- 1Department of Astronomy, Faculty of Mathematics, University of Belgrade, Belgrade, Serbia
- 2Physics and Astronomy, University Kent, Canterbury, United Kingdom
- 3Data Science, Simulation and Learning Division, Fermi National Accelerator Laboratory, Batavia, IL, United States
- 4Department of Astronomy and Astrophysics, University of Chicago, Chicago, IL, United States
- 5NSF-Simons AI Institute for the Sky (SkAI), Chicago, IL, United States
The Vera C. Rubin Observatory’s Legacy Survey of Space and Time (LSST) presents an unprecedented opportunity to advance the search for astrobiologically relevant data across diverse astronomical environments. Through its extensive imaging capabilities, LSST will enhance our inventory of Solar System objects and assess their potential astrobiological conditions. Beyond the Solar System, LSST will survey billions of stars, contributing to the detection of exoplanets, characterizing planetary atmospheres at a zeroth-order level, identifying transient phenomena, and exploring conditions within habitable zones. In this paper, we highlight LSST’s unique contribution to astrobiology, complementing other missions and expanding the search for life beyond Earth in the coming decades.
1 Introduction
The Vera C. Rubin Observatory, formerly the Large Synoptic Survey Telescope, located at Cerro Pachón in Chile, is one of the next class of giant earth-based telescopes (left plot in Figure 1). It will house the world’s largest digital camera ever fabricated for optical astronomy which will be used to collect hundreds of images of the Southern Hemisphere sky (right plot in Figure 1), collecting 20 TB of data every night, for 10 years, to produce a synoptic astronomical survey, the ‘Legacy Survey of Space and Time’ (Ivezić et al., 2019; Bianco et al., 2021). Its data will provide hitherto unseen details of our Universe. On a cosmological scale, it will provide the most detailed map of the distribution of dark matter in space and, hence, provide a measurement of the acceleration of the expansion of the universe, providing a stringent test of modern cosmology.
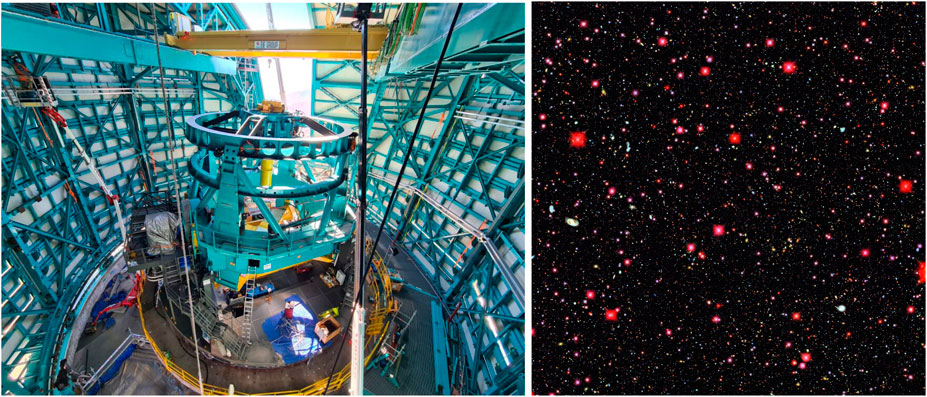
Figure 1. Left: LSST 3.2-Gpx digital Camera and Simonyi Survey Telescope. The telescope’s three-mirror design (8.4 m, 5 m, and 3.4 m) enables rapid repointing within
In parallel, the Vera C. Rubin Observatory will also be at the forefront of planetary science for the next decade. In our Solar system, LSST will be capable of detecting 90% of all near-Earth objects—asteroids with diameters
In this paper we review LSST’s transformative role in astrobiology, focusing on its technological capabilities, its multidata synergies, and interdisciplinary applications. Section 2 explores LSST’s technological features for multiscale astrobiology across time, energy, and chemistry. Section 3 provides examples of astrobiological studies with LSST. Section 4 positions LSST role in multimodal data and multimessenger astrobiology. Section 5 overviews LSST as a platform for interdsiciplinary collaboration. Finally, Section 6 concludes with short proposition of community organization.
2 LSST and multiscale astrobiology
A planet’s location in the habitable zone alone does not confirm its habitability (Kaltenegger, 2017). Assessing habitability requires detailed atmospheric and surface observations, typically through spectroscopy. Advanced facilities like the James Webb Space Telescope excel in this domain (Bello-Arufe et al., 2022; Pham and Kaltenegger, 2021), but spectroscopy is resource-intensive (Pham and Kaltenegger, 2021). Photometry, by contrast, provides a faster method to classify and prioritize exoplanet candidates. Studies show that photometric colors effectively distinguish surface types (e.g., icy, rocky, or gaseous) and can indicate biosignatures [see Pham and Kaltenegger (2021)]. LSST photometry provides a rapid and complementary approach to detecting astrobiological signals by using temporal, spectral, and chemical composition variations in images and light curves.
