doi: 10.1051/apido:19890406
Reyes-Quintana M., Espinosa-Montaño L. G., Prieto-Merlos D., Koleoglu G., Petukhova T., Correa-Benítez A., et al. (2019). Impact of Varroa destructor and deformed wing virus on emergence, cellular immunity, wing integrity and survivorship of Africanized honey bees in Mexico. J. Invertebr. Pathol. 164, 43–48. doi: 10.1016/j.jip.2019.04.009
Richards E. H., Jones B., Bowman A. (2011). Salivary secretions from the honeybee mite, Varroa destructor: effects on insect haemocytes and preliminary biochemical characterization. Parasitology 138, 602–608. doi: 10.1017/S0031182011000072
Rinderer T. E., Guzman L. I., Delatte G. T., Stelzer J. A., Lancaster V. A., Kuznetsov V., et al. (2001). Resistance to the parasitic mite Varroa destructor in honey bees from far-eastern Russia. Apidologie 32, 381–394. doi: 10.1051/apido:2001138
Rolff J., Reynolds S. (2009). Insect Infection and Immunity: Evolution, Ecology, and Mechanisms (Oxford: Oxford University Press).
Salem M. H., Abir A. G., Ramadan H. (2006). Effect of Varroa destructor on different haemocyte count, total haemolymph protein on larvae, pupae and adults of Apis mellifera drones. J. Egypt. Soc 35, 93–96.
Salmela H., Sundström L. (2018). Vitellogenin in inflammation and immunity in social insects. Inflamm. Cell Sig 5, e1506. doi: 10.14800/ics.1506
Santillan-Galicia M. T., Carzaniga R., Ball B. V., Alderson P. G. (2008). Immunolocalization of deformed wing virus particles within the mite Varroa destructor. J. Gen. Virol. 89, 1685–1689. doi: 10.1099/vir.0.83223-0
Schwartz K. R., Minor H., Magro C., McConnell J., Capani J., Griffin J., et al. (2021). The neonicotinoid imidacloprid alone alters the cognitive behavior in Apis mellifera L. and the combined exposure of imidacloprid and Varroa destructor mites synergistically contributes to trial attrition. J. Apic. Res. 60, 431–438. doi: 10.1080/00218839.2020.1866233
Shah K. S., Evans E. C., Pizzorno M. C. (2009). Localization of deformed wing virus (DWV) in the brains of the honeybee, Apis mellifera Linnaeus. Virol. J. 6, 182. doi: 10.1186/1743-422X-6-182
Shen M., Yang X., Cox-Foster D., Cui L. (2005). The role of varroa mites in infections of Kashmir bee virus (KBV) and deformed wing virus (DWV) in honey bees. Virology 342, 141–149. doi: 10.1016/j.virol.2005.07.012
Solignac M., Cornuet J.-M., Vautrin D., Le Conte Y., Anderson D., Evans J., et al. (2005). The invasive Korea and Japan types of Varroa destructor, ectoparasitic mites of the Western honeybee (Apis mellifera), are two partly isolated clones. Proc. Biol. Sci. 272, 411–419. doi: 10.1098/rspb.2004.2853
Spivak M., Reuter G. S. (1998). Performance of hygienic honey bee colonies in a commercial apiary. Apidologie 29, 291–302. doi: 10.1051/apido:19980308
Strand M. R. (2008). The insect cellular immune response. Insect Sci. 15 (1), 1–14. doi: 10.1111/j.1744-7917.2008.00183.x
Strapazzon R., Carneiro F. E., Guerra J. C. V., Moretto G. (2009). Genetic characterization of the mite Varroa destructor (Acari: Varroidae) collected from honey bees Apis mellifera (Hymenoptera, Apidae) in the state of Santa Catarina, Brazil. Genet. Mol. Res. 8, 990–997. doi: 10.4238/vol8-3gmr567
