- Department of Chemical and Materials Engineering, New Mexico State University, Las Cruces, NM, United States
To address the alerting issue of energy demand, lithium-ion capacitors (LICs) have been widely studied as promising electrochemical energy storage devices, which can deliver higher energy density than supercapacitors (SCs), and have higher power density with longer cycling life than lithium-ion batteries (LIBs). In this work, the active material lithium nickel cobalt manganese oxide LiNi0.5Co0.2Mn0.3O2 (NCM523) is grown on a cotton textile template and building a 3-dimensional (3D) integrity to improve capacitance and energy density of LICs by enhancing the interfacial ion-exchange process. With the 3D structure, the specific discharge capacitance is increased to 718.67
1 Introduction
The consumption and the demand for conventional energies are increasing along with the alerting issue of greenhouse gas emissions (Su et al., 2015; Yuan et al., 2016; Supply, 2021). In response, different energy storage devices have been developed to store and utilize clean renewable energies (engineering, 2017). However, such devices remain inferior to the ideal performance of the traditional combustion engine (Gnanomat, 2019). Taking supercapacitors (SCs) as an example, they are recognized with high power density yet compromised energy density (Education, 2018; Sedajová et al., 2020). Instead, lithium-ion capacitors (LICs) are considered as promising electrochemical energy storage devices, which can deliver higher energy density than SCs and have higher power density with longer cycling life than lithium-ion batteries (LIBs).
The theory is proposed based on the charge-storage mechanism of LIB and SC. Specifically, when a battery is discharged, lithium ions are released from the anode and intercalate into the vacant sites at the cathode’s lattice structure (Lee and Im, 2018). Despite the sluggish intercalation process, it allows LIBs to reach higher energy density (Vlad et al., 2014). On the contrary, SCs have high power density thanks to their rapid charge-discharge process, but the electrochemical reactions only occur on the electrode’s surface causing low energy density (Kötz and Carlen, 2000; Lewandowski and Galinski, 2007; Sharma and Bhatti, 2010). As a result, hybridizing the LIB’s cathode active materials (CAMs) with SC’s electrochemical double-layered capacitor (EDLC) component is expected to render a synergistic effect to control both the energy and power densities, (Vlad et al., 2014; Hagen et al., 2018; Zhao et al., 2020), which is named LICs. Up to now, there have been some promising progress reported. For instance, an energy density of more than 3 times that a conventional supercapacitor can reach has been achieved by employing Li4Ti5O12 (LTO) with graphite (Amatucci et al., 2001; Chen et al., 2012). However, the hybridization remains under-optimized due to the low power characteristic caused by poor Li+ diffusion coefficient and poor electronic conductivity (Kuwata et al., 2016). In response, with the incorporation of textiles to build a 3D skeleton, the problems of Li+ diffusion and ion exchange are substantially mitigated through increased porosity and surface area.
In this work, to further enhance their electrochemical performances of capacitance and energy density, LiNi0.5Co0.2Mn0.3O2 (NCM523) was incorporated with cotton textiles during the synthesis process to construct a 3D integrity. The NCM523 is selected to inherit the good cycle performance and to avoid cation mixing in Ni-rich NCM materials, while the incorporation of textiles during the synthesis process would expedite the interfacial ion-exchange processes to improve the energy density (Muto et al., 2009; Zhao et al., 2020; Zhao and Li, 2020). From which, a new record of energy density (28.26 Wh kg−1) has been written while maintaining the same level of power density (6,000 W kg−1) at a high current rate of 10 A g−1.