On the temporal scale, LSST’s frequent observations (with an average cadence of
On the scale of the spectrum, LSST’s six broadband filters—u (304.3–404.5 nm), g (385.6–566.3 nm), r (533.7–705.7 nm), i (669.9–837.8 nm), z (799.3–939.2 nm), and y (907.5–1,100 nm)3—approximate zeroth-order spectroscopy (Figure 2). Color indices, defined as magnitude differences between filters (e.g., g-r), can reveal stellar properties such as temperature and luminosity, which are critical for determining planetary habitability (Kopparapu et al., 2013). For example, cooler stars shift habitable zones inward, allowing conditions suitable for liquid water.
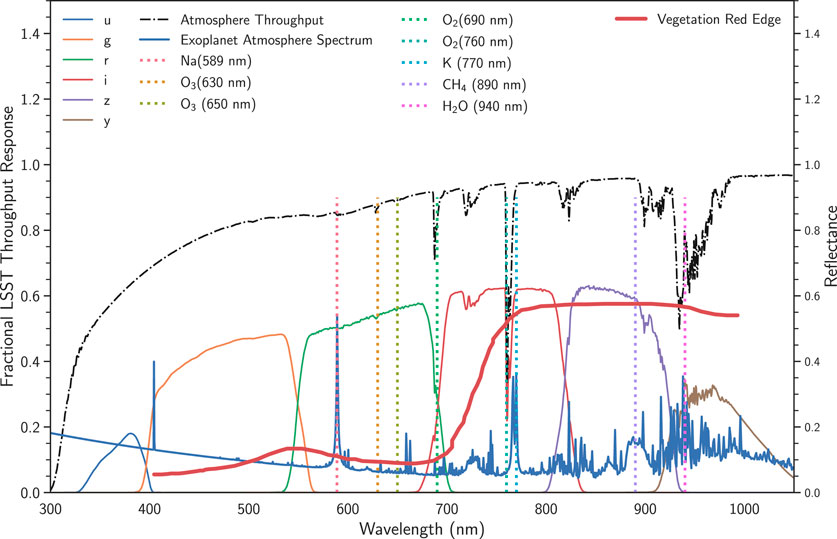
Figure 2. The LSST throughput curves across the ugrizy bands (colored lines) represent the combined effects of the Rubin Observatory system4, including mirrors, lenses, filters, detectors, and a fiducial atmosphere at 1.2 airmasses (black dash-dotted line). Overlaid is a scaled spectrum of a
On the scale of chemical composition, LSST can detect biosignatures by analyzing variations in atmospheric and surface features through broadband photometry. Magnitude differences between filters, such as g-r, may reveal molecular absorption features indicative of key compounds like ozone (
Additionally, molecular absorption by
With a wide field of view (3.5
3 Examples of astrobiological studies with LSST
3.1 Solar system
3.1.1 Detection of near-earth objects (NEOs) and potentially hazardous asteroids (PHAs)
Objects that are ejected from the main asteroid belt that can enter Earth-crossing orbits [see Jones et al. (2018)] are known as Near-Earth Objects (NEOs), including asteroids and comets with perihelion distances of
Using its baseline survey strategy, LSST will detect 66% of PHAs and 61% of NEOs with
NEOs are also of major astrobiology interest due to their potential to carry water, organics, and other life-forming materials. For example, the Apollo asteroid (101955) Bennu, a 500 m NEO, was targeted by the OSIRIS-REx (Origins, Spectral Interpretation, Resource Identification, and Security–Regolith Explorer) mission, returning regolith samples containing carbon-rich dust, organic compounds, and clay minerals such as serpentine (see Lauretta et al., 2024, and references therein). Bennu’s composition suggests it may have originated from an ancient ocean world, with discoveries of magnesium-sodium phosphate resembling compounds found on Enceladus and in Earth’s soda lakes (Lauretta et al., 2024). Understanding the distribution of NEOs and PHAs is crucial for assessing their role in delivering prebiotic materials to Earth and other planets.
3.1.2 Characterization of comets and Kuiper Belt objects
Recent observations from the Subaru Telescope suggest that the apparent small size of the Kuiper Belt compared to other planetary systems may be the result of observational bias (Ivezić et al., 2019; Buie et al., 2024). A distinct cluster of objects appears to orbit in a 70–90 AU ring-like region, separated from the Kuiper Belt by a sparsely populated “valley” between 55 and 70 AU (Ohashi et al., 2023). Confirming this distant KBO region would imply structural similarities between the Solar and other planetary systems.
With its extensive sky coverage and depth, LSST is well-positioned to detect fainter, more distant KBOs, improving our understanding of the Solar System’s outer structure. LSST DP0.3 contains classical KBOs clustering at low eccentricities
For KBO motion tracking along a reference axis, such as the ecliptic plane, the observed velocity component
where the
For TNOs moving below 10 arcsec/h and
Expanding KBO detections with LSST has astrobiological implications. Identifying a diverse KBO population may suggest that the Solar System shares structural features with systems capable of hosting life. Our Solar system KBO long appeared to be very small relatively to other systems (e.g., Nilsson et al., 2010). If an extended KBO region
LSST’s observations could detect collision clouds from recent impacts (Stern, 1996), which evolve on timescales of days to weeks, and enable systematic studies of small body nuclei before and after active events. These observations would improve our understanding of outgassing, space weathering, and the interiors of small objects (Collaboration et al., 2021). LSST may also detect cryovolcanic activity or resurfacing events on KBOs (Glein et al., 2024), revealing chemical reactions relevant to astrobiology. For example, a large cryovolcanic eruption could produce a temporary coma or atmosphere, increasing brightness and altering surface characteristics detectable by LSST. LSST’s long-term monitoring is ideal for the revealing gradual changes in brightness or color due to cumulative cryovolcanic activity or seasonal variations.
