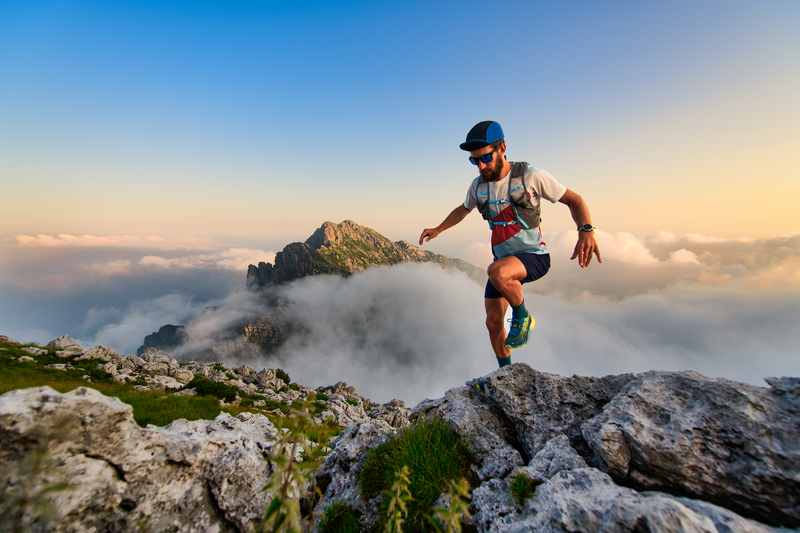
95% of researchers rate our articles as excellent or good
Learn more about the work of our research integrity team to safeguard the quality of each article we publish.
Find out more
MINI REVIEW article
Front. Batter. Electrochem. , 14 February 2024
Sec. Next Generation Batteries and Technologies
Volume 3 - 2024 | https://doi.org/10.3389/fbael.2024.1358199
This article is part of the Research Topic Women in Battery Science and Technology View all 13 articles
Rechargeable magnesium (Mg) batteries are promising candidates for the next-generation of energy storage systems due to their potential high-energy density, intrinsic safety features and cost-effectiveness. Among the various electrochemical couples, the combination of an Mg anode with a sulfur (S) cathode stands out as an attractive option, as it offers a remarkable theoretical volumetric energy density exceeding 3,200 Wh L–1. However, owing to the unique properties of Mg-ion electrolytes, Mg polysulfides and the surface passivation of Mg metal anodes, the development of Mg–S batteries is facing multiple challenges. In this review, recent advancements in designing efficient electrolytes for Mg–S battery systems are summarized. Apart from electrolytes, we also discuss the progress made in fabricating new S cathode composites, Mg anodes and functional separators, focusing on their roles in addressing the critical issues of the Mg–S systems. Finally, it is worth pointing out that the collaborative research combining experimental investigations and theoretical modelling could provide deeper insights into the mechanisms of Mg–S battery systems and promote their development. Overall, the comprehensive insights about the S-redox reaction, polysulfide shuttle problems and degradation mechanism in Mg–S batteries are discussed, which is of profound importance for creating solutions to enhance the overall performance of Mg–S batteries. This review aims to providing an overview of the current state of the research to stimulate innovative thoughts on the fundamental guidelines for facilitating development of Mg–S batteries.
The increasing demand for high-performance, sustainable and safe energy storage systems has prompted researchers to explore rechargeable battery systems that go beyond traditional lithium (Li)-ion batteries. In this context, the promise of magnesium (Mg) batteries as a post-Li battery solution becomes evident, given the high abundance of Mg in the Earth’s crust as well as in seawater, rendering it a more sustainable and scalable energy storage option. Besides, Mg batteries also show many other advantages, including high volumetric energy density, low-cost and environmental friendliness. Besenhard and Winter, (2002); Aurbach et al. (2007); Zhang et al. (2019) Notably, the application of magnesium–sulfur (Mg–S) batteries has attracted substantial attention as a prospective solution for next-generation energy storage. Zhirong and Maximilian, (2017); Wang and Buchmeiser, (2019); Montenegro et al. (2021).
Sulfur possesses the advantages of abundance, low-cost and non-toxicity, while Mg can serve as a safe metal anode, thereby augmenting the value of this battery system. Additionally, owing to its high theoretical capacity, S cathode enables Mg–S batteries to achieve a remarkable gravimetric (1,684 Wh kg–1) and volumetric energy density (3,221 Wh L–1). Zu and Li, (2011); Yu and Manthiram, (2020) The fundamental working principle of Mg–S batteries is based on the electrochemical conversion between S and Mg sulfide (MgS), as illustrated in Equations 1, 2:
During discharge, Mg2+ ions migrate from the anode to the cathode through the electrolyte and react with S8 to form MgS. Conversely, during charge, MgS transforms to Mg2+ ions and S8. Robba et al. (2017) While the overall conversion process seems relatively straightforward, the underlying electrochemical reactions involve a series of intricate steps (Equations 3–7), which may adversely influence the reversibility of the S/MgS conversion process. Therefore, Mg–S batteries face critical technical issues such as serious self-discharge, rapid capacity degradation, and poor cell life due to the well-known “polysulfide shuttle” phenomenon. Hu et al. (2018); Richter et al. (2021); Vincent et al. (2023) During the discharge, the solid-state S8 can be reduced to the long-chain polysulfide MgSn, (n=4–8), as shown in Equations 3–5.
MgSn (n=4–8) species are found to be soluble in organic electrolytes and may diffuse across the separator towards the Mg anode. Further, they may be reduced on Mg surface both electrochemically and chemically, leading to a loss of active material and anode passivation. However, some dissolved polysulfides can migrate back to the S cathode. Gao et al. (2018a); Laskowski et al. (2021) This continuous back-and-forth migration between cathode and anode is referred as polysulfide shuttle effect similar to other metal-sulfur systems, which has been demonstrated and investigated, for example, in Li–S battery systems. Barai et al. (2016); Dysart et al. (2016); Chen et al. (2017); Liu et al. (2017); Mistry and Mukherjee, (2017); Mistry and Mukherjee, (2018); Srinivasan et al. (2022) In order to address this issue, various strategies have been employed, including the optimization/modification of electrolytes, the design of novel S cathode structures, and the implementation of functional separators, which will be discussed in respective sections within this review.
