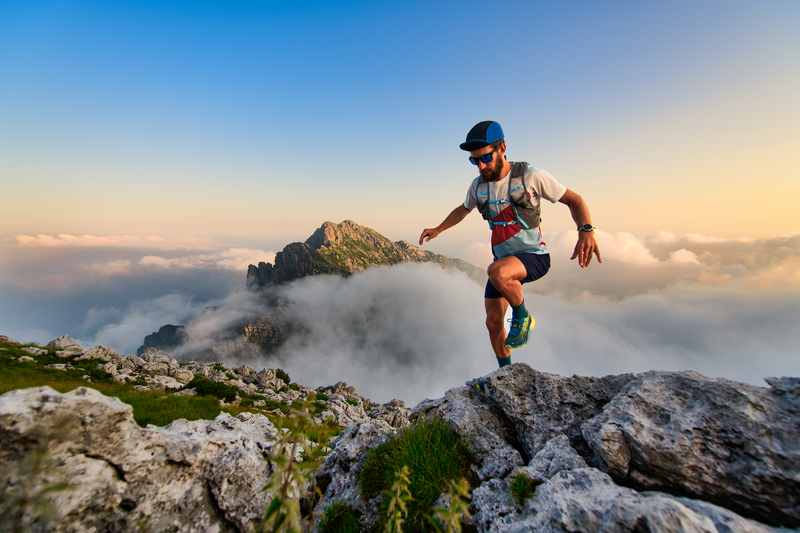
95% of researchers rate our articles as excellent or good
Learn more about the work of our research integrity team to safeguard the quality of each article we publish.
Find out more
BRIEF RESEARCH REPORT article
Front. Bacteriol. , 06 November 2023
Sec. Pathogenesis, Vaccines, and Immunity of Bacterial Infections
Volume 2 - 2023 | https://doi.org/10.3389/fbrio.2023.1303051
This article is part of the Research Topic Editors' Showcase: Pathogenesis, Vaccines, and Immunity of Bacterial Infections View all 8 articles
Burkholderia pseudomallei, the causative agent of melioidosis, has two phases of infection. The acute phase occurs shortly after infection and is associated with bacterial sepsis, potentially leading to death, whilst the chronic phase occurs when infection persists for longer periods or is asymptomatic for months or years. BALB/c mice are more susceptible to melioidosis compared to C57BL/6 mice and are routinely models for the acute phase of infection. However, in some instances when medical countermeasures are being evaluated, mice continue to succumb to disease throughout the course of the experimental infection. Whilst B. pseudomallei is not known to be transmitted from mouse-to-mouse, we hypothesized that mice that have recovered from infection after medical countermeasure intervention may become reinfected from chronically infected mice. We tested this hypothesis by cohousing naïve mice with mice exposed to B. pseudomallei by the inhalational or intraperitoneal routes in either static or ventilated caging. Mice that were exposed to aerosolized B. pseudomallei transmitted the bacterium to approximately 4% of their naïve cagemates, whereas mice that were infected by the intraperitoneal route transmitted to approximately 8% of their naïve cagemates. Whilst the exact route of transmission remains to be determined, the results of this study showed that low levels of mouse-to-mouse transmission of B. pseudomallei are possible. We conclude that although the chance of reinfection is low amongst mice housed in the same cage, this possible scenario should be considered when interpreting data from the BALB/c mouse model of melioidosis in lengthy studies.
Burkholderia pseudomallei is an environmental bacterium and opportunistic pathogen found mainly in the soil and surface water of tropical and subtropical regions. It is the etiologic agent of melioidosis, an emerging public health concern and potential biothreat agent (Cheng and Currie, 2005; Cheng et al., 2005; Currie, 2015; Limmathurotsakul et al., 2016; Dance and Limmathurotsakul, 2018; Wiersinga et al., 2018). Infections with B. pseudomallei are most commonly acquired through inhalation or direct cutaneous inoculation of the organism into a human or animal host (Currie, 2015; Limmathurotsakul, et al., 2016; Dance and Limmathurotsakul, 2018; Wiersinga, et al., 2018). It is a major cause of sepsis and mortality in endemic tropical regions such as Southeast Asia and Northern Australia (Currie, 2015; Limmathurotsakul, et al., 2016; Dance and Limmathurotsakul, 2018; Wiersinga, et al., 2018; Kaewrakmuk et al., 2023). Furthermore, recent advances in the environmental isolation of B. pseudomallei and the increased identification of melioidosis cases in locations such as South Asia, Africa, and the Americas, clearly support the expanded ecological range of B. pseudomallei and its clinical impact (Limmathurotsakul, et al., 2016; Dance and Limmathurotsakul, 2018).
Melioidosis is also being detected in the United States. Most of the cases have been related to travel to areas with endemic melioidosis or to exposure to contaminated imported products (Dawson et al., 2021; Gee et al., 2022; Currie et al., 2023). However, three non-travel-associated cases of melioidosis in Mississippi, which were found to be genetically associated with B. pseudomallei isolated locally from soil and water samples, clearly suggest that B. pseudomallei is endemic in the southern region of the USA (CDC, 2022; Currie, et al., 2023). Similar cases in Texas have been reported that were suggestive of local acquisition; but attempts to isolate a comparable strain from environmental samples were unsuccessful (Cossaboom et al., 2020). Burkholderia thailandensis, an opportunistic pathogen which only rarely causes human infections, is closely related to B. pseudomallei, and is similarly found in the environment. B. thailandensis has been isolated from water in Texas and Puerto Rico and from the soil in Mississippi, demonstrating an environment suitable for Burkholderia species colonization in parts of the Southern United States and in outlying territories (Hall et al., 2023). Interestingly, climate change appears to play a role in the increased range and magnitude of infections by these bacteria (Gassiep et al., 2023).