Comets and KBOs serve as time capsules from the early Solar System, preserving conditions that shaped planetary formation. Comparative studies of their compositions and activities will refine models of Solar System evolution and highlight why some regions retained complex organics while others experienced greater geologic activity.
The Oort Cloud, extending up to halfway between the Sun and Alpha Centauri6, might exchange cometary material with Alpha Centauri’s cometary cloud. This could be explored as a potential pathway for “slow boat” interstellar probes taking millennia to traverse, relying on physics consistent with current technology (see Gilster, 2013).
3.2 Exoplanets
The LSST will allocate 90% of its observational time to regular cadence monitoring, with the remaining
Ozone exhibits an absorption feature in the visible spectrum known as the Chappuis bands, occurring between 400–600 nm. LSST’s g and r bands partially cover this range. Although LSST lacks the spectral resolution to unambiguously identify ozone, variations in the SED inferred from LSST data that suggest an ozone feature could still be discerned, particularly when combined with additional data from JWST or other instruments. Among the prominent atmospheric features detectable in exoplanet atmospheres by both ground- and space-based facilities is Rayleigh scattering (Murgas et al., 2020). Rayleigh scattering, caused by molecules such as nitrogen or oxygen in an exoplanet’s atmosphere, enhances the blue end of the visible spectrum. The scattering at 400 nm is approximately 9.4 times stronger than at 700 nm. LSST’s u and g bands (320–550 nm) are particularly sensitive to this effect. An SED showing a Rayleigh scattering slope could indicate the presence of an atmosphere with significant amounts of these molecules, which are associated with life on Earth. Moreover, in hydrogen-dominated planetary atmospheres, Rayleigh scattering causes the measured planetary radius to increase toward blue wavelengths in the optical range (Murgas et al., 2020).
3.2.1 The role of dust in planetary climate and atmosphere development
The inner Solar System contains a diffuse zodiacal dust cloud formed by grains released by comets and asteroids as they pass through the Solar System. These particles spiral toward the Sun over millions of years due to the relativistic Poynting-Robertson drag (Borin et al., 2017), occupying the inner few astronomical units (AU). Numerical simulations of the orbital evolution of asteroidal dust particles have predicted two dense dust clumps—one leading and one trailing Earth, with the trailing clump being the denser of the two (Stark et al., 2013)—first observed by the Cosmic Background Explorer (COBE; Reach et al., 1995). On the other hand, KBOs are believed to be the dominant source of interplanetary dust particles in the outer solar system due to KBOs twofold collisons: mutual and with interstellar dust particles. New Horizons measurements through 55 AU show higher than predicted dust fluxes which remains unexplained but may involve KBOs (Doner et al., 2024).
The LSST will produce multi-color ugrizy images covering half the sky, with a depth of
Only recently has the structure of dust populations around other stars, known as exozodiacal disks, become studied via spectral line observations. However, missions like Kepler (Arkhypov et al., 2019) been studied dust detection across larger samples of objects through broadband photometry. For example, dust has been observed in rocky exoplanet tails (Garai, 2018) and at altitudes of
Exozodiacal light (exoZL), a faint, extended glow around stars, is 100–1,000 times fainter than Solar System ZL. LSST’s angular resolution (0.7 arcsec) may detect exoZL around nearby stars, but separating it from brighter starlight and background noise remains challenging. Models by Stark (2011) show that resonant dust clumps formed by planetary trapping produce transit-like light curve minima. Jupiter-mass planets generate the strongest signals (amplitudes
The Nancy Grace Roman Space Telescope, with its Coronagraph Instrument at Lagrange Point 2, will surpass LSST in sensitivity to warm exozodiacal dust in habitable zones (Douglas et al., 2022), particularly for rocky grains closer to stars (Ertel et al., 2020). Understanding exozodiacal dust chemistry offers unique insights into planetary formation and guides future missions to image habitable-zone planets.