Straub L., Williams G. R., Vidondo B., Khongphinitbunjong K., Retschnig G., Schneeberger A., et al. (2019). Neonicotinoids and ectoparasitic mites synergistically impact honeybees. Sci. Rep. 9, 8159. doi: 10.1038/s41598-019-44207-1
Strauss U., Dietemann V., Human H., Crewe R. M., Pirk C. W. W. (2016). Resistance rather than tolerance explains survival of savannah honeybees (Apis mellifera scutellata) to infestation by the parasitic mite Varroa destructor. Parasitology 143, 374–387. doi: 10.1017/S0031182015001754
Tesovnik T., Cizelj I., Zorc M., Čitar M., Čitar M., Glavan G., et al. (2017). Immune related gene expression in worker honey bee (Apis mellifera carnica) pupae exposed to neonicotinoid thiamethoxam and Varroa mites (Varroa destructor). PloS One 12, e0187079. doi: 10.1371/journal.pone.0187079
Tesovnik T., Zorc M., Gregorc A., Rinehart T., Adamczyk J., Narat M. (2019). Immune gene expression in developing honey bees (Apis mellifera L.) simultaneously exposed to imidacloprid and Varroa destructor in laboratory conditions. J. Apic. Res. 58, 730–739. doi: 10.1080/00218839.2019.1634463
Traynor K. S., Mondet F., de Miranda J. R., Techer M., Kowallik V., Oddie M. A. Y., et al. (2020). Varroa destructor: A complex parasite, crippling honey bees worldwide. Trends Parasitl. 36, 592–606. doi: 10.1016/j.pt.2020.04.004
Unger P., Guzman-Novoa E. (2010). Maternal effects on the hygienic behavior of Russian × Ontario hybrid honeybees (Apis mellifera L.). J. Hered. 101, 91–96. doi: 10.1093/jhered/esp092
van Dooremalen C., Cornelissen B., Poleij-Hok-Ahin C., Blacquière T. (2018). Single and interactive effects of Varroa destructor, Nosema spp., and imidacloprid on honey bee colonies (Apis mellifera). Ecosphere 9, e02378. doi: 10.1002/ecs2.2378
van Dooremalen C., Stam E., Gerritsen L., Cornelissen B., van der Steen J., van Langevelde F., et al. (2013). Interactive effect of reduced pollen availability and Varroa destructor infestation limits growth and protein content of young honey bees. J. Insect. Physiol. 59, 487–493. doi: 10.1016/j.jinsphys.2013.02.006
van Dooremalen C., van Langevelde F. (2021). Can colony size of honeybees (Apis mellifera) be used as predictor for colony losses due to Varroa destructor during Winter? Agriculture 11, 529. doi: 10.3390/agriculture11060529
vanEngelsdorp D., Evans J. D., Saegerman C., Mullin C., Haubruge E., Nguyen B. K., et al. (2009). Colony Collapse Disorder: A descriptive study. PloS One 4, e6481. doi: 10.1371/journal.pone.0006481
vanEngelsdorp D., Speybroeck N., Evans J. D., Nguyen B. K., Mullin C., Frazier M., et al. (2010). Weighing risk factors associated with bee colony collapse disorder by classification and regression tree analysis. J. Econ. Entomol. 103, 1517–1523. doi: 10.1603/ec09429
Verma L. R., Partap U. (1993). The Asian hive bee, Apis cerana, as a pollinator in vegetable seed production (International Centre for Integrated Mountain Development (ICIMOD).