The first proof-of-concept for Mg–S batteries was carried out in 2011. Kim et al. (2011)as shown in Figure 1. Due to the electrophilic nature of S, a non-nucleophilic electrolyte is essential for rechargeable Mg–S batteries. Through the incorporation of the Lewis acid AlCl3 into hexamethyldisilazide magnesium chloride (HMDSMgCl) electrolyte, a non-nucleophilic complex electrolyte [Mg2Cl3•6THF][HMDSAlCl3] was synthesized. This electrolyte exhibited reversible Mg stripping-plating behavior at room temperature with a Coulombic efficiency of 90%. Importantly, the electrolyte is chemically compatible with the S/carbon black cathode, as evidenced by the reversible charge/discharge curves of the Mg–S cells. In 2015, a new synthetic approach to the non-nucleophilic HMDS-based electrolytes was introduced for the application in Mg–S batteries. Zhao-Karger et al. (2015) A one-step reaction in different ethers, including diglyme and tetraglyme, was employed to optimize the electrolyte properties. Additionally, the ionic liquid N-methyl-N-butylpiperidinium bis(trifluoromethanesulfonyl)imide (PP14TFSI) was introduced as an additive to the electrolytes. When the S/CMK-3 cathode composites were combined with the modified electrolyte, a discharge potential closely approached the theoretical value of 1.65 V was achieved in the first cycle, along with a capacity of approximately 800 mAh g–1. However, the practical application of these electrolytes is hindered by their limited long-term stability, primarily attributed to the corrosive nature of chloride ions. In 2017, a new type of non-corrosive fluorinated alkoxyborate based Mg electrolyte Mg tetrakis(hexafluoroisopropyloxy)borate (Mg[B(hfip)4]2) was developed, which consisted of the weakly coordinating anion B(hfip)4– dissolved in glymes. Zhao-Karger et al. (2017) This electrolyte demonstrated remarkable oxidative stability (∼4 V) and high Coulombic efficiency (>98%). Initial tests with an S/CMK-3 cathode revealed a discharge voltage plateau at around 1.5 V and initial capacities of 400 mAh g–1. Besides, an analogous aluminum-based salt was also synthesized and reported in this study. It can be inferred that the strategy of designing new Mg salts by incorporating different fluorinated alkoxyborate anions was proved a valuable approach for further optimizing the electrolyte properties, especially in the context of Mg–S batteries. In the same year, researchers also employed an electrolyte solution comprising a blend of magnesium fluoride and tris(2H-hexafluoroisopropyl) borate in DME, which can generate the initial fluoro-tris(2H-hexafluoroisopropyl)borate BF(hfip)3– anion prior to cycling and the same fluorinated boron-centered B(hfip)4– anion during cycling. Zhang et al. (2017) While this electrolyte exhibited favorable compatibility with both S and selenium (Se) cathodes, its oxidative stability was slightly lower (∼3.5 V) when compared to the application of a Mg[B(hfip)4]2 solution. In Mg–S batteries, a flat discharge voltage plateau at approximately 1.1 V versus Mg was observed. The capacities consistently reached over 1,000 mAh g–1 during the initial 30 cycles, owing to the beneficial effect of the Cu current collector. This aspect was further elaborated upon in subsequent research endeavors. Robba et al. (2020) Later, the investigation of the Mg[B(hfip)4]2 salt was expanded, including simplifying the synthesis, optimizing the composition and examining the electrolyte-electrode interfaces. Zhao-Karger et al. (2018) With the updated electrolyte, Mg–S cell achieved an initial discharge capacity close to 1,000 mAh g–1. However, the capacity declined in the next 30 cycles to around 400 mAh g–1, due to the Mg polysulfide dissolution. To further solve the polysulfide problem, a gel polymer electrolyte (GPE) based on this Mg[B(hfip)4]2 salt was prepared by an in situ polymerization method. Wang L. P. et al. (2022) Galvanostatic cycling in a symmetric cell showed an efficient plating/stripping behavior of Mg over 500 cycles with a very low electrochemical overpotential (<0.06 V). More importantly, by trapping solvent molecules in a polymerized gel network, GPE may suppress the dissolution as well as retard the migration of polysulfides. Enabled by the GPE, both Mg−S coin cells and the prototype pouch cell were assembled. Moreover, the extremely stable open-circuit voltage (OCV) over 48 h validates that the GPE can suppress the dissolution as well as the diffusion of polysulfide, avoiding the serious self-discharge behavior shown in liquid electrolytes. GPEs provide new inspiration for the development of high-performance Mg–S batteries. However, there are still residual solvents inside GPEs, which potentially affecting the cycling stability. In this case, the development of solid-state electrolytes stands as a promising avenue to address polysulfide shuttle-related challenges, offering enhanced cycling stability for Mg–S batteries. Additionally, there is another direction: designing self-standing polymer electrolytes and using them as separators to simplify the battery manufacturing process. Chen et al. (2023).
FIGURE 1. The development progress of rechargeable Mg–S batteries with different electrolytes: in 2011 the first studies with chloride containing complex electrolytes (e.g., Mg(HMDS)2-AlCl3, MgCl2-AlCl3) in various solvents/ionic liquids. (Reproduced with permission Kim et al. (2011); Zhao-Karger et al. (2015); Yang et al. (2019). Copyright 2014, Wiley-VCH. Copyright 2019, American Chemical Society); in 2017 investigations with chloride free electrolyte single-salts such as Mg[B(hfip)4]2 and Mg(TFSI)2 Zhang et al. (2017); Zhao-Karger et al. (2017); Gao et al. (2018b) (Copyright 2017, Wiley-VCH); in 2018 further investigation with the combinations based on commercially available salts (e.g., Mg(TFSI)2, Mg(OTf)2) and optimization of the application of the chloride free single-salt Mg[B(hfip)4]2 (Reproduced with permission. Zhao-Karger et al. (2018); Xu et al. (2019); Yang et al. (2019); Zou et al. (2021); Wang L. P. et al. (2022); Sheha et al. (2022) Copyright 2022, American Chemical Society).
Apart from synthesizing novel Mg electrolyte salts, efforts were made to explore the application of readily available commercial salts, such as Mg(TFSI)2, Gao et al. (2017); Zou et al. (2021) Mg(OTf)2, Yang et al. (2019) MgCl2, Xu et al. (2019) or Mg(NO3)2•6H2O. Sheha et al. (2022) High-donor-number solvents such as DMSO and DMF were explored as potential alternatives to achieve polysulfide mediation in Mg–S batteries. Gao et al. (2018b) Additives including different metal chlorides, Gao et al. (2018b); Xu et al. (2019) and Li salts (Yang et al., 2019; Zou et al., 2021) were applied to improve the reversibility of Mg plating/stripping. Another attempt at formulating an electrolyte involved the use of Mg(NO3)2 in a mixture of acetonitrile and tetraglyme as the solvent. Sheha et al. (2022) Additionally, a polymer interlayer containing polyvinylidene fluoride (PVDF) and Mg triflate was employed to protect the Mg surface from passivation. This study suggests future possibilities for the advancement of halogen-free Mg electrolytes, aligning with the long-term objective of Mg battery development. Additionally, employing electrolyte additives proves to be an effective strategy for performance enhancement; however, the choice of additives must still adhere to criteria such as non-corrosiveness, sustainability and cost-effectiveness.
The electrochemical kinetics of conversion-type S cathodes are influenced by two primary factors: the speed of charge transfer during S redox reactions as well as the specific surface area. Due to the inherently low electrical conductivity of S (∼10–28 S cm−1), a large amount of conductive additives are needed to enable fast redox reactions Li et al. (2023). Among these additives, carbon is the most commonly employed, which can offer both robust electronic conductivity and high specific surface area. In previous reports, various kinds of carbon based materials were used including Ketjenblack, Häcker et al. (2021); Ji et al. (2021); Zou et al. (2021); Häcker et al. (2023) activated carbon cloth (ACC), Gao et al. (2015); Zhao-Karger et al. (2018); Wang L. P. et al. (2022); Wang et al. (2023a) CMK-3, Zhao-Karger et al. (2015); Wu et al. (2021); Yang et al. (2023) CNF, Yu and Manthiram, (2016) CNT, Du et al. (2017) and graphene. Vinayan et al. (2016); Li et al. (2016).