2 Experimental section
2.1 Preparation of NCM523
The polymer solution was prepared by mixing ethylenediaminetetraacetic acid (EDTA) and polyethylenimine (PEI, 50% in water) under a ratio of 1:2 in 20 mL of deionized (DI) water. Then, LiOH, Ni (CH3COO)2∙6H2O, Co (CH3COO)2∙6H2O, and Mn (CH3COO)2∙6H2O (1.5:0.5:0.2:0.3) were added into the polymer solution once it reached homogeneity. 50% excess of lithium was applied to account for its evaporation during the high-temperature annealing process. Cotton textiles were soaked in the solution as templates for 3D NCM523 synthesis, and the mixture was kept stirring at 80
2.2 Characterizations
Thermo-gravimetric analysis (TGA—Thermogravimetric Analyzer Model 500 from the TA Instrument) was conducted to find the appropriate annealing temperature. The material morphology and phase structure were analyzed using Scanning Electron Microscope (SEM—Hitachi Model S-3400N II) and X-ray diffraction (XRD—Empyrean XRD PANalytical). The surface area and composition of elements in electrode material were examined by Brunauer–Emmett–Teller (BET—Micromeritics ASAP 2050 High-Pressure Sorption Analyzer) and Inductively Coupled Plasma (ICP—Octopole Reaction System (ORS) Agilent 7500ce with a CETAC XS520 autosampler).
2.3 Electrochemical measurements
Ni-foams were pre-treated with HCl and washed with ethanol and acetone, which were dried at 80
The measurements were conducted using the three-electrode configuration (3EC) to study exclusively the electrochemical properties of the textile NCM523 and the two-electrode configuration (2EC) to evaluate the full-cell capacitor’s performance. In the 3EC, the textile NCM523 or non-textile NCM523 was the working electrode, a platinum foil was used as the counter electrode, and Ag/AgCl was used as the reference electrode. For the 2EC, the full cell was assembled by hybridizing the cathode of NCM523 with the anode of activated carbon (AC).
Cyclic Voltammetry (CV), Galvanostatic Charge-Discharge (GCD), and Electrochemical Impedance (EIS) were performed at room temperature using the CHI 660E electrochemical workstation. The voltage window was −0.1–0.5 V for the half-cell cathode, and −0.8 to 0.1 V for the anode, and different voltage windows were applied for the full cell (0–0.9 V, 0–1 V, 0–1.1 V, and 0–1.2 V). The stability was tested using GCD for 200 cycles. The specific discharge capacitance (C,
Where I (A) is the response current, ν (V s−1) is the scan rate, m is the mass loading of the NCM 523 and textile NCM523, ΔV (V) is the voltage window, j (A mg−1) is the current density and Δt (s) is the discharging time.
Here, m± and C± represent the active material mass and specific capacitance of the positive (or negative) electrode, respectively, ΔE± is the potential window of the positive (or negative) electrode.
Where C is specific capacitance, ΔV (V) is the voltage window.
Here, E is energy density and Δt is the discharge time (s).
3 Results and discussion
3.1 Material characterization
To investigate processes during calcination, thermogravimetric analysis (TGA) and derivative thermogravimetric (DTG) analysis were conducted. The TGA was performed in a range between room temperature and 900
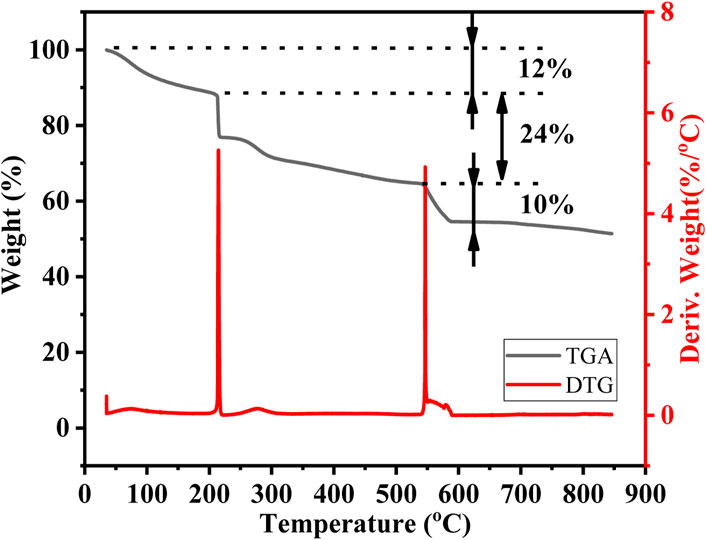
Figure 2. Thermogravimetric analysis (TGA) and derivative thermogravimetric (DTG) curves of the NCM523 precursor solution.