Dust surges into Earth’s atmosphere have been proposed to influence climate, potentially causing cooling events or contributing to glacial cycles (e.g., Muller and MacDonald, 1997). Milankovitch cycles of order
3.3 Interstellar medium (ISM)
3.3.1 The ISM and prebiotic chemistry
The ISM, composed of gas, dust, and organic molecules, acts as both a repository and transit region for elements essential to life (Herbst, 2021). Planet formation in protoplanetary discs, which occurs over a few million years, may depend on late-stage gas infall from the ISM (Winter et al., 2024), potentially critical for forming life-bearing planets. The ISM is highly heterogeneous, with extreme conditions (Puzzarini and Alessandrini, 2024): temperatures from 10 to 200 K, densities of
LSST’s Galactic science program will map ISM dust across the Milky Way and neighboring galaxies using five-color spectral energy distributions (SEDs) of main-sequence stars (see page 35 in Ivezić et al., 2019). This mapping will reveal variations in dust properties and their implications for habitability. LSST’s multi-band photometry will also enable reddening
Detailed ISM maps will provide critical insights into regions favorable for prebiotic hotspots. Variations in grain size, metallicity, density, and radiation fields will help pinpoint areas with optimal conditions for complex molecule synthesis. LSST will highlight regions with protective dust shielding or metal-rich environments conducive to life-forming processes. Another key area is active galactic nuclei (AGN) feedback and its influence on the ISM and exoplanets (Balbi and Tombesi, 2017). AGN-driven outflows and radiation may either hinder or enhance organic chemistry by altering molecular stability and dust shielding. For instance, recent observations of the Sgr
3.4 Star and planet formation
3.4.1 Monitoring stellar activity and flaring stars
Stellar flares, transient events caused by magnetic reconnection in the stellar photospheres, are particularly common on M dwarfs. These events serve as indicators of stellar magnetic activity, correlating with age and rotation rates (Davenport, 2016). Flares have astrobiological implications, as their intense UV flux can deplete planetary ozone layers or influence prebiotic chemistry, acting as both triggers and inhibitors of life (Ramsay et al., 2021).
Flares are challenging to capture in synoptic surveys like LSST due to their short-lived nature, often yielding only single-point detections. To address this, Clarke et al. (2024) have developed a method leveraging Differential Chromatic Refraction (DCR) to estimate flare temperatures from single-epoch detections. By modeling the DCR effect as a function of atmospheric column density, photometric filter, and flare temperature, they showed that flare temperatures
Complementary methods can enhance LSST’s ability to study flares. Time-domain segmentation in LSST’s alert stream can trigger follow-up observations by fast-response telescopes, capturing multi-band data to constrain flare properties. Machine learning (ML) models trained on LSST data could identify flare candidates from single exposures. Stacking and probabilistic modeling of single-visit observations can reconstruct flare temperature and energy distributions, enabling statistical analyses of occurrence rates and properties.
Cross-matching LSST data with Gaia and TESS would also add value; TESS provides continuous light curves, while Gaia offers precise astrometry for multi-wavelength studies. A synthetic reference star framework in LSST images could further enable real-time analysis of flare-induced DCR offsets, revealing subtle astrometric shifts and temperature changes over time. These methods will refine flare emission models and clarify relationships between flare parameters (temperature, duration, energy) and stellar characteristics (spectral type, rotation, magnetic field strength). A catalog of flare temperatures will also guide candidate selection for biosignature surveys, given the atmospheric impact of intense flares (Clarke et al., 2023; Clarke et al., 2024).
3.4.2 Understanding the distribution of life-forming elements
The metallicity of a host star, particularly its iron content (Fe/H), correlates with the likelihood of planetary system formation, serving as a proxy for other heavy elements essential for planet formation and organic chemistry, such as C, N, O, and Si (Covone and Giovannelli, 2022). Phosphorus is also essential, as its scarcity in a star may result in planets that are unsuitable for life (Hinkel et al., 2020).
LSST’s photometric metallicity measurements will provide an unprecedented dataset of approximately 200 million main-sequence F/G stars, extending to 100 kpc in the Galactic halo (see Ivezić et al., 2019, and references therein). LSST’s observations will surpass Gaia, which is flux-limited at
From an astrobiological perspective, LSST’s stellar metallicity insights will aid in understanding conditions for planet formation and habitability. Probing metallicity patterns in the halo will identify regions enriched or depleted in heavy elements, informing planetary formation potential in diverse Galactic environments. LSST’s trigonometric parallax measurements for low-mass stars near the hydrogen-burning limit will map stellar populations driving chemical enrichment, contributing to models of Galactic habitability. Additionally, LSST’s measurements of the white dwarf luminosity function will provide independent age estimates for the Galactic disk and halo, identifying ancient, stable systems with long-term habitability potential.
Cross-matching LSST’s stellar metallicity catalog with spectroscopic surveys like 4MOST (de Jong et al., 2019) will refine biosignature searches by identifying regions rich in life-supporting elements. LSST’s combined stellar and exoplanet datasets will enable Bayesian modeling to evaluate the likelihood of life under different metallicity thresholds and atmospheric conditions. By linking metallicity data with known atmospheric biosignatures, LSST can improve Bayesian posterior probabilities for the likelihood of life-supporting environments.
Synergies with spectroscopic studies, such as the Apache Point Observatory Galactic Evolution Experiment (APOGEE, Majewski et al., 2017), demonstrate the importance of mapping life-supporting elements. APOGEE has detected higher concentrations of heavy elements in the inner Galaxy, where stars are older, suggesting that essential life elements were synthesized earlier in the inner regions compared to the outer (Albareti et al., 2017).
3.5 Mapping the galactic habitable zone
Galactic habitability examines how large-scale environmental factors within a galaxy influence the abundance of planets conducive to life (see e.g., Dayal et al., 2015; Lacki, 2021). Key factors include (1) stellar population metallicity, which affects planet formation frequency; (2) high-energy events from massive stars, potentially driving mass extinctions; and (3) frequent stellar encounters in dense regions, which may destabilize planetary systems.