Melioidosis is a complex disease with manifestations ranging from acute and rapidly fatal to protracted chronic infections. Infected individuals usually display symptoms of acute disease (pneumonia, bacteremia, or localized infection) upon medical evaluation. However, ~9-15% manifest a chronic infection, with symptoms lasting over two months, or are subclinically infected (Cheng et al., 2005; Currie et al., 2010; Currie, 2015; CDC, 2022; Seng et al., 2023). Most patients have coexisting risk factors for the disease including diabetes, excessive alcohol use, chronic lung or renal disease, and other immunocompromising conditions (Currie et al., 2010; Currie, 2015; Limmathurotsakul et al., 2016; Dance and Limmathurotsakul, 2018; Wiersinga et al., 2018; Kaewrakmuk et al., 2023). Several animal models have been developed to study the pathogenesis of melioidosis (Leakey et al., 1998; Conejero et al., 2011; Limmathurotsakul et al., 2015; Welkos et al., 2015; Amemiya et al., 2017; Bearss et al., 2017; Trevino et al., 2018; Trevino et al., 2021; Nelson et al., 2023). The BALB/c is a highly susceptible strain of mice and is typically used to model acute melioidosis; in contrast, the C57BL/6 mouse strain is significantly more resistant, representing a more chronic model of disease which is often used for vaccine evaluation (Leakey et al., 1998; Tan et al., 2008; Lever et al., 2009; Srisurat et al., 2010; Conejero et al., 2011; Massey et al., 2014; Amemiya et al., 2017; Bearss et al., 2017; Trevino et al., 2018; Funnell et al., 2019; Nelson et al., 2023). By varying the dose of B. pseudomallei delivered by aerosolization (a relevant route when evaluating countermeasures for public health or biodefense purposes), both acute and more protracted models of disease have been modelled in BALB/c mice (Srisurat et al., 2010; Bearss et al., 2017; Funnell et al., 2019).
Mice infected with B. pseudomallei can display acute or chronic disease which resembles that of human melioidosis (Tan et al., 2008; Srisurat et al., 2010; Conejero et al., 2011; Massey et al., 2014; Bearss et al., 2017; Burtnick et al., 2018; Amemiya et al., 2019; Klimko et al., 2022). Despite their sensitivity, BALB/c mice (which survive the initial phase of infection due to intervention with medical countermeasures) can continue to succumb to disease throughout the course of the experimental infection. This apparent persistence of the infection is observed despite resolution of symptomatic disease and has been reported in naïve animals as well as those immunized with vaccines and treated with antibiotics. This has been demonstrated, in studies in which the animals are monitored for two to three months post exposure, with bacteria often recovered in tissues collected from survivors, e.g., spleen, liver, and lungs, at the study endpoint. (Conejero et al., 2011; Burtnick et al., 2018; Biryukov et al., 2022; Klimko et al., 2022). This chronic infection state could be attributable to the ability of B. pseudomallei to survive intracellularly, e.g., in pyogranulomatous lesions (Valvano et al., 2005; Finlay and McFadden, 2006; Limmathurotsakul et al., 2006; Conejero et al., 2011; Titball et al., 2017; Avraham, 2023; Seng et al., 2023) potentially facilitated by the formation of multi-nucleated giant cells, antibiotic-resistant biofilms and/or the development of a bacterial ‘persister’ state (Velapatino et al., 2012; Lazar Adler et al., 2013; Butt et al., 2014; Austin et al., 2015; Stockton and Torres, 2020). Alternately, the apparent persistence or relapse of infection may be due to re-infection following clearance of the initial infection.
Our objective was to evaluate the basis of protracted infection with delayed mortality in the BALB/c mouse model. Whilst B. pseudomallei is not typically transmissible (person-to-person spread has rarely been documented) and the bacteria are not known to be readily transmitted between animals (Aziz et al., 2020; Rees et al., 2021), we hypothesized that mice exposed to infection and recovered following treatment could potentially become re-infected by chronically-infected mice (Cheng and Currie, 2005; Cheng et al., 2005; Wiersinga et al., 2018; DPIRD, 2018). In the current study, we tested this hypothesis by cohousing naïve mice with mice exposed to B. pseudomallei by the aerosol or parenteral routes of infection and followed the disease progression for several months. Whilst infrequent, we showed that infected mice can infect naïve mice when cohoused. It is impractical to avoid cohousing laboratory rodents, however, the possibility that animals showing clinical signs of infection can infect other animals must be considered when interpreting in vivo data, particularly from long-duration therapeutics studies.
B. pseudomallei strain K96243 is a fully virulent strain that is commonly used in laboratory studies to assess the efficacy of vaccines or antibiotics (Burtnick et al., 2018; Biryukov et al., 2022). For preparation of the challenge inoculum, a frozen aliquot was grown in 4% glycerol (Sigma Aldrich, St. Louis, MO) with 1% tryptone (Difco, Becton Dickinson, Sparks, MD) and 5% NaCl (Sigma Aldrich, St. Louis, MO) broth (GTB) at 37˚C with shaking at 200 rpm until late log phase, for approximately 16 h (Biryukov et al., 2022). For preparation of mouse challenge doses, the bacteria were harvested, resuspended in GTB for aerosolization or PBS for intraperitoneal delivery and quantified by OD620 estimation (Welkos et al., 2015; Bearss et al., 2017; Biryukov et al., 2022). The actual delivered dose of bacteria, as the number of colony forming units (CFU), was then determined by plate counts on sheep’s blood agar (Trypticase soy agar with sheep blood-SBA) plates (Remel™, Thermo-Fisher Scientific, Waltham, MA) incubated at 37˚C for approximately 48 h.