However, owing to the low affinity between nonpolar carbon and polar polysulfides, there is a risk of polysulfides diffusion into the electrolyte during the cycling, which leads to the irreversible depletion of active materials. Introducing heteroatom doping into carbon-based materials is a promising strategy to enhance their affinity for polysulfides and restrain the polysulfide shuttle. Vinayan et al. (2019) reported a nitrogen-doped hybrid nanocomposite of multiwall carbon nanotubes (MWCNTs) and graphene, serving as the host matrix. This composite demonstrated robust adsorption capabilities for polysulfides, resulting in an enhanced cycling stability. Even at a high S loading of 3 mg cm−2, a level unprecedented in Mg–S batteries, the cell demonstrated a stable cycling behavior with 53% capacity retention after 50 cycles. A similar concept was applied by Lee and his colleagues. Lee et al. (2022) They employed nitrogen-doped mesoporous carbon (NdMC) materials as host for S. With the help of the surface nitrogen atoms, NdMC effectively facilitated polysulfide fragmentation and accelerated the S redox reactions, enabling the cells to demonstrate improved reversible capacity and capacity retention in Mg–S batteries. Recently, a novel 3D composite conductive framework consisting of MXene and reduced graphene oxide (rGO) was presented. Guo et al. (2023) Besides, TiO2 nanoparticles were uniformly distributed inside the composite. Within the structure, the nanoconfined TiO2 can provide catalytic active sites for polysulfides, which greatly improves the reversibility of S conversion in hybrid Mg/Li–S batteries. Table 1 summarizes the electrochemical performance of various Mg–S batteries recently reported in the literatures for easy comparison .
In addition to utilizing polar conductive host matrices, notable efforts are also directed towards reducing the mobility of polysulfide species within the cathode by forming covalent bonding between the S species and their hosts. A prime example are sulfurized poly(acrylonitrile) (PAN) composites, NuLi et al. (2007); Wang et al. (2020a); Wang et al. (2020b); Wang et al. (2021); Zhang et al. (2022) which have been thoroughly investigated and are widely employed as cathode material. The preparation of S@PAN composites includes mixing PAN with an excess of S, then subjecting the mixture to controlled heating, which varies in the range of 350°C–550°C across different publications. During this process, elemental S sublimates, generating free radicals, that can covalently bound to the matrix. The covalent bonding proves highly effective in capturing Mg polysulfides and preventing the polysulfide shuttle. However, it is important to acknowledge that the thermal stability of covalently bonded materials may not be optimal, carrying the potential risk of structural damage during the diffusion of molten S. Furthermore, the mechanism and the redox pathway are more complicated, which needs in-depth post mortem analysis to get a comprehensive understanding. Until now, most research works have incorporated hybrid electrolytes containing Li ions as mediator to enhance the kinetics of S@PAN cathode. For this reason, it is important to mitigate the substantial overpotential observed during the solid-state conversion into the final product (MgS) in pure Mg system. These aspects should be carefully considered in the efforts of S cathodes design. Besides, the loading of S in most reported works is still at quite low level (∼1 mg cm⁻2), which is far below the requirements for practical applications. The primary goal in the design of S cathodes remains ensuring a high loading of active materials while maintaining effective utilization of S.
Apart from cathode materials, there are several important challenges and unanswered questions related to the surface and interfacial processes of the Mg anode within Mg–S batteries. While it is widely acknowledged that Li ion batteries can form solid electrolyte interfaces (SEI) on the anode, allowing for Li ion transport; (Cheng et al., 2017); Mg batteries, on the contrary, encounter challenges arising from the formation of a blocking interface on the Mg surface. This layer may hinder Mg ion transport and cause uneven Mg stripping/plating, detrimentally affecting battery efficiency and stability. Lu et al. (1999); Aurbach et al. (2001); Jayasyaee et al. (2019); Li et al. (2020); Bieker et al. (2021) This influence becomes particularly prominent in the presence of polysulfides, resulting in a pitting corrosion mechanism in Mg–S batteries. Bracamonte et al. (2023) The dissolved polysulfides shuttle through the separator, react with Mg metal and form numerous pits on the anode surface, which detrimentally affects the electrochemical performance of the cell. Therefore, research on the Mg anode is equally crucial, even though it may occasionally receive less attention when compared to the cathode and electrolyte.
In reported Mg–S batteries, currently used anode materials are mainly metallic Mg discs Gao et al. (2017); Kaland et al. (2021); Kaland et al. (2021) and foils. Zhao-Karger et al. (2015); Ford et al. (2018); Zhao-Karger et al. (2018); Xu et al. (2019); Bosubabu et al. (2021); Ji et al. (2021); Laskowski et al. (2021); Wang L. et al. (2022) The surface area of Mg discs or foils is typically restricted. Furthermore, prior to cell assembly, it is common to use a scalpel to remove the oxidation layer from the Mg surface. However, this process may unavoidably create an uneven surface, leading to variable electrochemical reactivity. Consequently, this non-uniformity contributes to the uneven deposition and dissolution of Mg. Liu et al. (2022) By replacing the Mg metal anode with MgxM (M= Bi, Sn, Si, Ga … ) alloy that is compatible with conventional electrolytes, could be a possible solution to circumvent these issues in some extent. Yaghoobnejad Asl et al. (2018); Niu et al. (2020); Wang et al. (2023b) However, the alloy anode may lower energy density on the cell level and cause larger volume change. To enhance the performance of Mg metal, different Mg anodes featuring expanded and uniform surface areas have been explored. For example, fine Mg particles were dispersed in carbon and pressed into an anode pellet, Sievert et al. (2017) with increased surface area controlled by the applied pressure. The cells with these pressed anodes demonstrated higher Coulombic efficiencies, extended cycle lifespans, and reduced Ohmic resistances compared to cells using Mg foil anodes. However, finely dispersed Mg particles may strongly react with oxygen in air so that passivation may occur when handling the materials. Later, a 3D-structured Mg anode based on an activated carbon cloth current collector, noted as Mg@ACC, was reported. Wang et al. (2023a) Through an electrodeposition process, Mg@ACC was prepared without the risk of air exposure. The robust carbon matrix can regulate a uniform Mg electrodeposition with a reduced nucleation barrier, bringing a much-reduced initial overpotential of 0.16 V in the Mg–Mg symmetric cells. Both long cycle life and low resistances were also obtained in Mg–S batteries. The excellent electrochemical performance manifests that the 3D framework is beneficial for accelerating charge transfer and homogenizing the metal deposits by providing a higher surface area and a lower current density. More importantly, the enlarged surface area guarantees sufficient electrochemically active sites, thus avoiding the detrimental passivation of Mg anode in Mg–S cells and increasing the cycle life significantly. However, the passivation issue remains because a high-surface area electrode is potentially more reactive toward the electrolyte and polysulfides. Recently, Häcker et al. employed an organic artificial SEI to hinder the contact of dissolved S species, Häcker et al. (2023) protecting the Mg metal anode from the parasitic reactions on the surface. Authors chose a combination of sulfonated tetrafluoroethylene-based copolymer (Aquivion) and poly(vinylidene fluoride) (PVDF) to merge ionic conductivity with mechanical robustness. Remarkably, Mg–S cells that use Mg-Aquivion/PVDF coated anodes exhibit a significant increase in both discharge capacity and initial Coulombic efficiency compared to cells that use pristine Mg foil anodes (as listed in Table 1). However, the ex situ formed artificial SEI resulted in increased overpotential and initial impedance in Mg–Mg symmetric cells, implying a higher migration barrier caused by the SEI layer. The results suggest that it is crucial to find an artificial SEI that has high ionic conductivity and low Mg2+ migration barrier.
The most promising characteristic of Mg–S batteries is their remarkable energy density, which can only be unlocked through the application of Mg metal anodes. Optimizing the performance of the metal anode requires engineering improvements for both the anodes and their interfaces. From our perspective, finding flexible Mg-ion conductive polymers with dual capabilities of self-healing and good ionic conductivity is a compelling option for serving as a protective coating layer or artificial SEI on the Mg anode. Apart from that, to enhance the energy density at the cell level, it is essential to manufacture thin Mg anodes. This is crucial for mitigating the excessive mass associated with the conventional metal foil anodes, which typically have a thickness of approximately 100 µm and beyond. One of the most promising approaches is utilizing pre-magnesiated substrates through methods such as sputter coating and galvanostatic deposition.