Analysis by Dayal et al. (2015), based on SDSS data, revealed that over 100,000 local galaxies lie on a remarkably thin plane in a 3D space defined by these parameters. The Milky Way, classified as “typically average,” exemplifies this trend. Larger galaxies, particularly giant ellipticals lacking spiral structures, are more metal-rich and exhibit lower supernova rates, making them strong candidates for habitability. Remarkably, a giant elliptical at least twice the size of the Milky Way could support up to 10,000 times more habitable Earth-like planets due to its reduced high-energy activity and enriched metallicity.
The LSST will expand on SDSS’s insights by observing billions of galaxies. Its deep, multi-band photometry will enable mapping of stellar populations and metal-rich regions, while high-cadence imaging will track supernovae, refining galactic habitability models by identifying low-radiation environments. LSST’s photometric focus necessitates alternative methods to calculate star formation rates (SFRs) without direct spectroscopic data. UV-optical colors (e.g., u - g or u - r) serve as proxies for recent star formation, with calibrations available for estimating SFRs through empirical or ML models trained on datasets with known SFRs.
Programs like CIGALE (Code Investigating Galaxy Emission, Boquien et al., 2019) and LePhare (Photometric Analysis for Redshift Estimates, Arnouts and Ilbert, 2011) are designed for spectral energy distribution fitting (Yang et al., 2020) and can estimate SFRs from photometry by modeling the contributions of young stars and dust attenuation8. ML models trained on galaxies with spectroscopic data from surveys like SDSS, DESI (Dark Energy Spectroscopic Instrument), or future follow-ups (e.g., Wide Spectroscopic Telescope-WST, 4MOST) can directly map LSST photometry to known SFRs. LSST’s overlap with these surveys will enable robust calibrations, leveraging color, magnitude, and redshift to predict SFRs and extend our understanding of galactic habitability across diverse environments.
3.6 Biosignatures and technosignatures searches
3.6.1 Biosignatures
The search for life focuses on two primary goals (Saha et al., 2018): identifying planets with Earth-like conditions (Earth similarity) and exploring the broader potential for life (habitability). Beyond paired atmospheric biosignatures such as
On Earth, the VRE is detectable as a global increase in the reflectance spectrum, with typical values around 50% for present-day vegetation (O’Malley-James and Kaltenegger, 2018), covering approximately 60% of the land surface. However, the VRE is not unique to modern plants, which only became dominant
In addition to photosynthesis, other forms of life, including microorganisms and extremophiles, could significantly influence a planet’s reflectance spectrum if they are widespread. Recent efforts, such as the development of spectral libraries for diverse microorganisms (Hegde et al., 2015), provide valuable data for interpreting these potential biosignatures. These libraries include reflectance measurements from the visible to near-infrared spectrum, offering a crucial tool for identifying and characterizing surface biosignatures on exoplanets9.
The VRE could potentially be detected through the color index derived from LSST transit light curves. If an exoplanet’s surface exhibits a VRE, a distinct increase in the
The distribution of stars
3.6.2 Technosignatures searches
Fast-moving objects (FMOs) in the LSST DP0.3 catalogue are characterized by high orbital eccentricity, small perihelion distances, and, in some cases, high inclinations (see Supplementary Figure S4). These result in rapid motion and increased brightness at perihelion distances, and swift positional changes across survey images. These features make FMOs briefly detectable but challenging to track, requiring high-cadence observations and advanced algorithms. For instance, an FMO moving at
LSST’s high-precision astrometry can detect deviations in Solar System object orbits that suggest artificial manipulation, potentially identifying free-floating probes, surface-based constructs, or artificial satellites (NASA Technosignatures Workshop Participants, 2018). Searches for small bodies may inherently uncover anomalies via unusual orbits, colors, or light curves. Furthermore, technosignatures may involve spatial or temporal coordination, such as synchronized transiting systems or rebroadcasted natural astrophysical events (e.g., novae), which could signal extraterrestrial communication (Davenport, 2019). LSST’s wide-field observations could also reveal phenomena like long-term stellar dimming or disappearing stars, indicative of artificial structures like Dyson spheres (see Suazo et al., 2024; Villarroel et al., 2022, and references therein).
A key challenge in technosignature searches is avoiding anthropocentric assumptions. Patterns like prime numbers or Fibonacci sequences reflect human biases toward mathematical relevance (Wigner, 1995). While mathematics may appear universal (Tegmark, 2008), it is argued to be shaped by human cognition (Lakoff and nez, 2000). Broader frameworks that incorporate non-traditional communication or structural forms are crucial (Döbler, 2020). To address possibility of non-traditional SETI signatures in the optical domain, recent studies by Megias Homar et al. (2023) highlight LSST’s ability to detect millisecond Fast Optical Bursts (FOBs), which may represent technosignatures or unknown phenomena. These bursts, distinct from steady sources, produce anomalous spatial patterns. Simulations with neural network classifiers show LSST can isolate FOBs, mapping their duration-intensity space and predicting significant detections over its 10-year survey (Megias Homar et al., 2023). Complementary efforts like PANOSETI (Wright et al., 2019) will expand the search by targeting nanosecond laser pulses in optical and near-infrared spectra, reducing reliance on human-centered assumptions.