All animal work was performed under a research protocol approved by the USAMRIID Institutional Animal Care and Use Committee (IACUC). Female BALB/c mice were exposed to aerosolized B. pseudomallei in a whole-body exposure chamber and the inhaled doses calculated from CFU determinations from an all glass impinger using Guyton’s formula (Guyton, 1947a; Guyton, 1947b; Biryukov, et al., 2022). The mice inhaled a dose of approximately 106 CFU (approximately 10 LD50s). Exposed mice (n = 4 per cohort) were then cohoused with naïve mice (n = 4 per cohort) in static or ventilated cages (< 0.2m/sec, 70 air changes per h, exhaust air -55% air exhaust) starting at approximately 1 h or 48 h following exposure to aerosolized bacteria. There were three cages for each parameter tested for a total of 24 mice in each cohort. The differential times used to initiate cohousing would account for bacteria on the fur that would normally be removed by grooming or by potential loss of bacterial viability within 48 h (Shams et al., 2007). The cages were changed every 7 days. The mice were observed daily for 99 days (approximately 60 days after the last exposed mouse had succumbed to disease or was euthanized in accordance with early endpoint criteria). The naïve mice that succumbed had blood collected for antibody analysis by ELISA and organs harvested and plated for bacterial growth. The surviving mice were euthanized at the end of study and the spleens and lungs were sampled for evidence of B. pseudomallei colonization. Briefly, blood was collected from the axillary vessels of deeply anesthetized mice. The mice were then euthanized, the spleens and lungs removed, washed in sterile PBS, weighed and homogenized using tissue grinders (Covidien, Dublin, Republic of Ireland). The organ homogenates were serially diluted and 100 μl of homogenate was used to enumerate bacterial burden on SBA plates, where the limit of detection was approximately 5 CFU per organ.
Female BALB/c mice were infected with approximately 3.8x104 CFU (approximately 1 LD50) of B. pseudomallei via intraperitoneal injection. The experimental design was as described above, without the cohort that was cohoused together 48 h after infection, since there was limited potential for bacteria to be contaminating the fur of these animals. Mice in this study were observed daily for 140 days post infection (approximately 62 days after the last death of an exposed mouse was observed). We observed these mice for a longer period of time postchallenge than the cohort exposed to aerosolized bacteria because the disease course following intraperitoneal inoculation is more protracted than the inhalational route and also because we had two exposed mice surviving the infection. The infected mice (with the exception of 2 infected animals that survived the initial infection) and 2 naïve mice had succumbed to disease or were euthanized before the end of study. Subsets of mice had blood and organs harvested and plated for bacterial growth as described above.
Serum immunoglobulin (Ig) IgG titers in infected mice were determined by ELISA as described by Biryukov et al. (Biryukov et al., 2022). Briefly, sera were harvested from terminal blood collection and assayed for Immunoglobulin G (IgG) antibody levels by semi-quantitative endpoint ELISA in 96-well Immulon 2HB plates (Thermo Fisher). Plates were coated overnight with B. pseudomallei K96243 cells inactivated by approximately 21 kGy of γ-radiation (10 μg/ml) at 4°C as previously described (Biryukov et al., 2022). Two-fold dilutions of serum in PBS/0.05% Tween 20 were made in triplicate, incubated for 30 min at 37°C, then washed and the signal detected as previously described (Biryukov et al., 2022). Results are reported as the geometric mean (GM) and geometric standard deviation (GSD) of the reciprocal of the highest dilution (giving a mean OD of at least 0.1 ± 1 SD at 450 nm (570 nm used as reference wavelength)), then the triplicate sample values were averaged. The limit of detection was a geometric mean of 50, with titer values <50 considered negative. In this study, pooled samples were analyzed and if there was evidence of a possible positive antibody titer then serum samples from individual mice were analyzed.
In this study a total of 48 naïve mice were cohoused with mice that were exposed to aerosolized B. pseudomallei. Naïve mice (n = 4) were introduced to the cages containing the exposed mice (n = 4) immediately after the aerosolization procedure or approximately 48 h later, to help account for any transmission associated with bacteria on the fur after exposure in a whole-body aerosol chamber that would normally be removed by grooming behavior. The USAMRIID routinely utilizes whole-body exposure systems because it reduces the stress associated with the aerosolization procedure, and it increases the number of animals exposed to the same number of bacteria, making statistical power easier to achieve with less variability (Biryukov et al., 2022). We also examined static caging versus actively ventilated caging, hypothesizing that this could be an important parameter when examining transmission rates in infected laboratory rodents. Static cages had no active ventilation. Ventilated cages (Techniplast GR900; Techniplast, West Chester, PA) had an airflow of < 0.2 m/sec in order to prevent perceptible draft conditions within the cage and resulted in 70 air changes per hour.
During the course of this study all animals intentionally exposed to the aerosolized bacteria inhaled approximately 106 CFU (approximately 10 LD50s). Mice in all cages showed clinical signs of melioidosis starting at approximately day two post exposure and all mice that were exposed succumbed to disease or were euthanized by day 39 postchallenge (Figure 1A). The mice were then observed for an additional 60 days (end of study was day 99) during which two naïve mice developed clinical signs indicative of melioidosis, one mouse succumbed to disease on day 41 and another mouse was euthanized due to overt clinical signs on day 72 (Figure 1A). The two naïve mice that succumbed to disease from infected cage-mates represented a transmission rate of approximately 4% and had detectable B. pseudomallei in spleen and lung tissues at high levels (Table 1).