The crystallinity and phase purity can be examined by the X-ray diffraction (XRD) patterns. Figure 3A compares the XRD patterns among the textile and non-textile NCM523 with the standard single-layered hexagonal crystal structure of lithium cobalt oxide (LiCoO2). All the peak positions are almost aligned with the standard pattern despite a slight left shift because the dopants of Ni and Mn induces an expansion in lattice parameters of the crystal structure. The lattice parameters are tabulated in Table 2. The splitting of (108/110) and (006/102) peaks indicates a highly ordered hexagonal crystal structure (Xu et al., 2015; Meng et al., 2017). The integrated intensity ratio of the (003) and (104) are >1.4, indicating the suppressed cation mixing (Xu et al., 2015; Yabuuchi et al., 2011; Yang et al., 2014). The BET surface area was obtained by the isotherm curves as shown in Figure 3B. The textile NCM523 attains the surface area of 5.3
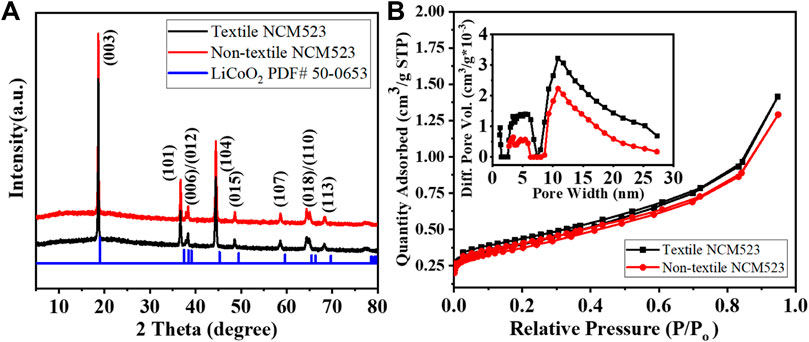
Figure 3. (A) XRD patterns of the NCM523 samples and the standard LiCoO2; and (B) N2 adsorption and desorption isotherm curves, inserted for pore size distribution.
The 3D structure is revealed in the SEM images. As shown in Figure 4A, the NCM523 material replicates the shape of the textiles forming a 3D interwoven network whereas the control sample fails to construct any specific shape. The particle distribution is also more even compared to that of the non-textile NCM523, as shown in Figures 4B–D. In addition, non-textile NCM523 presents some large particles attributing to the lower surface area.
3.2 Electrochemical measurements