Wagoner K., Spivak M., Hefetz A., Reams T., Rueppell O. (2019). Stock-specific chemical brood signals are induced by Varroa and Deformed wing virus, and elicit hygienic response in the honey bee. Sci. Rep. 9, 8753. doi: 10.1038/s41598-019-45008-2
Wu J.-L., Zhou C.-X., Wu P.-J., Xu J., Guo Y.-Q., Xue F., et al. (2017). Brain metabolomic profiling of eastern honey bee (Apis cerana) infested with the mite Varroa destructor. PloS One 12, e0175573. doi: 10.1371/journal.pone.0175573
Yang X., Cox-Foster D. L. (2005). Impact of an ectoparasite on the immunity and pathology of an invertebrate: Evidence for host immunosuppression and viral amplification. PNAS 102, 7470–7475. doi: 10.1073/pnas.0501860102
Yang X., Cox-Foster D. (2007). Effects of parasitization by Varroa destructor on survivorship and physiological traits of Apis mellifera in correlation with viral incidence and microbial challenge. Parasitology 134, 405–412. doi: 10.1017/S0031182006000710
Yang H., Shi J., Liao C., Yan W., Wu X. (2021). Varroa destructor mite infestations in capped brood cells of honeybee workers affect emergence development and adult foraging ability. Curr. Zool. 67, 569–571. doi: 10.1093/cz/zoab002
Yue C., Genersch E. (2005). RT-PCR analysis of Deformed wing virus in honeybees (Apis mellifera) and mites (Varroa destructor). J. Gen. Virol. 86, 3419–3424. doi: 10.1099/vir.0.81401-0
Zakaria M. E., Abd El-Wahab T. E. (2004). Scanning electron microscopic (SEM) of hypopharyngeal and mandibular glands of honey bee workers infested by Varroa mites (Varroa destructor). EJAS 55 (3), 375–384.
Zanni V., Galbraith D. A., Annoscia D., Grozinger C. M., Nazzi F. (2017). Transcriptional signatures of parasitization and markers of colony decline in Varroa-infested honey bees (Apis mellifera). Insect. Biochem. Mol. Biol. 87, 1–13. doi: 10.1016/j.ibmb.2017.06.002
Zaobidna E. A., Żółtowska K., Łopieńska-Biernat E. (2015). Expression of the Prophenoloxidase gene and phenoloxidase activity, during the development of Apis mellifera brood infected with Varroa destructor. J. Apic. Sci. 59, 85–93. doi: 10.1515/jas-2015-0025
Zaobidna E. A., Żółtowska K., Łopieńska-Biernat E. (2017). Varroa destructor induces changes in the expression of immunity-related genes during the development of Apis mellifera worker and drone broods. Acta Parasitol. 62, 779–789. doi: 10.1515/ap-2017-0094
Zhang Y., Han R. (2018). A saliva protein of Varroa mites contributes to the toxicity toward Apis cerana and the DWV elevation in A. mellifera. Sci. Rep. 8, 3387. doi: 10.1038/s41598-018-21736-9
Zhang Y., Han R. (2019). Insight into the salivary secretome of Varroa destructor and salivary toxicity to Apis cerana. J. Econ. Entomol 112, 505–514. doi: 10.1093/jee/toy224
Keywords: Varroa destructor, varroosis, virus, honey bees, gene expression, behavior, immunity
Citation: Morfin N, Goodwin PH and Guzman-Novoa E (2023) Varroa destructor and its impacts on honey bee biology. Front. Bee Sci. 1:1272937. doi: 10.3389/frbee.2023.1272937
Received: 04 August 2023; Accepted: 21 September 2023;
Published: 12 October 2023.
Edited by:
David De Jong, University of Sao Paulo, BrazilReviewed by:
Mustafa Necati Muz, Namik Kemal University, TürkiyeCopyright © 2023 Morfin, Goodwin and Guzman-Novoa. This is an open-access article distributed under the terms of the Creative Commons Attribution License (CC BY). The use, distribution or reproduction in other forums is permitted, provided the original author(s) and the copyright owner(s) are credited and that the original publication in this journal is cited, in accordance with accepted academic practice. No use, distribution or reproduction is permitted which does not comply with these terms.
*Correspondence: Nuria Morfin, bnVyaWFtb3JmaW5AdHRwLWJjaHBhLmNh
Disclaimer: All claims expressed in this article are solely those of the authors and do not necessarily represent those of their affiliated organizations, or those of the publisher, the editors and the reviewers. Any product that may be evaluated in this article or claim that may be made by its manufacturer is not guaranteed or endorsed by the publisher.
Research integrity at Frontiers
Learn more about the work of our research integrity team to safeguard the quality of each article we publish.