Figure 1 Survival curves of BALB/c mice exposed to B. pseudomallei (filled shapes) and cohoused with naïve mice (open shapes). (A) Infected mice were introduced immediately after exposure to aerosolized bacteria or 48 h later. All mice that were exposed to aerosolized bacteria succumbed to disease or were euthanized in accordance with early endpoint euthanasia criteria by day 39 postchallenge. The survival curves of the infected cohorts are in the bottom left of the graph, while the survival curves of the naïve mice are in the top right of the graph. Note that three of the four naïve cohorts exhibited 100% survival and are all depicted on the top line. Naïve mouse A succumbed to disease on day 41 and naïve mouse B was euthanized on day 72. (B) Survival curves of BALB/c mice infected by the intraperitoneal route with B. pseudomallei (filled shapes) and cohoused with naïve mice (open shapes). Infected mice were introduced immediately following infection. Most of the mice exposed to bacteria (two infected mice in a static cage survived to the end of study) succumbed to disease or were euthanized in accordance with early endpoint euthanasia criteria by day 78 postchallenge. The survival curves of the infected cohorts are in the bottom left of the graph, while the survival curves of the naïve mice are in the top right of the graph. Naïve mouse A succumbed to disease on day 92 and naïve mouse B succumbed on day 95.
Table 1 Bacterial burden in naïve mice that became infected following cohousing with mice exposed to B. pseudomallei.
Interestingly, the two naïve mice that developed disease were housed in the same ventilated cage and the mice were not cohoused until 48 h after exposure to aerosolized bacteria. This result was unexpected as this should have been the group of mice that was least likely to transmit the bacteria because of the 48-h delay in cohousing and the ventilation of the caging. Whilst the occurrence of transmission is too infrequent to reliably know the cause of transmission, there is a chance that the ventilation system may have played a role. Thus, mouse-to-mouse transmission occurs at very low frequency under the experimental conditions described here. Importantly, the remaining surviving naïve mice (46 out of 48) had no detectable bacteria in the lungs and spleens and no evidence of detectable serum IgG levels against B. pseudomallei at the end of the study (day 99 after infected mice were exposed to aerosolized bacteria).
To understand if this mouse-to-mouse transmission was only associated with inhalational melioidosis, we performed a study that cohoused naïve mice with mice that were infected by the intraperitoneal route with approximately 3.8x104 CFU (approximately 1 LD50) of B. pseudomallei (Welkos et al., 2015). The results generated in this study were very similar to those generated in the earlier study. All but two mice that were infected intraperitoneally had succumbed or were euthanized by day 78 (Figure 1B). Two out of 24 naïve mice became infected during the study (within 140 days), both of which were housed in the same ventilated cage (transmission rate of approximately 8%). They had detectable bacteria in the spleen and lungs at high levels (Table 1).
The remaining surviving mice had no detectable bacterial colonization in the lungs and spleens. There was evidence, however, of an antibody response to B. pseudomallei at the end of the study in three naïve mice (Tables 2, 3). It is possible that these mice were infected and resolved the infection or that the infection in these mice became persistent and clinical signs were not observed. It is also possible that the level of bacterial colonization was below the limit of detection (approximately 5 CFU/organ examined), bacteria were located in a tissue that was not assayed in this study, or the bacteria remained in a differentially culturable state (i.e., viable but non-culturable) (Pumpuang et al., 2011; Auty et al., 2022). Of note, one naïve mouse in group 4 had an IgG titer of 3,733 (Table 3) which was higher than the titer determined in two mice that were infected intraperitoneally with B. pseudomallei but survived to the end of study (group 2 infected mice (Table 2) had an average titer of approximately 1,033).
As described in Table 1, the two naïve mice that became infected by their cage-mates that were exposed to B. pseudomallei by the inhalational route had high levels of bacteria in their spleens and lungs. One possibility is that this was a result of a primary pneumonia following an aerosolization or re-aerosolization event (i.e. cage changes or due to the active ventilation system present in the specific cage). It is also possible that it was due to a secondary pneumonia as a result of hematogenous spread following infection by another route (e.g. orogastric).
To understand possible scenarios as to how these two naïve mice became infected, we evaluated the lung burden following parenteral infection. Mice (n = 13) that were infected with B. pseudomallei by the intraperitoneal route had high levels of bacteria in their spleens (1.14x108 CFU/g [ranging from 2.52x103 to 7.42x108 CFU/g]). Importantly, these mice also had substantial bacterial burden in their lungs (2.45x105 CFU/g [ranging from 0 to 2.67x106 CFU/g] which almost certainly resulted from hematogenous spread. Therefore, it is inconclusive as to how B. pseudomallei was transmitted to the naïve mice in the first study (Figure 1A).
Mouse models of melioidosis have been invaluable to understand B. pseudomallei pathogenesis and for the development and evaluation of novel medical countermeasures to treat melioidosis. Importantly, there are several “waves” of clinical illness that can be observed when performing long-duration laboratory evaluations. We evaluated the hypothesis that clinically ill mice could infect naive mice or theoretically reinfect cage mates that had cleared an initial bacterial infection during medical countermeasure evaluations. In this manuscript, we have demonstrated that mouse-to-mouse transmission of B. pseudomallei is infrequent in the laboratory setting, but possible. Whilst not enough naïve mice became infected for statistical analyses, in both studies, transmission was observed in mice housed in ventilated cages. It is tempting to correlate the ventilated caging system with the possible aerosolization of bacteria and subsequent infection of naïve mice, however, it is impossible to draw that conclusion with our current data set. Additionally, there are reports suggesting that rodents housed in ventilated caging systems are the least-likely to transmit infectious diseases to cohoused mice (Compton et al., 2004). As melioidosis is known to be transmitted via ingestion of contaminated material (Sanchez-Villamil et al., 2020; Nelson et al., 2021), we believe that other routes of transmission are possible, including ingestion or inhaling contaminated fecal material or urine and by fomite transmission via the watering system. Additionally, whilst cannibalism of dead mice was not observed in the cages where transmission was observed, it is another possibility for a route of infection if the tissues consumed by cage mates contain B. pseudomallei (Lane-Petter, 1968; Nicklas et al., 2012). We also demonstrated that several naïve cage mates with no clinical signs of melioidosis had anti-B. pseudomallei antibody titers (Tables 2, 3). Whilst it is possible that these mice were colonized with B. pseudomallei at concentrations below the level of detection or in an organ not sampled, the mice had no detectable bacteria in their lungs or spleens. This seroconversion of seemingly healthy mice may be reminiscent of what is reported in humans living in highly endemic areas and the debate regarding the prevalence of subclinical infections in healthy, yet seropositive, individuals (Limmathurotsakul and Peacock, 2011; Nithichanon et al., 2018).