3.2.1 Half-cell capacitor (3EC)
To further investigate the electrochemical behavior of the active materials, the relevant tests were carried out including CV, GCD, and EIS in the 1 M KOH three-electrode systems at room temperature. Figure 5A compares the CVs of both samples at a scan rate of 1
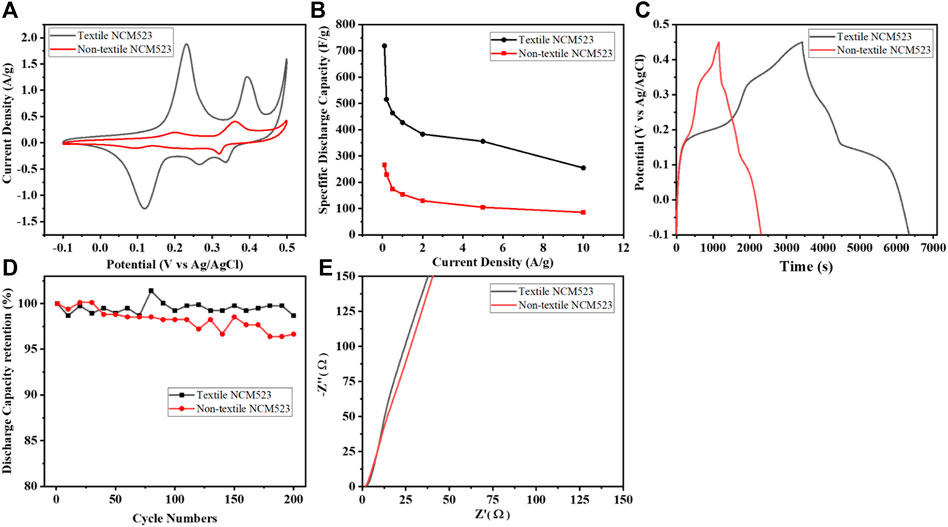
Figure 5. Electrochemical measurement results: (A) CV profile at the scan rate of 1
Textile NCM523 electrode exhibited larger CV integrated areas than that of the non-NCM 523 electrode, indicating the introduction of 3D structure can improve the charge storage capacity of the electrode materials. GCDs were conducted at various current densities (0.1, 0.2, 0.5, 1, 2, 5, and 10
3.2.2 Full-cell capacitor (2EC)
AC with a mass load of 1
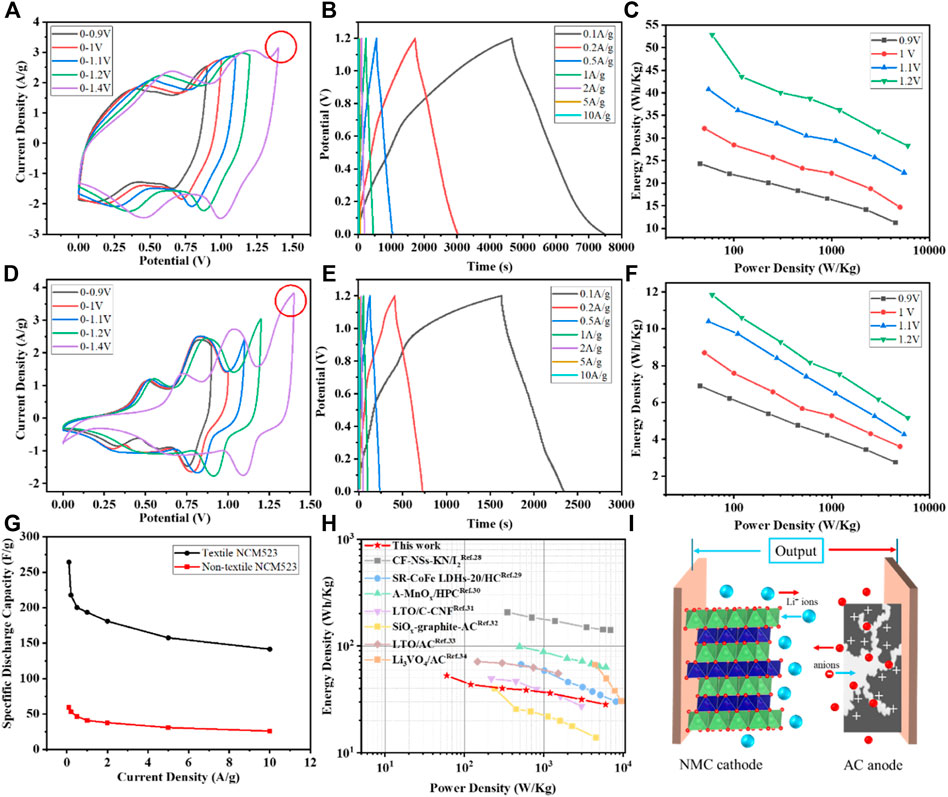
Figure 6. Electrochemical measurement results: (A) CV profiles at different voltage windows of the textile NCM523; (B) GCD profiles at different current densities of the textile NCM523; (C) Ragone plot of the textile NCM523; (D) CV profiles at different voltage windows of the non-textile NCM523; (E) GCD profiles at different current densities of the non-textile NCM523; (F) Ragone plot of the non-textile NCM523; (G) Capacitance at difference current densities for textile and non-textile NCM523; and (H) Ragone plot with the performance of the textile NCM523 edited from literature (Xu et al., 2014; Shen et al., 2017; Li et al., 2019a; Li et al., 2019b; Babu et al., 2020; Sun et al., 2023; Wang et al., 2023; Wang et al., 2024); (I) Schematic diagram of LIC using NMC cathode and AC anode.