4 LSST’s role in multimodal and multimessenger astrobiology
Over the past century, astronomical data have been primarily based on 2D images and 1D spectra or time series. Modern instruments like LSST are shifting this paradigm toward more complex, multi-dimensional data. LSST’s multi-epoch survey will generate time-image cubes, capturing dynamic phenomena across time, similar to video sequences. Additionally, photometric light curves from multiple filters can be stacked to form data cubes that combine both temporal and spectral information. This high-dimensional dataset is central to LSST’s role as an explorer of the ‘Cosmic Haystack’ (Tarter, 1985), enabling a systematic search for weak or infrequent signals, which may reveal astrobiological markers, technosignatures, or other anomalous phenomena.
The rise of multimessenger astronomy facilitates the integration of data from multiple observatories, such as LSST optical data, gravitational waves (GW), and neutrinos. Unlike radio surveys, which are limited to scanning a narrow spectral window at a given time, LSST’s wide-field optical monitoring offers a systematic, time-resolved approach to detect potential artificial sources (Liu et al., 2020). By complementing GW and neutrino observations, LSST supports multi-messenger strategies that narrow the search space for astrophysical anomalies possibly linked to extraterrestrial intelligence (Sellers et al., 2022). For example, GW from rapidly accelerating massive objects, potentially interpreted as extraterrestrial spacecraft, might coincide with LSST’s detection of unusual light curves and neutrino bursts that suggest advanced technology. Another scenario could involve GW from interacting massive objects, with LSST transits revealing atmospheric biosignatures and neutrino patterns indicating high-energy processes. Combining these observations offers a more robust framework for detecting extraterrestrial activity.
Multimessenger astronomy is essential for SETI, as it reduces false positives and strengthens signal detection through cross-validation (see also Wright, 2018). The number of technosignatures could far exceed biosignatures (Wright et al., 2022), highlighting the need for such integrated strategies. However, the vast space-time scales involved present significant challenges, requiring advanced methods for cross-validation, simulations, and theoretical analysis (Allen et al., 2019).
The spectral overlap between LSST and JWST (600–28,500 nm) facilitates multi-wavelength studies. In the 600–1,050 nm range, LSST’s optical bands overlap with JWST, covering the r, i, z, and y bands10. This overlap enables a comprehensive characterization of exoplanetary atmospheres by combining JWST’s infrared spectroscopy with LSST’s time-domain photometry (Greene et al., 2016). Joint observations allow for the extraction of crucial atmospheric parameters, such as molecular absorption features, cloud properties, and thermal variations (Madhusudhan, 2019).
4.1 ML prospects for multimessenger technosignature detection
To efficiently analyze these multimodal datasets, the Structured Data Fusion (SDF) framework offers a powerful method for integrating diverse astrophysical observations. SDF represents datasets as high-dimensional tensors and applies decomposition techniques to identify shared structures across different types of data (Lahat et al., 2015). This approach has already been applied in multimodal astrophysical analyses, such as combining photometry, spectroscopy, and GW data to extract underlying physical processes (Cuoco et al., 2022). Importantly, the astronomical community has rising awarness of the worth of a multimodal approach. For example, the ‘Multimodal Universe’ collaboration capitalizes on observations available from a diversity of ground- and space-based telescopes forming a dataset of hundreds of millions of astrophysical objects and phenomena. Altogether, this comrises
Recent development in quantum computing, deployed on platforms like Google Quantum AI (Arute et al., 2019) and IBM Quantum Services12, offers the potential for adavancing multimodal data analysis. Despite ongoing debates about quantum advantage (e.g., Renaud et al., 2024), its integration into scientific pipelines for medical research is gaining momentum (Li et al., 2024). For instance, in astronomy hybrid quantum-classical models could help detect technosignatures by identifying anomalous periodic light curves and even artificial gravitational wave signals (Sellers et al., 2022). These models can encode latent representations using parameterized quantum circuits and decode them through classical neural networks, which show promise in reconstructing weak signals from noise (Kovačević et al., 2024).
4.2 LSST’s follow up of GW events–kilonovae
Neutron star mergers generate kilonovae—high-energy explosions that synthesize and disperse heavy elements, including iodine and gold, which are essential for biochemistry and advanced technologies (Wehmeyer et al., 2023; Perkins et al., 2024). While GW detections from these events provide critical insights into merger dynamics, it is LSST’s optical follow-up that constrains ejecta properties, tracking how these elements propagate through interstellar space. Over LSST’s 10-year survey, up to three Galactic kilonovae are expected (Perkins et al., 2024), with JWST’s infrared spectroscopy offering complementary data on r-process nucleosynthesis (Levan et al., 2024).