It is our interpretation that this low-level of transmission, reported here, is unlikely to impact the results of vaccination experiments performed with C57BL/6 mice due to their increased resistance to B. pseudomallei, but this assumption has not been investigated (Tan et al., 2008; Srisurat et al., 2010; Conejero et al., 2011; Massey et al., 2014; Bearss et al., 2017; Burtnick et al., 2018; Amemiya et al., 2019; Klimko et al., 2022). This low-level of transmission should be considered when analyzing data obtained from lengthy therapeutic studies using more sensitive animal models (i.e. BALB/c mouse or hamster) (Amemiya et al., 2017; Nelson et al., 2023). Whilst it is impractical to singly house mice for numerous reasons (including ethical reasons) in certain circumstances it may be prudent to isolate animals showing signs of disease to reduce the risk of them infecting their cage mates that may have resolved the infection after medical countermeasure intervention (Wurbel, 2001; Kappel et al., 2017). These extra measures may only be warranted for pivotal studies in cases where medical countermeasures are undergoing advanced development, and even then, it would be difficult to implement for mouse studies.
The original contributions presented in the study are included in the article/supplementary material. Further inquiries can be directed to the corresponding author.
The animal study was approved by The United States Army Medical Research Institute of Infectious Diseases (USAMRIID) Institutional Animal Care and Use Committee (IACUC). The study was conducted in accordance with the local legislation and institutional requirements.
CK: Conceptualization, Data curation, Formal Analysis, Investigation, Methodology, Writing – review & editing. KB: Conceptualization, Writing – review & editing. NR: Investigation, Writing – review & editing. JS: Investigation, Writing – review & editing. JD: Data curation, Formal Analysis, Investigation, Writing – review & editing. MH: Investigation, Writing – review & editing. SW: Writing – original draft. DD: Funding acquisition, Writing – review & editing. SB: Formal Analysis, Investigation, Writing – review & editing. SH: Conceptualization, Writing – review & editing. CC: Conceptualization, Writing – review & editing, Data curation, Formal Analysis, Funding acquisition, Investigation, Project administration, Resources, Supervision, Writing – original draft.
The author(s) declare financial support was received for the research, authorship, and/or publication of this article. This work was funded by the US Defense Threat Reduction Agency (DTRA) under project #CB10794.
The authors declare that the research was conducted in the absence of any commercial or financial relationships that could be construed as a potential conflict of interest.
The author CC declared that they were an editorial board member of Frontiers, at the time of submission. This had no impact on the peer review process and the final decision.
All claims expressed in this article are solely those of the authors and do not necessarily represent those of their affiliated organizations, or those of the publisher, the editors and the reviewers. Any product that may be evaluated in this article, or claim that may be made by its manufacturer, is not guaranteed or endorsed by the publisher.
Opinions, interpretations, conclusions, and recommendations are those of the authors and are not necessarily endorsed by the U.S. Army or the Department of Defense Health Agency. Research was conducted in compliance with the Animal Welfare Act and other federal statutes and regulations relating to animals and experiments involving animals and adheres to principles stated in the Guide for the Care and Use of Laboratory Animals, National Research Council, 2011. The facility where this research was conducted is fully Accredited by the Association for Assessment and Accreditation of Laboratory Animal Care International.