4 Conclusion
The 3D NCM523 was successfully synthesized with suppressed cation mixing and facilitated the ion exchange process at the interface. In addition, the incorporation of 3D integrity allows to reach a higher capacitance for the half-cell capacitor while improving the energy density of the full-cell capacitor. Not only is the electrochemical performance dominant over the non-textile NCM523, but it also surpasses the existing LIC’s materials making it a promising choice for ultra-performance energy storage devices.
Data availability statement
The raw data supporting the conclusion of this article will be made available by the authors, without undue reservation.
Author contributions
PT: Writing–original draft, Methodology, Data curation. QS: Writing–original draft, Methodology, Investigation, Formal Analysis, Data curation. WT: Writing–review and editing, Validation, Investigation, Formal Analysis. RH: Writing–review and editing, Validation, Methodology, Investigation, Formal Analysis. MZ: Writing–review and editing, Writing–original draft, Validation, Supervision, Resources, Project administration, Methodology, Investigation, Funding acquisition, Formal Analysis, Conceptualization. HL: Writing–review and editing, Validation, Supervision, Resources, Project administration, Methodology, Investigation, Conceptualization.
Funding
The author(s) declare that financial support was received for the research, authorship, and/or publication of this article. The work is supported by the U.S. National Science Foundation OIA-2119688.
Conflict of interest
The authors declare that the research was conducted in the absence of any commercial or financial relationships that could be construed as a potential conflict of interest.
Publisher’s note
All claims expressed in this article are solely those of the authors and do not necessarily represent those of their affiliated organizations, or those of the publisher, the editors and the reviewers. Any product that may be evaluated in this article, or claim that may be made by its manufacturer, is not guaranteed or endorsed by the publisher.
References
Amatucci, G. G., Badway, F., Du Pasquier, A., and Zheng, T. (2001). An asymmetric hybrid nonaqueous energy storage cell. J. Electrochem. Soc. 148 (8), A930. doi:10.1149/1.1383553
Babu, B., Simon, P., and Balducci, A. (2020). Fast charging materials for high power applications. Adv. Energy Mater. 10 (29), 2001128. doi:10.1002/aenm.202001128
Chen, S., Hu, H., Wang, C., Wang, G., Yin, J., and Cao, D. (2012). LiFePO4-AC/Li4Ti5O12 hybrid supercapacitor: the effect of LiFePO4 content on its performance. J. Renew. Sustain. Energy 4 (3), 033114. doi:10.1063/1.4727929
Du, H., Li, Y., Ding, F., Zhao, J., Zhang, X., Li, Y., et al. (2018). Boosting the capacitance of NiCo2O4 hierarchical structures on nickel foam in supercapacitors. Int. J. Hydrogen Energy 43 (32), 15348–15357. doi:10.1016/j.ijhydene.2018.06.079
Education, E. (2018). Energy density vs power density. Available at: https://energyeducation.ca/encyclopedia/Energy_density_vs_power_density.
engineering (2017). Case school of engineering, C. W. R. U. Electrochemical devices. Available at: https://engineering.case.edu/chemical-and-biomolecular-engineering/research/electrochemical-devices.
Gnanomat (2019). Gnanomat innovative energy storage systems. Available at: https://gnanomat.com/2019/05/16/innovative-energy-storage-systems/.