Recent observations of GRB 230307A, a kilonova similar to GW170817, confirmed the astrophysical production of iodine, highlighting the role of neutron star mergers in distributing biologically significant elements (Crockford, 2009). LSST is projected to detect more than 300 kilonovae within 1,400 Mpc, with 1%–10% classified as fast-evolving transients (Andreoni et al., 2021). Strategies for identifying these events include all-sky surveys and targeted follow-up of GW alerts from LIGO/Virgo (Ragosta et al., 2024).
By combining LSST light curves with JWST spectra, researchers can assess superheavy-element production in kilonova ejecta (Holmbeck et al., 2023). Notably, JWST has already detected tellurium—an r-process element relevant to life—suggesting that iodine and other biologically significant elements may also be present13 (Levan et al., 2024). These elements, crucial for materials such as magnets and lasers (see Supplementary Figure S5), could influence planetary habitability and even the emergence of technologically advanced societies (Zhang et al., 2023). A Type III civilization, capable of harnessing a galaxy’s energy, might rely on such elements for infrastructure and computational technologies (Zhang et al., 2023). Mapping their interstellar distribution with LSST and JWST will improve our understanding of both astrobiology and the conditions necessary for advanced technological evolution.
4.3 Neutrino event follow-up with LSST
Neutrinos have been proposed as a potential medium for interstellar communication, given their ability to propagate over cosmological distances with minimal attenuation (Pasachoff and Kutner, 1979). Experimental demonstrations have shown the feasibility of neutrino-based communication over short distances, such as the 1.035 km low-rate neutrino link established using the NuMI beam line and MINERvA detector (Stancil et al., 2012). In astrophysical contexts, brief, targeted signals synchronized with transient events, such as supernovae or gamma-ray bursts (GRBs), have been suggested as Schelling points that could enhance signal detectability (Nishino and Seto, 2018). Learned et al. (2009) demonstrated that 6.3 PeV electron–anti-neutrino beams, undetectable from natural sources, could be used as extraterrestrial intelligence (ETI) signals if synchronized with such astrophysical events. Detecting such rare signals would require continuous monitoring by neutrino observatories and systematic cross-matching with transient detections.
The LSST survey is expected to detect millions of transient events, including supernovae, over its 10-year operational period (Gris et al., 2023). While supernovae generally exhibit slow-evolving light curves, they could function as Schelling points for synchronized ETI communication, particularly if accompanied by neutrino bursts (Learned et al., 2009). For example, the GW170817 neutron star merger produced unexpected neutrino signals (Fischbach et al., 2018), prompting discussions on possible non-astrophysical origins (Santos et al., 2020). Cross-matching LSST’s transient detections with GW and neutrino data could help identify regions of interest (ROIs) for further investigation.
LSST’s Field of View (FoV) is defined as
5 LSST as a platform for interdisciplinary collaboration
5.1 Bridging physics and astrobiology
A new and compelling connection between physics and astrobiology emerges from the study of dark matter candidates, such as ultralight bosons, and their interactions with supermassive black holes (SMBHs), altering their spin distributions and influencing phenomena like tidal disruption events (TDEs). Stars disrupted by SMBH tidal forces produce bright transient flares observable in sky surveys, with occurrence rates tied to black hole spins (Du et al., 2022). The LSST will measure TDE rates, offering a unique opportunity to detect ultralight bosons with masses in the
On the other hand, TDEs may impact galactic centers by redistributing radiation and heavy elements, alternately sterilizing regions or enhancing conditions for life. LSST’s unprecedented ability to track TDEs and transient events in real time will advance our understanding of galactic habitability. By observing SMBHs influenced by ultralight bosons, LSST will probe how dark matter may indirectly shape life-supporting environments. This example of synergy between fundamental physics and astrobiology highlights LSST’s transformative role in exploring life’s cosmic context.
5.2 Citizen science in astrobiology
Citizen science plays a vital role in analyzing LSST’s vast transient data, bridging gaps not addressed by automated systems (Balcom, 2015; Marshall et al., 2015). Platforms like Zooniverse15, originally developed for Galaxy Zoo (Masters and Galaxy Zoo Team, 2020), enable public participation in projects. SETI@Home pioneered distributed computing for SETI signal analysis (Anderson et al., 2000), and citizen scientists have discovered phenomena such as the unusual light curve of KIC 8462852, linked to exocomets or technosignatures (see Hambleton et al., 2023, and references therein). Independent projects have also explored astrobiology and technosignatures, contributing to the field’s growth (Villarroel et al., 2022).
Integration of Artificial Intelligence (AI) platforms enhances LSST-driven citizen science by enabling efficient anomaly detection within its vast data streams (Villarroel et al., 2022). Symbiotic learning, where humans and AI iteratively refine training sets, combines human intuition with machine precision to improve outcomes (Barrat, 2013). Projects like Backyard Worlds16 and Planet Hunters TESS17 demonstrate this approach by involving volunteers in identifying fast-moving objects and exoplanet transits, while SuperWASP and Supernova Hunters apply similar methods to classify light curves and detect real transients. These strategies could, and indeed should, be adapted to LSST’s unprecedented data volumes, enhancing the potential for meaningful discoveries. Moreover, there are growing varieties of applications of self-suprevised learning algorithms that do not require prelabeled data in astronomical problems (Mesarcik et al., 2023). Such algorithms can be used in conjunction with humans for citizen science by enabling systems to label and interpret vast amounts of data autonomously, while human volunteers can validate, refine, and provide feedback on the AI’s predictions (see e.g., Jiménez et al., 2023).