Amemiya K., Bozue J. A., Cote C. K., DeShazer D., Soffler C., Welkos S. L., et al. (2017). Animal models for melioidosis. Curr. Trop. Med. Rep. 4, 208–222. doi: 10.1007/s40475-017-0131-5
Amemiya K., Dankmeyer J. L., Biryukov S. S., Trevino S. R., Klimko C. P., Mou S. M., et al. (2019). Deletion of two genes in Burkholderia pseudomallei MSHR668 that target essential amino acids protect acutely infected BALB/c mice and promote long term survival. Vaccines (Basel) 7 (4), 196. doi: 10.3390/vaccines7040196
Austin C. R., Goodyear A. W., Bartek I. L., Stewart A., Sutherland M. D., Silva E. B., et al. (2015). A Burkholderia pseudomallei colony variant necessary for gastric colonization. mBio 6 (1), e02462-14. doi: 10.1128/mBio.02462-14
Auty J. M., Jenkins C. H., Hincks J., Straatman-Iwanowska A. A., Allcock N., Turapov O., et al. (2022). Generation of Distinct Differentially Culturable Forms of Burkholderia following Starvation at Low Temperature. Microbiol. Spectr. 10 (1), e0211021. doi: 10.1128/spectrum.02110-21
Avraham R. (2023). Untangling cellular host-pathogen encounters at infection bottlenecks. Infect. Immun. 91 (4), e0043822. doi: 10.1128/iai.00438-22
Aziz A., Currie B. J., Mayo M., Sarovich D. S., Price E. P. (2020). Comparative genomics confirms a rare melioidosis human-to-human transmission event and reveals incorrect phylogenomic reconstruction due to polyclonality. Microb. Genom. 6 (2), e000326. doi: 10.1099/mgen.0.000326
Bearss J. J., Hunter M., Dankmeyer J. L., Fritts K. A., Klimko C. P., Weaver C. H., et al. (2017). Characterization of pathogenesis of and immune response to Burkholderia pseudomallei K96243 using both inhalational and intraperitoneal infection models in BALB/c and C57BL/6 mice. PloS One 12, e0172627. doi: 10.1371/journal.pone.0172627
Biryukov S. S., Cote C. K., Klimko C. P., Dankmeyer J. L., Rill N. O., Shoe J. L., et al. (2022). Evaluation of two different vaccine platforms for immunization against melioidosis and glanders. Front. Microbiol. 13, 965518. doi: 10.3389/fmicb.2022.965518
Burtnick M. N., Shaffer T. L., Ross B. N., Muruato L. A., Sbrana E., DeShazer D., et al. (2018). Development of subunit vaccines that provide high-level protection and sterilizing immunity against acute inhalational melioidosis. Infect. Immun. 86 (1), e00724-17. doi: 10.1128/IAI.00724-17
Butt A., Higman V. A., Williams C., Crump M. P., Hemsley C. M., Harmer N., et al. (2014). The HicA toxin from Burkholderia pseudomallei has a role in persister cell formation. Biochem. J. 459, 333–344. doi: 10.1042/BJ20140073
CDC (2022). Melioidosis Locally Endemic in Areas of the Mississippi GulfCoast after Burkholderia pseudomallei Isolated in Soil and Water and Linked to Two Cases—Mississippi, 2020 and 2022. Distributed online via the CDC Health Alert Network July 27, 2022. CDCHAN-00470.
Cheng A. C., Currie B. J. (2005). Melioidosis: epidemiology, pathophysiology, and management. Clin. Microbiol. Rev. 18, 383–416. doi: 10.1128/CMR.18.2.383-416.2005
Cheng A. C., Dance D. A., Currie B. J. (2005). Bioterrorism, glanders and melioidosis. Euro Surveill 10, E1–E2. doi: 10.2807/esm.10.03.00528-en
Compton S. R., Homberger F. R., MacArthur Clark J. (2004). Microbiological monitoring in individually ventilated cage systems. Lab. Anim. (NY) 33, 36–41. doi: 10.1038/laban1104-36
Conejero L., Patel N., de Reynal M., Oberdorf S., Prior J., Felgner P. L., et al. (2011). Low-dose exposure of C57BL/6 mice to Burkholderia pseudomallei mimics chronic human melioidosis. Am. J. Pathol. 179, 270–280. doi: 10.1016/j.ajpath.2011.03.031
Cossaboom C. M., Marinova-Petkova A., Strysko J., Rodriguez G., Maness T., Ocampo J., et al. (2020). Melioidosis in a resident of texas with no recent travel history, United States. Emerg. Infect. Dis. 26 (6), 1295–1299. doi: 10.3201/eid2606.190975
Currie B. J. (2015). Melioidosis: evolving concepts in epidemiology, pathogenesis, and treatment. Semin. Respir. Crit. Care Med. 36, 111–125. doi: 10.1055/s-0034-1398389
Currie B. J., Meumann E. M., Kaestli M. (2023). The expanding global footprint of Burkholderia pseudomallei and melioidosis. Amer J. Trop. Med. Hyg. 108 (6), 1081–1083. doi: 10.4269/ajtmh.23-0223
Currie B. J., Ward L., Cheng A. C. (2010). The epidemiology and clinical spectrum of melioidosis: 540 cases from the 20 year Darwin prospective study. PloS Negl. Trop. Dis. 4, e900. doi: 10.1371/journal.pntd.0000900
Dance D. A., Limmathurotsakul D. (2018). Global burden and challenges of melioidosis. Trop. Med. Infect. Dis. Jan 29, 3. doi: 10.3390/tropicalmed3010013
Dawson P., Duwell M. M., Elrod M. G., Thompson R. J., Crum D. A., Jacobs R. M., et al. (2021). Human melioidosis caused by novel transmission of Burkholderia pseudomallei from freshwater home aquarium, United States. Emerg. Infect. Dis. 27, 3030–3035. doi: 10.3201/eid2712.211756
DPIRD (2018). Department of Primary Industries and Regional Development; Government of Western Australia, Agriculture and Food: Melioidosis in animals. Available at: https://www.agric.wa.gov.au/livestock-biosecurity/melioidosis-animals.