Hagen, M., Cao, W., Shellikeri, A., Adams, D., Chen, X., Brandt, W., et al. (2018). Improving the specific energy of Li-Ion capacitor laminate cell using hybrid activated Carbon/LiNi0. 5Co0. 2Mn0. 3O2 as positive electrodes. J. Power Sources 379, 212–218. doi:10.1016/j.jpowsour.2018.01.036
Kötz, R., and Carlen, M. (2000). Principles and applications of electrochemical capacitors. Electrochimica acta 45 (15-16), 2483–2498. doi:10.1016/s0013-4686(00)00354-6
Kuwata, N., Lu, X., Miyazaki, T., Iwai, Y., Tanabe, T., and Kawamura, J. (2016). Lithium diffusion coefficient in amorphous lithium phosphate thin films measured by secondary ion mass spectroscopy with isotope exchange methods. Solid State Ionics 294, 59–66. doi:10.1016/j.ssi.2016.06.015
Lee, S.-H., and Im, I.-H. (2018). Excellent performance hybrid supercapacitors based on LiNi1/3Mn1/3Co1/3O2/activated carbon electrode. Mater. Lett. 231, 38–42. doi:10.1016/j.matlet.2018.07.115
Lewandowski, A., and Galinski, M. (2007). Practical and theoretical limits for electrochemical double-layer capacitors. J. Power Sources 173 (2), 822–828. doi:10.1016/j.jpowsour.2007.05.062
Li, C., Zhang, X., Wang, K., Sun, X., and Ma, Y. (2019a). A 29.3 Wh kg− 1 and 6 kW kg− 1 pouch-type lithium-ion capacitor based on SiOx/graphite composite anode. J. Power Sources 414, 293–301. doi:10.1016/j.jpowsour.2018.12.090
Li, H., Guo, S., Wang, L., Wu, J., Zhu, Y. J., and Hu, X. (2019b). Thermally durable lithium-ion capacitors with high energy density from all hydroxyapatite nanowire-enabled fire-resistant electrodes and separators. Adv. Energy Mater. 9 (46), 1902497. doi:10.1002/aenm.201902497
Meng, K., Wang, Z., Guo, H., and Li, X. (2017). Enhanced cycling stability of LiNi0. 8Co0. 1Mn0. 1O2 by reducing surface oxygen defects. Electrochimica Acta 234, 99–107. doi:10.1016/j.electacta.2017.03.054
Muto, S., Sasano, Y., Tatsumi, K., Sasaki, T., Horibuchi, K., Takeuchi, Y., et al. (2009). Capacity-fading mechanisms of LiNiO2-based lithium-ion batteries: II. diagnostic analysis by electron microscopy and spectroscopy. J. Electrochem. Soc. 156 (5), A371. doi:10.1149/1.3076137
Peng, L., Zhu, Y., Khakoo, U., Chen, D., and Yu, G. (2015). Self-assembled LiNi1/3Co1/3Mn1/3O2 nanosheet cathodes with tunable rate capability. Nano Energy 17, 36–42. doi:10.1016/j.nanoen.2015.07.031
Sedajová, V., Jakubec, P., Bakandritsos, A., Ranc, V., and Otyepka, M. (2020). New limits for stability of supercapacitor electrode material based on graphene derivative. Nanomaterials 10 (9), 1731. doi:10.3390/nano10091731
Sharma, P., and Bhatti, T. (2010). A review on electrochemical double-layer capacitors. Energy Convers. Manag. 51 (12), 2901–2912. doi:10.1016/j.enconman.2010.06.031
Shen, L., Lv, H., Chen, S., Kopold, P., van Aken, P. A., Wu, X., et al. (2017). Peapod-like Li3VO4/N-doped carbon nanowires with pseudocapacitive properties as advanced materials for high-energy lithium-ion capacitors. Adv. Mater. 29 (27), 1700142. doi:10.1002/adma.201700142
Su, X., Yu, L., Cheng, G., Zhang, H., Sun, M., and Zhang, X. (2015). High-performance α-MnO2 nanowire electrode for supercapacitors. Appl. Energy 153, 94–100. doi:10.1016/j.apenergy.2014.07.094
Sun, Z., Han, X., and Wang, D. (2023). Zinc-iodine battery-capacitor hybrid device with excellent electrochemical performance enabled by a robust iodine host. J. Energy Storage 62, 106857. doi:10.1016/j.est.2023.106857
Supply, E. (2021). Energy supply evolves to meet demand projections. Available at: https://corporate.exxonmobil.com/Energy-and-innovation/Outlook-for-Energy/Energy-supply#Naturalgas.