Between cathodes and anodes, separators play a vital role in ionic conduction and electronic insulation, influencing both the electrochemical performance and overall safety. As shown in Table 1, glass fiber separators, typically with a thickness of several hundred micrometers, are predominantly utilized in reported Mg–S battery systems. However, as we progress towards broader applications of Mg–S battery technology, particularly on a larger scale or at pouch cell levels, it becomes crucial to reduce the separator thickness and, consequently, the volume of electrolyte. Montenegro et al. (2021) Therefore, it is necessary to screen thinner separators that can maintain the battery’s cycling stability at the same time. Moreover, it should be noted that in Mg–S batteries, separators also play an important role in preventing the migration of polysulfides towards Mg anode. The use of a functionalized separator can enhance the overall performance of the battery. Chen et al. (2023).
For example, researchers have coated decavanadate-based polyoxometalate (POM) clusters/carbon on glass fiber separator by electrospinning. Ji et al. (2021) Analogous to the mechanism of heteroatom doping in cathode materials, the carbon matrix can effectively immobilize polysulfides, while the vanadate component catalyze polysulfide conversion. By incorporating this modified separator, the cycling stability of Mg–S batteries enhanced (listed in Table 1). Later, Mo6S8, the benchmark cathode material, was attempted as a modification layer for the Celgard separator in Mg–S batteries. Wang L. et al. (2022) A combined DFT calculation and experimental study manifested that the Mo6S8 layer could offer both a powerful chemical affinity for polysulfide adsorption and a catalytic effect on accelerating the transformation of polysulfides. Moreover, the separator was prepared via a facile slurry-casting process, which is adaptable for scaling up. With Mo6S8 coated separator, the prototype Mg–S pouch cells were fabricated and exhibited promising cycling stability over 100 cycles. Recently, a copper phosphide (Cu3P) modified functional separator (Celgard 2,400) was prepared also via a doctor blade casting method. Yang et al. (2023) As described, Cu3P could facilitate a rapid catalytic transformation of polysulfides into short-chain MgS2/MgS, attributed to the chemical binding of polysulfides at the oxidation state surface (Cu−O−P) of Cu3P nanoparticles. Remarkably, the cells can achieve a long cycle life up to 500 cycles at 0.5C, which is unprecedented in Mg−S batteries. Overall, functionalization of separators proves to be an effective approach to minimize self-discharge and realize stable cycling in Mg–S batteries. Furthermore, the modification can be applied to thinner separators such as Celgard to avoid the short circuit, which is an important step for advancing pouch cell development.
Modeling on multiple scales enables a better understanding of limiting and beneficial processes in Mg–S batteries and thereby, greatly supports and accelerates experimental research. Over the last few years, more and more atomistic studies have complemented experimental efforts. For instance, structures and stabilities of species in various electrolytes were analyzed including MgSn intermediates. Rajput et al. (2015); Baskin and Prendergast, (2016); Sa et al. (2016); Salama et al. (2016); Samuel et al. (2017); Vinayan et al. (2019); Kopač Lautar et al. (2020); Muthuraj et al. (2021); Jankowski et al. (2022); Jiang et al. (2023) Moreover, atomistic modeling has often been applied to investigate materials, which can strongly adsorb polysulfides and thereby, mitigate adverse shuttle effects and/or catalyze polysulfide conversion. Zhao et al. (2021); Wang L. et al. (2022); Paul et al. (2023); Yang et al. (2023).
In contrast, there are only few approaches to describe Mg plating and stripping as well as full Mg battery cells on continuum scale. Chadwick et al. (2016); DeWitt et al. (2016); Kim et al. (2016); Drews et al. (2020); Richter et al. (2020); Drews et al. (2021); Richter et al. (2021); Drews et al. (2023) First continuum simulations were reported in 2015 and focus on the 3D Mg deposition morphology. DeWitt et al. (2016) Shortly after, Chadwick et al. proposed a 1D model for Mg plating and stripping on a noble substrate. They aimed towards an adequate parameterization, especially regarding the electrochemical kinetics, by fitting simulated to experimentally measured CVs. Chadwick et al. (2016) Although these early continuum simulations provide valuable insights, the models are based on many simplifications. For instance, transport in the electrolyte was described solely by diffusion and dilute solution theory, respectively. Chadwick et al. (2016); DeWitt et al. (2016) Nevertheless, follow-up work was not reported until half a decade later. The recent continuum models are based on the thermodynamic consistent transport theory and do not assume an ideal solution without interactions between dissolved species. Latz and Zausch, (2011) Drews et al. explicitly considered Mg-characteristic phenomena, such as ion aggregation and desolvation prior to electron transfer, as well as the state-of-the-art electrolyte based on Mg[B(hfip)4]2. Drews et al. (2020) However, the effect of polysulfides on transport properties and electrochemical kinetics of Mg deposition has not been properly investigated yet.
The first continuum model of a full Mg–S cell was presented just 3 years ago. Richter et al. generalized a well-established Li–S model and studied differences of self-discharge and degradation effects between the Mg–S and the Li–S systems. Richter et al. (2020); Richter et al. (2021) The work focused on the behavior of the S species and therefore, no significant differences between Mg and Li dissolution/deposition kinetics were considered and ions were assumed to be fully dissociated in the electrolyte. Nonetheless, the model coupled the complex conversion and precipitation reactions of S species to their transport in the electrolyte, with side reactions on the anode surface and resulting passivation being considered. Moreover, the impact of spatial confinement by encapsulating S into a porous carbon structure was included by a 1+1D approach, which described transport and reactions on the macroscopic cell level as well as on the microscopic particle level. Kinetics of undesired side reactions were found to be significantly faster for Mg than for Li, leading to a more rapid self-discharge during storage under OCV and fast, irreversible growth of passivating MgS during the first cycle. One reason therefore might be the absence of a protective SEI on the Mg metal. Therefore, formation of a suitable artificial SEI is a promising strategy to mitigate negative effects of the polysulfide shuttle. Moreover, dissolution of S is found to be remarkably faster in the Mg electrolyte, so that S leaves the carbon host very quickly. Since all S dissolves, there is no driving force for a predominant precipitation inside the carbon host and instead S is formed on the particle surface. In addition, the pronounced polysulfide shuttle prevents reoxidation of a significant amount of S species during charge. Both effects contribute to a strong capacity fading during cycling.
Overall, there are numerous instances where theory and experiments mutually enrich each other, and the synergistic collaboration between experiments, DFT calculations, and continuum simulations fosters a more comprehensive comprehension of crucial and constraining processes in Mg batteries. Vinayan et al. (2019); Richter et al. (2020); Drews et al. (2021); Muthuraj et al. (2021); Richter et al. (2021); Zhao et al. (2021); Wang L. et al. (2022); Jankowski et al. (2022); Paul et al. (2023); Yang et al. (2023) However, reports on joint theoretical and experimental studies - especially on adequate continuum models and comprehensive simulations - are still rare for the Mg–S system and a joint effort will be key to address remaining challenges.
Due to their complex chemistry, the development of Mg–S batteries remains at the laboratory level. Challenges associated with polysulfide shuttle, uneven Mg metal deposition and interface passivation still need to be addressed holistically. Combining the approaches of electrolyte modification, cathode design and anode surface engineering would be necessary for improving the electrochemical performance, efficiency, and lifespan of Mg–S batteries. Moreover, a profound comprehension of the underlying electrochemical and chemical processes through collaborative experimental and theoretical research is required. It can provide guidance for the development of the battery components, including electrolyte, electrode materials and separators, with the goal of enhancing the overall performance and reliability of Mg–S batteries.