Finlay B. B., McFadden G. (2006). Anti-immunology: evasion of the host immune system by bacterial and viral pathogens. Cell 124, 767–782. doi: 10.1016/j.cell.2006.01.034
Funnell S. G. P., Tree J. A., Hatch G. J., Bate S. R., Hall G., Pearson G., et al. (2019). Dose-dependant acute or subacute disease caused by Burkholderia pseudomallei strain NCTC 13392 in a BALB/c aerosol model of infection. J. Appl. Microbiol. 127, 1224–1235. doi: 10.1111/jam.14396
Gassiep I., Grey V., Thean L. J., Farquhar D., Clark J. E., Ariotti L., et al. (2023). Expanding the geographic boundaries of melioidosis in queensland, Australia. Am. J. Trop. Med. Hyg 108, 1215–1219. doi: 10.4269/ajtmh.23-0002
Gee J. E., Bower W. A., Kunkel A., Petras J., Gettings J., Bye M., et al. (2022). Multistate outbreak of melioidosis associated with imported aromatherapy spray. N Engl. J. Med. 386, 861–868. doi: 10.1056/NEJMoa2116130
Guyton A. C. (1947a). Analysis of respiratory patterns in laboratory animals. Am. J. Physiol. 150, 78–83. doi: 10.1152/ajplegacy.1947.150.1.78
Guyton A. C. (1947b). Measurement of the respiratory volumes of laboratory animals. Am. J. Physiol. 150, 70–77. doi: 10.1152/ajplegacy.1947.150.1.70
Hall C. M., Stone N. E., Martz M., Hutton S. M., Santana-Propper E., Versluis L., et al. (2023). Burkholderia thailandensis isolated from the environment, United States. Emerg. Infect. Dis. 29, 618–621. doi: 10.3201/eid2903.221245
Kaewrakmuk J., Chusri S., Hortiwakul T., Kawila S., Patungkaro W., Jariyapradub B., et al. (2023). Under-reporting cases and deaths from melioidosis: A retrospective finding in songkhla and phatthalung province of southern Thailand, 2014-2020. Trop. Med. Infect. Dis. 8 (5), 286. doi: 10.3390/tropicalmed8050286
Kappel S., Hawkins P., Mendl M. T. (2017). To group or not to group? Good practice for housing male laboratory mice. Animals (Basel) 7 (12), 88. doi: 10.3390/ani7120088
Klimko C. P., Shoe J. L., Rill N. O., Hunter M., Dankmeyer J. L., Talyansky Y., et al. (2022). Layered and integrated medical countermeasures against Burkholderia pseudomallei infections in C57BL/6 mice. Front. Microbiol. 13, 965572. doi: 10.3389/fmicb.2022.965572
Lazar Adler N. R., Dean R. E., Saint R. J., Stevens M. P., Prior J. L., Atkins T. P., et al. (2013). Identification of a predicted trimeric autotransporter adhesin required for biofilm formation of Burkholderia pseudomallei. PLoS One 8 (11), e79461. doi: 10.1371/journal.pone.0079461
Leakey A. K., Ulett G. C., Hirst R. G. (1998). BALB/c and C57Bl/6 mice infected with virulent Burkholderia pseudomallei provide contrasting animal models for the acute and chronic forms of human melioidosis. Microb. Pathog. 24, 269–275. doi: 10.1006/mpat.1997.0179
Lever M. S., Nelson M., Stagg A. J., Beedham R. J., Simpson A. J. (2009). Experimental acute respiratory Burkholderia pseudomallei infection in BALB/c mice. Int. J. Exp. Pathol. 90, 16–25. doi: 10.1111/j.1365-2613.2008.00619.x
Limmathurotsakul D., Chaowagul W., Chierakul W., Stepniewska K., Maharjan B., Wuthiekanun V., et al. (2006). Risk factors for recurrent melioidosis in northeast Thailand. Clin. Infect. Dis. 43 (6), 979–986. doi: 10.1086/507632
Limmathurotsakul D., Funnell S. G., Torres A. G., Morici L. A., Brett P. J., Dunachie S., et al. (2015). Consensus on the development of vaccines against naturally acquired melioidosis. Emerg. Infect. Dis. 21 (6), e141480. doi: 10.3201/eid2106.141480
Limmathurotsakul D., Golding N., Dance D. A., Messina J. P., Pigott D. M., Moyes C. L., et al. (2016). Predicted global distribution of Burkholderia pseudomallei and burden of melioidosis. Nat. Microbiol. 1, 15008. doi: 10.1038/nmicrobiol.2015.8
Limmathurotsakul D., Peacock S. J. (2011). Melioidosis: a clinical overview. Br. Med. Bull. 99, 125–139. doi: 10.1093/bmb/ldr007
Massey S., Yeager L. A., Blumentritt C. A., Vijayakumar S., Sbrana E., Peterson J. W., et al. (2014). Comparative Burkholderia pseudomallei natural history virulence studies using an aerosol murine model of infection. Sci. Rep. 4, 4305. doi: 10.1038/srep04305
Nelson M., Barnes K. B., Davies C. H., Cote C. K., Meinig J. M., Biryukov S. S., et al. (2023). The BALB/c mouse model for the evaluation of therapies to treat infections with aerosolized Burkholderia pseudomallei. Antibiotics (Basel). Mar. 3, 12. doi: 10.3390/antibiotics12030506
Nelson M., Nunez A., Ngugi S. A., Atkins T. P. (2021). The lymphatic system as a potential mechanism of spread of melioidosis following ingestion of Burkholderia pseudomallei. PloS Negl. Trop. Dis. 15, e0009016. doi: 10.1371/journal.pntd.0009016
Nicklas W., Bleich A., Mahler M. (2012). Viral infections of laboratory mice. The Laboratory Mouse 2012, 427–480. doi: 10.1016/B978-0-12-382008-2.00019-2
Nithichanon A., Rinchai D., Buddhisa S., Saenmuang P., Kewcharoenwong C., Kessler B., et al. (2018). Immune control of Burkholderia pseudomallei–common, high-frequency T-cell responses to a broad repertoire of immunoprevalent epitopes. Front. Immunol. 9, 484. doi: 10.3389/fimmu.2018.00484
Pumpuang A., Chantratita N., Wikraiphat C., Saiprom N., Day N. P., Peacock S. J., et al. (2011). Survival of Burkholderia pseudomallei in distilled water for 16 years. Trans. R Soc. Trop. Med. Hyg 105, 598–600. doi: 10.1016/j.trstmh.2011.06.004