Vlad, A., Singh, N., Rolland, J., Melinte, S., Ajayan, P., and Gohy, J.-F. (2014). Hybrid supercapacitor-battery materials for fast electrochemical charge storage. Sci. Rep. 4 (1), 4315–4317. doi:10.1038/srep04315
Wang, D., Han, X., and Zhang, X. (2024). Achieving high-capacity aqueous calcium-ion storage in amorphous manganese oxide nanospheres for calcium-ion asymmetric supercapacitors. J. Power Sources 599, 234215. doi:10.1016/j.jpowsour.2024.234215
Wang, D., Sun, J., and Chen, L. (2023). Structural reconstruction strategy enables CoFe LDHs for high-capacity NH4+ storage and application in high-energy density ammonium-ion hybrid supercapacitors. ChemSusChem 16 (12), e202300207. doi:10.1002/cssc.202300207
Xu, H., Hu, X., Sun, Y., Luo, W., Chen, C., Liu, Y., et al. (2014). Highly porous Li4Ti5O12/C nanofibers for ultrafast electrochemical energy storage. Nano Energy 10, 163–171. doi:10.1016/j.nanoen.2014.09.003
Xu, M., Chen, Z., Zhu, H., Yan, X., Li, L., and Zhao, Q. (2015). Mitigating capacity fade by constructing highly ordered mesoporous Al2O3/polyacene double-shelled architecture in Li-rich cathode materials. J. Mater. Chem. A 3 (26), 13933–13945. doi:10.1039/c5ta03676c
Yabuuchi, N., Yoshii, K., Myung, S.-T., Nakai, I., and Komaba, S. (2011). Detailed studies of a high-capacity electrode material for rechargeable batteries, Li2MnO3−LiCo1/3Ni1/3Mn1/3O2. J. Am. Chem. Soc. 133 (12), 4404–4419. doi:10.1021/ja108588y
Yang, X., Wang, D., Yu, R., Bai, Y., Shu, H., Ge, L., et al. (2014). Suppressed capacity/voltage fading of high-capacity lithium-rich layered materials via the design of heterogeneous distribution in the composition. J. Mater. Chem. A 2 (11), 3899–3911. doi:10.1039/c3ta14513a
Yuan, C., Lin, H., Lu, H., Xing, E., Zhang, Y., and Xie, B. (2016). Synthesis of hierarchically porous MnO2/rice husks derived carbon composite as high-performance electrode material for supercapacitors. Appl. Energy 178, 260–268. doi:10.1016/j.apenergy.2016.06.057
Zhao, D., and Li, S. (2020). Regulating the performance of lithium-ion battery focus on the electrode-electrolyte interface. Front. Chem. 8, 821. doi:10.3389/fchem.2020.00821
Keywords: lithium-ion capacitor (LIC), 3D structure, lithium nickel cobalt manganese oxide (NCM), energy density, battery cathode material
Citation: Truong P, Sun Q, Tang W, He R, Zhou M and Luo H (2024) Morphology modification of LiNi0.5Co0.2Mn0.3O2 by incorporating cotton textiles in lithium-ion capacitors. Front. Batteries Electrochem. 3:1388494. doi: 10.3389/fbael.2024.1388494
Received: 19 February 2024; Accepted: 15 April 2024;
Published: 01 May 2024.
Edited by:
Wei Tong, Berkeley Lab (DOE), United StatesReviewed by:
Dewei Wang, North Minzu University, ChinaBapi Bera, University of Tennessee, Knoxville, United States
Copyright © 2024 Truong, Sun, Tang, He, Zhou and Luo. This is an open-access article distributed under the terms of the Creative Commons Attribution License (CC BY). The use, distribution or reproduction in other forums is permitted, provided the original author(s) and the copyright owner(s) are credited and that the original publication in this journal is cited, in accordance with accepted academic practice. No use, distribution or reproduction is permitted which does not comply with these terms.
*Correspondence: Hongmei Luo, aGx1b0BubXN1LmVkdQ==
†These authors have contributed equally to this work