LW: Writing–original draft, Writing–review and editing. SR: Writing–original draft, Writing–review and editing. JD: Writing–original draft, Writing–review and editing. ZZ-K: Writing–review and editing.
The author(s) declare financial support was received for the research, authorship, and/or publication of this article. This work contributes to the research performed at CELEST (Center for Electrochemical Energy Storage Ulm-Karlsruhe) and was funded by the German Research Foundation (DFG) under Project ID 390874152 (POLiS Cluster of Excellence, EXC 2154). We acknowledge support by the KIT-Publication Fund of the Karlsruhe Institute of Technology.
The authors declare that the research was conducted in the absence of any commercial or financial relationships that could be construed as a potential conflict of interest.
All claims expressed in this article are solely those of the authors and do not necessarily represent those of their affiliated organizations, or those of the publisher, the editors and the reviewers. Any product that may be evaluated in this article, or claim that may be made by its manufacturer, is not guaranteed or endorsed by the publisher.
Aurbach, D., Gizbar, H., Schechter, A., Chusid, O., Gottlieb, H. E., Gofer, Y., et al. (2001). Electrolyte solutions for rechargeable magnesium batteries based on organomagnesium chloroaluminate complexes. J. Electrochem. Soc. 149 (2), A115–A121. doi:10.1149/1.1429925
Aurbach, D., Suresh, G. S., Levi, E., Mitelman, A., Mizrahi, O., Chusid, O., et al. (2007). Progress in rechargeable magnesium battery technology. Adv. Mater. 19 (23), 4260–4267. doi:10.1002/adma.200701495
Barai, P., Mistry, A., and Mukherjee, P. P. (2016). Poromechanical effect in the lithium–sulfur battery cathode. Extreme Mech. Lett. 9, 359–370. doi:10.1016/j.eml.2016.05.007
Baskin, A., and Prendergast, D. (2016). Exploration of the detailed conditions for reductive stability of Mg(TFSI)2 in diglyme: implications for multivalent electrolytes. J. Phys. Chem. C 120 (7), 3583–3594. doi:10.1021/acs.jpcc.5b08999
Besenhard, J. O., and Winter, M. (2002). Advances in battery technology: rechargeable magnesium batteries and novel negative-electrode materials for lithium ion batteries. ChemPhysChem 3 (2), 155–159. doi:10.1002/1439-7641(20020215)3:2<155::aid-cphc155>3.0.co;2-s
Bieker, G., Küpers, V., Kolek, M., and Winter, M. (2021). Intrinsic differences and realistic perspectives of lithium-sulfur and magnesium-sulfur batteries. Commun. Mat. 2 (1), 37. doi:10.1038/s43246-021-00143-0
Bosubabu, D., Li, Z., Meng, Z., Wang, L.-P., Fichtner, M., and Zhao-Karger, Z. (2021). Mitigating self-discharge and improving the performance of Mg–S battery in Mg[B(hfip)4]2 electrolyte with a protective interlayer. J. Mat. Chem. A 9 (44), 25150–25159. doi:10.1039/d1ta06114c
Bracamonte, M. V., Vizintin, A., Kapun, G., Cometto, F., Bitenc, J., Randon-Vitanova, A., et al. (2023). Surface film formation on Mg electrode containing magnesium polysulfides in TFSI-based electrolytes. J. Power Sources 555, 232367. doi:10.1016/j.jpowsour.2022.232367
Chadwick, A. F., Vardar, G., DeWitt, S., Sleightholme, A. E. S., Monroe, C. W., Siegel, D. J., et al. (2016). Computational model of magnesium deposition and dissolution for property determination via cyclic voltammetry. J. Electrochem. Soc. 163 (9), A1813–A1821. doi:10.1149/2.0031609jes
Chen, C.-F., Mistry, A., and Mukherjee, P. P. (2017). Probing impedance and microstructure evolution in lithium–sulfur battery electrodes. J. Phys. Chem. C 121 (39), 21206–21216. doi:10.1021/acs.jpcc.7b07245
Chen, S., Wang, Y., Sun, Y., Zhang, D., Zhang, S., Zhao, Y., et al. (2023). Research status and prospect of separators for magnesium-sulfur batteries. J. Energy Chem. 87, 225–246. doi:10.1016/j.jechem.2023.08.020
Cheng, X.-B., Zhang, R., Zhao, C.-Z., and Zhang, Q. (2017). Toward safe lithium metal anode in rechargeable batteries: a review. Chem. Rev. 117 (15), 10403–10473. doi:10.1021/acs.chemrev.7b00115
DeWitt, S., Hahn, N., Zavadil, K., and Thornton, K. (2016). Computational examination of orientation-dependent morphological evolution during the electrodeposition and electrodissolution of magnesium. J. Electrochem. Soc. 163 (3), A513–A521. doi:10.1149/2.0781603jes
Drews, J., Danner, T., Jankowski, P., Vegge, T., García Lastra, J. M., Liu, R., et al. (2020). Modeling of ion agglomeration in magnesium electrolytes and its impacts on battery performance. ChemSusChem 13 (14), 3599–3604. doi:10.1002/cssc.202001034
Drews, J., Jankowski, P., Häcker, J., Li, Z., Danner, T., García Lastra, J. M., et al. (2021). Modeling of electron-transfer kinetics in magnesium electrolytes: influence of the solvent on the battery performance. ChemSusChem 14 (21), 4820–4835. doi:10.1002/cssc.202101498
Drews, J., Wiedemann, J., Maça Alaluf, R. R., Wang, L., Blázquez, J. A., Zhao-Karger, Z., et al. (2023). Modeling of magnesium intercalation into chevrel phase Mo6S8: report on improved cell design. Batter. Supercaps 6 (5), e202200562. doi:10.1002/batt.202200562
Du, A., Zhang, Z., Qu, H., Cui, Z., Qiao, L., Wang, L., et al. (2017). An efficient organic magnesium borate-based electrolyte with non-nucleophilic characteristics for magnesium–sulfur battery. Energy Environ. Sci. 10 (12), 2616–2625. doi:10.1039/c7ee02304a
Dysart, A. D., Burgos, J. C., Mistry, A., Chen, C.-F., Liu, Z., Hong, C. N., et al. (2016). Towards next generation lithium-sulfur batteries: non-conventional carbon compartments/sulfur electrodes and multi-scale analysis. J. Electrochem. Soc. 163 (5), A730–A741. doi:10.1149/2.0481605jes
Ford, H. O., Merrill, L. C., He, P., Upadhyay, S. P., and Schaefer, J. L. (2018). Cross-linked ionomer gel separators for polysulfide shuttle mitigation in magnesium–sulfur batteries: elucidation of structure–property relationships. Macromolecules 51 (21), 8629–8636. doi:10.1021/acs.macromol.8b01717
Gao, T., Hou, S., Huynh, K., Wang, F., Eidson, N., Fan, X., et al. (2018a). Existence of solid electrolyte interphase in Mg batteries: Mg/S chemistry as an example. ACS Appl. Mat. Interfaces 10 (17), 14767–14776. doi:10.1021/acsami.8b02425