Rees E. M., Minter A., Edmunds W. J., Lau C. L., Kucharski A. J., Lowe R. (2021). Transmission modelling of environmentally persistent zoonotic diseases: a systematic review. Lancet Planet Health 5, e466–e478. doi: 10.1016/S2542-5196(21)00137-6
Sanchez-Villamil J. I., Tapia D., Borlee G. I., Borlee B. R., Walker D. H., Torres A. G. (2020). Burkholderia pseudomallei as an enteric pathogen: identification of virulence factors mediating gastrointestinal infection. Infect. Immun. 15, 89. doi: 10.1128/IAI.00654-20
Seng R., Phunpang R., Saiprom N., Dulsuk A., Chewapreecha C., Thaipadungpanit J., et al. (2023). Phenotypic and genetic alterations of Burkholderia pseudomallei in patients during relapse and persistent infections. Front. Microbiol. 14, 1103297. doi: 10.3389/fmicb.2023.1103297
Shams A. M., Rose L. J., Hodges L., Arduino M. J. (2007). Survival of Burkholderia pseudomallei on environmental surfaces. Appl. Environ. Microbiol. 73, 8001–8004. doi: 10.1128/AEM.00936-07
Srisurat N., Sermswan R. W., Tatawasart U., Wongratanacheewin S. (2010). Bacterial loads and antibody responses in BALB/c mice infected with low and high doses of Burkholderia pseudomallei. Am. J. Trop. Med. Hyg. 82 (6), 1102–1105. doi: 10.4269/ajtmh.2010.09-0567
Stockton J. L., Torres A. G. (2020). Multinucleated giant cell formation as a portal to chronic bacterial infections. Microorganisms 8 (11), 1637. doi: 10.3390/microorganisms8111637
Tan G. G., Liu Y., Sivalingam S. P., Sim S. H., Wang D., Paucod J. C., et al. (2008). Burkholderia pseudomallei aerosol infection results in differential inflammatory responses in BALB/c and C57Bl/6 mice. J. Med. Microbiol. 57 (Pt 4), 508–515. doi: 10.1099/jmm.0.47596-0
Titball R. W., Burtnick M. N., Bancroft G. J., Brett P. (2017). Burkholderia pseudomallei and Burkholderia mallei vaccines: Are we close to clinical trials? Vaccine 35 (44), 5981–5989. doi: 10.1016/j.vaccine.2017.03.022
Trevino S. R., Dankmeyer J. L., Fetterer D. P., Klimko C. P., Raymond J. L. W., Moreau A. M., et al. (2021). Comparative virulence of three different strains of Burkholderia pseudomallei in an aerosol non-human primate model. PloS Negl. Trop. Dis. 15 (2), e0009125. doi: 10.1371/journal.pntd.0009125
Trevino S. R., Klimko C. P., Reed M. C., Aponte-Cuadrado M. J., Hunter M., Shoe J. L., et al. (2018). Disease progression in mice exposed to low-doses of aerosolized clinical isolates of Burkholderia pseudomallei. PloS One 13, e0208277. doi: 10.1371/journal.pone.0208277
Valvano M. A., Keith K. E., Cardona S. T. (2005). Survival and persistence of opportunistic Burkholderia species in host cells. Curr. Opin. Microbiol. 8, 99–105. doi: 10.1016/j.mib.2004.12.002
Velapatino B., Limmathurotsakul D., Peacock S. J., Speert D. P. (2012). Identification of differentially expressed proteins from Burkholderia pseudomallei isolated during primary and relapsing melioidosis. Microbes Infect. 14, 335–340. doi: 10.1016/j.micinf.2011.11.011
Welkos S. L., Klimko C. P., Kern S. J., Bearss J. J., Bozue J. A., Bernhards R. C., et al. (2015). Characterization of Burkholderia pseudomallei strains using a murine intraperitoneal infection model and in vitro macrophage assays. PloS One 10, e0124667. doi: 10.1371/journal.pone.0124667
Wiersinga W. J., Virk H. S., Torres A. G., Currie B. J., Peacock S. J., Dance D. A. B., et al. (2018). Melioidosis. Nat. Rev. Dis. Primers 4, 17107. doi: 10.1038/nrdp.2017.107
Keywords: melioidosis, Burkholderia pseudomallei, mice, inhalational, intraperitoneal, transmission, reinfection
Citation: Klimko CP, Barnes KB, Rill NO, Shoe JL, Dankmeyer JL, Hunter M, Welkos SL, DeShazer D, Biryukov SS, Harding SV and Cote CK (2023) Detection of low-level animal-to-animal transmission in BALB/c mouse models of melioidosis. Front. Bacteriol. 2:1303051. doi: 10.3389/fbrio.2023.1303051
Received: 27 September 2023; Accepted: 17 October 2023;
Published: 06 November 2023.
Edited by:
Myron Christodoulides, University of Southampton, United KingdomReviewed by:
David Allan Brett Dance, Retired, Buckland Monachorum, Devon, United KingdomCopyright © 2023 Klimko, Barnes, Rill, Shoe, Dankmeyer, Hunter, Welkos, DeShazer, Biryukov, Harding and Cote. This is an open-access article distributed under the terms of the Creative Commons Attribution License (CC BY). The use, distribution or reproduction in other forums is permitted, provided the original author(s) and the copyright owner(s) are credited and that the original publication in this journal is cited, in accordance with accepted academic practice. No use, distribution or reproduction is permitted which does not comply with these terms.
*Correspondence: Christopher K. Cote, Y2hyaXN0b3BoZXIuay5jb3RlLmNpdkBoZWFsdGgubWls
Disclaimer: All claims expressed in this article are solely those of the authors and do not necessarily represent those of their affiliated organizations, or those of the publisher, the editors and the reviewers. Any product that may be evaluated in this article or claim that may be made by its manufacturer is not guaranteed or endorsed by the publisher.
Research integrity at Frontiers
Learn more about the work of our research integrity team to safeguard the quality of each article we publish.