Gao, T., Hou, S., Wang, F., Ma, Z., Li, X., Xu, K., et al. (2017). Reversible S0/MgSx redox chemistry in a MgTFSI2/MgCl2/DME electrolyte for rechargeable Mg/S batteries. Angew. Chem. Int. Ed. 56 (43), 13526–13530. doi:10.1002/anie.201708241
Gao, T., Ji, X., Hou, S., Fan, X., Li, X., Yang, C., et al. (2018b). Thermodynamics and kinetics of sulfur cathode during discharge in MgTFSI2–DME electrolyte. Adv. Mater. 30 (3), 1704313. doi:10.1002/adma.201704313
Gao, T., Noked, M., Pearse, A. J., Gillette, E., Fan, X., Zhu, Y., et al. (2015). Enhancing the reversibility of Mg/S battery chemistry through Li+ mediation. J. Am. Chem. Soc. 137 (38), 12388–12393. doi:10.1021/jacs.5b07820
Guo, M., Yuan, C. Y., Xu, T., Zhong, S. L., Wang, W. B., Zou, T. X., et al. (2023). In situ built nanoconfined TiO2 particles in robust-flexible MXene@rGO conductive framework enabling high-performance hybrid magnesium-sulfur batteries. Adv. Energy Mater. 13 (26), 2300417. doi:10.1002/aenm.202300417
Häcker, J., Nguyen, D. H., Rommel, T., Zhao-Karger, Z., Wagner, N., and Friedrich, K. A. (2021). Operando UV/vis spectroscopy providing insights into the sulfur and polysulfide dissolution in magnesium–sulfur batteries. ACS Energy Lett. 7 (1), 1–9. doi:10.1021/acsenergylett.1c02152
Häcker, J., Rommel, T., Lange, P., Zhao-Karger, Z., Morawietz, T., Biswas, I., et al. (2023). Magnesium anode protection by an organic artificial solid electrolyte interphase for magnesium-sulfur batteries. ACS Appl. Mat. Interfaces 15 (27), 33013–33027. doi:10.1021/acsami.3c07223
Hu, X.-C., Shi, Y., Lang, S.-Y., Zhang, X., Gu, L., Guo, Y.-G., et al. (2018). Direct insights into the electrochemical processes at anode/electrolyte interfaces in magnesium-sulfur batteries. Nano Energy 49, 453–459. doi:10.1016/j.nanoen.2018.04.066
Jankowski, P., Li, Z., Zhao-Karger, Z., Diemant, T., Fichtner, M., Vegge, T., et al. (2022). Development of magnesium borate electrolytes: explaining the success of Mg[B(hfip)4]2 salt. Energy Storage Mater. 45, 1133–1143. doi:10.1016/j.ensm.2021.11.012
Jayasyaee, K., Rerthelot, R., Lethesh, K. C., and Sheridan, E. M. (2019). Anode materials for rechargeable Mg batteries. Magnes. Batter. 114–141. doi:10.1039/9781788016407-00114
Ji, Y. C., Liu-Theato, X., Xiu, Y. L., Indris, S., Njel, C., Maibach, J., et al. (2021). Polyoxometalate modified separator for performance enhancement of magnesium-sulfur batteries. Adv. Funct. Mater. 31 (26), 2100868. doi:10.1002/adfm.202100868
Jiang, X., Wu, J., Zhang, P., Jiang, L., Lu, S., Zhao, X., et al. (2023). First-principles investigation on multi-magnesium sulfide and magnesium sulfide clusters in magnesium-sulfide batteries. RSC Adv. 13 (30), 20926–20933. doi:10.1039/d3ra03165a
Kaland, H., Håskjold Fagerli, F., Hadler-Jacobsen, J., Zhao-Karger, Z., Fichtner, M., Wiik, K., et al. (2021). Performance study of MXene/carbon nanotube composites for current collector- and binder-free Mg–S batteries. ChemSusChem 14 (8), 1864–1873. doi:10.1002/cssc.202100173
Kim, H. S., Arthur, T. S., Allred, G. D., Zajicek, J., Newman, J. G., Rodnyansky, A. E., et al. (2011). Structure and compatibility of a magnesium electrolyte with a sulphur cathode. Nat. Commun. 2 (1), 427. doi:10.1038/ncomms1435
Kim, S. U., Perdue, B., Apblett, C. A., and Srinivasan, V. (2016). Understanding performance limitations to enable high performance magnesium-ion batteries. J. Electrochem. Soc. 163 (8), A1535–A1542. doi:10.1149/2.0321608jes
Kopač Lautar, A., Bitenc, J., Rejec, T., Dominko, R., Filhol, J.-S., and Doublet, M.-L. (2020). Electrolyte reactivity in the double layer in Mg batteries: an interface potential-dependent DFT study. J. Am. Chem. Soc. 142 (11), 5146–5153. doi:10.1021/jacs.9b12474
Laskowski, F. A. L., Stradley, S. H., Qian, M. D., and See, K. A. (2021). Mg anode passivation caused by the reaction of dissolved sulfur in Mg–S batteries. ACS Appl. Mat. Interfaces 13 (25), 29461–29470. doi:10.1021/acsami.1c02788
Latz, A., and Zausch, J. (2011). Thermodynamic consistent transport theory of Li-ion batteries. J. Power Sources 196 (6), 3296–3302. doi:10.1016/j.jpowsour.2010.11.088
Lee, M., Jeong, M., Nam, Y. S., Moon, J., Lee, M., Lim, H.-D., et al. (2022). Nitrogen–doped graphitic mesoporous carbon materials as effective sulfur imbibition hosts for magnesium-sulfur batteries. J. Power Sources 535, 231471. doi:10.1016/j.jpowsour.2022.231471
Li, D., Yuan, Y., Liu, J., Fichtner, M., and Pan, F. (2020). A review on current anode materials for rechargeable Mg batteries. J. Magnesium Alloys 8 (4), 963–979. doi:10.1016/j.jma.2020.09.017
Li, W., Cheng, S., Wang, J., Qiu, Y., Zheng, Z., Lin, H., et al. (2016). Synthesis, crystal structure, and electrochemical properties of a simple magnesium electrolyte for magnesium/sulfur batteries. Angew. Chem. 128 (22), 6516–6520. doi:10.1002/ange.201600256
Li, Z. Y., Hacker, J., Fichtner, M., and Zhao-Karger, Z. (2023). Cathode materials and chemistries for magnesium batteries: challenges and opportunities. Adv. Energy Mater. 13 (27), 2300682. doi:10.1002/aenm.202300682
Liu, X., Du, A., Guo, Z., Wang, C., Zhou, X., Zhao, J., et al. (2022). Uneven stripping behavior, an unheeded killer of Mg anodes. Adv. Mater. 34 (31), 2201886. doi:10.1002/adma.202201886
Liu, Z., Mistry, A., and Mukherjee, P. P. (2017). Mesoscale physicochemical interactions in lithium–sulfur batteries: progress and perspective. J. Electrochem. Energy Convers. Storage 15 (1), 010802. doi:10.1115/1.4037785
Lu, Z., Schechter, A., Moshkovich, M., and Aurbach, D. (1999). On the electrochemical behavior of magnesium electrodes in polar aprotic electrolyte solutions. J. Electroanal. Chem. 466 (2), 203–217. doi:10.1016/s0022-0728(99)00146-1
Mistry, A., and Mukherjee, P. P. (2017). Precipitation–microstructure interactions in the Li-sulfur battery electrode. J. Phys. Chem. C 121 (47), 26256–26264. doi:10.1021/acs.jpcc.7b09997
Mistry, A. N., and Mukherjee, P. P. (2018). Electrolyte transport evolution dynamics in lithium–sulfur batteries. J. Phys. Chem. C 122 (32), 18329–18335. doi:10.1021/acs.jpcc.8b05442
Montenegro, C. T., Peters, J. F., Baumann, M., Zhao-Karger, Z., Wolter, C., and Weil, M. (2021). Environmental assessment of a new generation battery: the magnesium-sulfur system. J. Energy Storage 35, 102053. doi:10.1016/j.est.2020.102053
Muthuraj, D., Pandey, M., Krishna, M., Ghosh, A., Sen, R., Johari, P., et al. (2021). Magnesium polysulfide catholyte (MgSx): synthesis, electrochemical and computational study for magnesium-sulfur battery application. J. Power Sources 486, 229326. doi:10.1016/j.jpowsour.2020.229326
Niu, J., Zhang, Z., and Aurbach, D. (2020). Alloy anode materials for rechargeable Mg ion batteries. Adv. Energy Mater. 10 (23), 2000697. doi:10.1002/aenm.202000697
NuLi, Y., Guo, Z., Liu, H., and Yang, J. (2007). A new class of cathode materials for rechargeable magnesium batteries: organosulfur compounds based on sulfur–sulfur bonds. Electrochem. Commun. 9 (8), 1913–1917. doi:10.1016/j.elecom.2007.05.009
Paul, A., Pandey, M., and Johari, P. (2023). Computational study of adsorption of magnesium polysulfides on VS4 magnesium sulfur batteries. Mater. Today Proc. 76, 352–358. doi:10.1016/j.matpr.2022.11.399
Rajput, N. N., Qu, X., Sa, N., Burrell, A. K., and Persson, K. A. (2015). The coupling between stability and ion pair formation in magnesium electrolytes from first-principles quantum mechanics and classical molecular dynamics. J. Am. Chem. Soc. 137 (9), 3411–3420. doi:10.1021/jacs.5b01004
Richter, R., Häcker, J., Zhao-Karger, Z., Danner, T., Wagner, N., Fichtner, M., et al. (2020). Insights into self-discharge of lithium– and magnesium–sulfur batteries. ACS Appl. Energy Mat. 3 (9), 8457–8474. doi:10.1021/acsaem.0c01114
Richter, R., Häcker, J., Zhao-Karger, Z., Danner, T., Wagner, N., Fichtner, M., et al. (2021). Degradation effects in metal–sulfur batteries. ACS Appl. Energy Mat. 4 (3), 2365–2376. doi:10.1021/acsaem.0c02888
Robba, A., Mežnar, M., Vizintin, A., Bitenc, J., Bobnar, J., Arčon, I., et al. (2020). Role of Cu current collector on electrochemical mechanism of Mg–S battery. J. Power Sources 450, 227672. doi:10.1016/j.jpowsour.2019.227672
Robba, A., Vizintin, A., Bitenc, J., Mali, G., Arčon, I., Kavčič, M., et al. (2017). Mechanistic study of magnesium–sulfur batteries. Chem. Mat. 29 (21), 9555–9564. doi:10.1021/acs.chemmater.7b03956
Sa, N., Rajput, N. N., Wang, H., Key, B., Ferrandon, M., Srinivasan, V., et al. (2016). Concentration dependent electrochemical properties and structural analysis of a simple magnesium electrolyte: magnesium bis(trifluoromethane sulfonyl)imide in diglyme. RSC Adv. 6 (114), 113663–113670. doi:10.1039/c6ra22816j
Salama, M., Shterenberg, I., Gizbar, H., Eliaz, N. N., Kosa, M., Keinan-Adamsky, K., et al. (2016). Unique behavior of dimethoxyethane (DME)/Mg(N(SO2CF3)2)2 solutions. J. Phys. Chem. C 120 (35), 19586–19594. doi:10.1021/acs.jpcc.6b07733
Samuel, D., Steinhauser, C., Smith, J. G., Kaufman, A., Radin, M. D., Naruse, J., et al. (2017). Ion pairing and diffusion in magnesium electrolytes based on magnesium borohydride. ACS Appl. Mat. Interfaces 9 (50), 43755–43766. doi:10.1021/acsami.7b15547
Sheha, E., Farrag, M., Fan, S., Kamar, E., and Sa, N. (2022). A simple Cl–-Free electrolyte based on magnesium nitrate for magnesium–sulfur battery applications. ACS Appl. Energy Mat. 5 (2), 2260–2269. doi:10.1021/acsaem.1c03778
Sievert, B., Häcker, J., Bienen, F., Wagner, N., and Friedrich, K. A. (2017). Magnesium sulfur battery with a new magnesium powder anode. ECS Trans. 77 (11), 413–424. doi:10.1149/07711.0413ecst
Srinivasan, V., and Mistry, A. (2022). “Chapter 6 - modeling of electrode, electrolyte, and interfaces of lithium-sulfur batteries,” in Lithium-sulfur batteries. Editors P. N. Kumta, A. F. Hepp, M. K. Datta, and O. I. Velikokhatnyi (Elsevier), 201–231.
Sun, J., Deng, C., Bi, Y., Wu, K.-H., Zhu, S., Xie, Z., et al. (2020). In situ sulfurized carbon-confined cobalt for long-life Mg/S batteries. ACS Appl. Energy Mat. 3 (3), 2516–2525. doi:10.1021/acsaem.9b02232
Vinayan, B. P., Euchner, H., Zhao-Karger, Z., Cambaz, M. A., Li, Z., Diemant, T., et al. (2019). Insights into the electrochemical processes of rechargeable magnesium–sulfur batteries with a new cathode design. J. Mat. Chem. A 7 (44), 25490–25502. doi:10.1039/c9ta09155f
Vinayan, B. P., Zhao-Karger, Z., Diemant, T., Chakravadhanula, V. S., Schwarzburger, N. I., Cambaz, M. A., et al. (2016). Performance study of magnesium-sulfur battery using a graphene based sulfur composite cathode electrode and a non-nucleophilic Mg electrolyte. Nanoscale 8 (6), 3296–3306. doi:10.1039/c5nr04383b
Vincent, S., Chang, J. H., Canepa, P., and García-Lastra, J. M. (2023). Mechanisms of electronic and ionic transport during Mg intercalation in Mg–S cathode materials and their decomposition products. Chem. Mat. 35 (9), 3503–3512. doi:10.1021/acs.chemmater.2c03784
Wang, L., Diemant, T., Li, Z., Dasari, B., and Zhao-Karger, Z. (2023a). Insights into the degradation mechanism of the magnesium anode in magnesium–chalcogen batteries: revealing principles for anode design with a 3D-structured magnesium anode. ACS Appl. Energy Mat. 6 (2), 1008–1018. doi:10.1021/acsaem.2c03516
Wang, L., Nelson Weker, J., Family, R., Liu, J., and Detsi, E. (2023b). Morphology evolution in self-healing liquid-gallium-based Mg-ion battery anode. ACS Energy Lett. 8, 4932–4940. doi:10.1021/acsenergylett.3c01761
Wang, L., Jankowski, P., Njel, C., Bauer, W., Li, Z., Meng, Z., et al. (2022). Dual role of Mo6S8 in polysulfide conversion and shuttle for Mg-S batteries. Adv. Sci. 9 (7), e2104605. doi:10.1002/advs.202104605
Wang, L. P., Li, Z. Y., Meng, Z., Xiu, Y. L., Dasari, B., Zhao-Karger, Z., et al. (2022). Designing gel polymer electrolyte with synergetic properties for rechargeable magnesium batteries. Energy Storage Mater. 48, 155–163. doi:10.1016/j.ensm.2022.03.006
Wang, P., and Buchmeiser, M. R. (2019). Rechargeable magnesium–sulfur battery technology: state of the art and key challenges. Adv. Funct. Mater. 29 (49). doi:10.1002/adfm.201905248
Wang, P., Kappler, J., Sievert, B., Häcker, J., Küster, K., Starke, U., et al. (2020a). Characteristics of magnesium-sulfur batteries based on a sulfurized poly(acrylonitrile) composite and a fluorinated electrolyte. Electrochimica Acta 361, 137024. doi:10.1016/j.electacta.2020.137024
Wang, P., Trück, J., Niesen, S., Kappler, J., Küster, K., Starke, U., et al. (2020b). High-performance magnesium-sulfur batteries based on a sulfurated poly(acrylonitrile) cathode, a borohydride electrolyte, and a high-surface area magnesium anode. Batter. Supercaps 3 (11), 1239–1247. doi:10.1002/batt.202000097
Wang, P. W., Kuster, K., Starke, U., Liang, C., Niewa, R., and Buchmeiser, M. R. (2021). Performance enhancement of rechargeable magnesium-sulfur batteries based on a sulfurized poly(acrylonitrile) composite and a lithium salt. J. Power Sources 515, 230604. doi:10.1016/j.jpowsour.2021.230604
Wu, D., Ren, W., Yang, Y., Wang, J., and NuLi, Y. (2021). A Se-doped S@CMK3 composite as a high-performance cathode for magnesium–sulfur batteries with Mg2+/Li+ hybrid electrolytes. J. Phys. Chem. C 125 (47), 25959–25967. doi:10.1021/acs.jpcc.1c07324
Xu, Y., Zhou, G., Zhao, S., Li, W., Shi, F., Li, J., et al. (2019). Improving a Mg/S battery with YCl3 additive and magnesium polysulfide. Adv. Sci. 6 (4), 1800981. doi:10.1002/advs.201800981
Yaghoobnejad Asl, H., Fu, J., Kumar, H., Welborn, S. S., Shenoy, V. B., and Detsi, E. (2018). In situ dealloying of bulk Mg2Sn in Mg-ion half cell as an effective route to nanostructured Sn for high performance Mg-ion battery anodes. Chem. Mat. 30 (5), 1815–1824. doi:10.1021/acs.chemmater.7b04124
Yang, Y., Fu, W., Zhang, D., Ren, W., Zhang, S., Yan, Y., et al. (2023). Toward high-performance Mg-S batteries via a copper phosphide modified separator. ACS Nano 17 (2), 1255–1267. doi:10.1021/acsnano.2c09302
Yang, Y., Wang, W., Nuli, Y., Yang, J., and Wang, J. (2019). High active magnesium trifluoromethanesulfonate-based electrolytes for magnesium–sulfur batteries. ACS Appl. Mat. Interfaces 11 (9), 9062–9072. doi:10.1021/acsami.8b20180
Yu, X., and Manthiram, A. (2016). Performance enhancement and mechanistic studies of magnesium–sulfur cells with an advanced cathode structure. ACS Energy Lett. 1 (2), 431–437. doi:10.1021/acsenergylett.6b00213
Yu, X., and Manthiram, A. (2020). A progress report on metal–sulfur batteries. Adv. Funct. Mater. 30 (39), 2004084. doi:10.1002/adfm.202004084
Zhang, S., Ren, W., NuLi, Y., Wang, B., Yang, J., and Wang, J. (2022). Sulfurized-pyrolyzed polyacrylonitrile cathode for magnesium-sulfur batteries containing Mg2+/Li+ hybrid electrolytes. Chem. Eng. J. 427, 130902. doi:10.1016/j.cej.2021.130902
Zhang, Y., Geng, H., Wei, W., Ma, J., Chen, L., and Li, C. C. (2019). Challenges and recent progress in the design of advanced electrode materials for rechargeable Mg batteries. Energy Storage Mater. 20, 118–138. doi:10.1016/j.ensm.2018.11.033
Zhang, Z., Cui, Z., Qiao, L., Guan, J., Xu, H., Wang, X., et al. (2017). Novel design concepts of efficient Mg-ion electrolytes toward high-performance magnesium–selenium and magnesium–sulfur batteries. Adv. Energy Mater. 7 (11), 1602055. doi:10.1002/aenm.201602055
Zhao, Q., Wang, R., Zhang, Y., Huang, G., Jiang, B., Xu, C., et al. (2021). The design of Co3S4@MXene heterostructure as sulfur host to promote the electrochemical kinetics for reversible magnesium-sulfur batteries. J. Magnesium Alloys 9 (1), 78–89. doi:10.1016/j.jma.2020.12.001
Zhao-Karger, Z., Gil Bardaji, M. E., Fuhr, O., and Fichtner, M. (2017). A new class of non-corrosive, highly efficient electrolytes for rechargeable magnesium batteries. J. Mat. Chem. A 5 (22), 10815–10820. doi:10.1039/c7ta02237a
Zhao-Karger, Z., Liu, R., Dai, W., Li, Z., Diemant, T., Vinayan, B. P., et al. (2018). Toward highly reversible magnesium–sulfur batteries with efficient and practical Mg[B(hfip)4]2 electrolyte. ACS Energy Lett. 3 (8), 2005–2013. doi:10.1021/acsenergylett.8b01061
Zhao-Karger, Z., Zhao, X., Wang, D., Diemant, T., Behm, R. J., and Fichtner, M. (2015). Performance improvement of magnesium sulfur batteries with modified non-nucleophilic electrolytes. Adv. Energy Mater. 5 (3), 1401155. doi:10.1002/aenm.201401155
Zhirong, Z. K., and Maximilian, F. (2017). Magnesium–sulfur battery: its beginning and recent progress. MRS Commun. 7 (4), 770–784. doi:10.1557/mrc.2017.101
Zhou, X., Tian, J., Hu, J., and Li, C. (2018). High rate magnesium–sulfur battery with improved cyclability based on metal–organic framework derivative carbon host. Adv. Mater. 30 (7), 1704166. doi:10.1002/adma.201704166
Zou, Q., Sun, Y., Liang, Z., Wang, W., and Lu, Y.-C. (2021). Achieving efficient magnesium–sulfur battery chemistry via polysulfide mediation. Adv. Energy Mater. 11 (31), 2101552. doi:10.1002/aenm.202101552
Keywords: magnesium-sulfur batteries, polysulfide shuttle, electrolyte, sulfur cathode, magnesium anode, separator, continuum simulation
Citation: Wang L, Riedel S, Drews J and Zhao-Karger Z (2024) Recent developments and future prospects of magnesium–sulfur batteries. Front. Batteries Electrochem. 3:1358199. doi: 10.3389/fbael.2024.1358199
Received: 19 December 2023; Accepted: 24 January 2024;
Published: 14 February 2024.
Edited by:
Wei Tong, Berkeley Lab (DOE), United StatesReviewed by:
Daniel Juarez-Robles, University of Guanajuato, MexicoCopyright © 2024 Wang, Riedel, Drews and Zhao-Karger. This is an open-access article distributed under the terms of the Creative Commons Attribution License (CC BY). The use, distribution or reproduction in other forums is permitted, provided the original author(s) and the copyright owner(s) are credited and that the original publication in this journal is cited, in accordance with accepted academic practice. No use, distribution or reproduction is permitted which does not comply with these terms.
*Correspondence: Liping Wang, bGlwaW5nLTEud2FuZ0B1bmktdWxtLmRl; Zhirong Zhao-Karger, emhpcm9uZy56aGFvLWthcmdlckBraXQuZWR1
Disclaimer: All claims expressed in this article are solely those of the authors and do not necessarily represent those of their affiliated organizations, or those of the publisher, the editors and the reviewers. Any product that may be evaluated in this article or claim that may be made by its manufacturer is not guaranteed or endorsed by the publisher.
Research integrity at Frontiers
Learn more about the work of our research integrity team to safeguard the quality of each article we publish.