The Rubin Observatory partners with Zooniverse on astrobiology-related projects such as Daily Minor Planet and Active Asteroids18. LSST’s multiepoch surveys are expected to generate unprecedented transient data volumes, making citizen scientists essential for identifying anomalies that automated pipelines might miss. By leveraging platforms like Zooniverse, the public could be trained to search for biosignatures (e.g., water or oxygen absorption lines) and technosignatures (e.g., artificial signals or unusual periodicities) within LSST’s data.
Citizen scientists could further contribute to multimodal investigations by identifying coincident signals, such as gamma-ray bursts associated with gravitational wave detections, revealing environments near neutron star mergers. LSST’s observations of exoplanet atmospheres may also uncover disequilibrium chemistry indicative of biological activity. These efforts emphasize LSST’s pivotal role in combining public engagement and advanced science to advance astrobiology.
6 Conclusion
The Vera C. Rubin Observatory’s LSST provides the astrobiology community enormous opportunities to develop its search for evidence for life having evolved elsewhere in the universe. In this paper, we have reviewed these opportunities. Through its extensive imaging capabilities it provides methods for detecting biosignatures and for mapping elementary compositions in stars, the ISM and exoplanets that are conducive to determining habitable regions. LSST will significantly extend our inventory of Solar System objects and, beyond the Solar System, survey billions of stars, contributing to the detection of exoplanets, characterizing planetary atmospheres and detecting the presence of flora through search for a ‘red edge’ in surface reflection spectra. Through the study of transient phenomena, LSST may provide a means for searching for technologically advanced civilisations. Thus, we encourage the astrobiology community to exploit the scientific opportunities of the Vera C. Rubin Observatory’s LSST, perhaps through the creation of a specific topical science team working group.
Author contributions
AK: Writing – review and editing, Writing – original draft, Visualization, Methodology, Conceptualization, Investigation. NM: Methodology, Conceptualization, Writing – review and editing, Supervision, Writing – original draft. AĆ: Supervision, Writing – review and editing.
Funding
The author(s) declare that financial support was received for the research and/or publication of this article. NM recognises funding from the European Union’s Horizon 2020 research and innovation programme project Europlanet-2024-RI grant agreement No 871149. AK acknowledges funding provided by the University of Belgrade - Faculty of Mathematics (the contract 451-03-136/2025-03/200104) through the grants by the Ministry of Science, Technological Development and Innovation of the Republic of Serbia.
Acknowledgments
AK expresses gratitude to the staff and students of the University of Kent Physics and Astronomy Group, as well as the Europlanet Office at the University of Kent, for their support, hospitality, and invaluable discussions that contributed to the content of this paper.
Licenses and Permissions
This manuscript has been coauthored by Fermi Forward Discovery Group, LLC under Contract No. 89243024CSC000002 with the U.S. Department of Energy, Office of Science, Office of High Energy Physics. The United States Government retains and the publisher, by accepting the work for publication, acknowledges that the United States Government retains a non-exclusive, paid-up, irrevocable, worldwide license to publish or reproduce the published form of this work, or allow others to do so, for United States Government purposes. The Department of Energy will provide public access to these results of federally sponsored research in accordance with the DOE Public Access Plan (http://energy.gov/downloads/doe-public-access-plan).
Conflict of interest
The authors declare that the research was conducted in the absence of any commercial or financial relationships that could be construed as a potential conflict of interest.
Generative AI statement
The author(s) declare that no Generative AI was used in the creation of this manuscript.
Publisher’s note
All claims expressed in this article are solely those of the authors and do not necessarily represent those of their affiliated organizations, or those of the publisher, the editors and the reviewers. Any product that may be evaluated in this article, or claim that may be made by its manufacturer, is not guaranteed or endorsed by the publisher.
Supplementary material
The Supplementary Material for this article can be found online at: https://www.frontiersin.org/articles/10.3389/fspas.2025.1594485/full#supplementary-material
Footnotes
1See Sections 2.4 and 3.3.2.3 in https://docushare.lsstcorp.org/docushare/dsweb/Get/LPM-17
2Filter cutoffs: https://github.com/lsst/throughputs/blob/main/baseline/
3https://github.com/lsst/throughputs/blob/main/baseline/README.md
4https://earth.esa.int/eogateway/missions/rapideye
5See also https://www.nao.ac.jp/en/news/science/2024/20240905-subaru.html
6https://science.nasa.gov/solar-system/oort-cloud/
7www.lsst.org/science/science_portfolio
9https://carlsaganinstitute.cornell.edu/data
10https://webb.nasa.gov/content/about/faqs/facts.html
13https://www.nasa.gov/missions/webb/nasas-webb-makes-first-detection-of-heavy-element-from-star-merger/
14https://www.lsst.org/scientists/keynumbers
16https://www.zooniverse.org/projects/coolneighbors/backyard-worlds-cool-neighbors
17https://www.zooniverse.org/projects/nora-dot-eisner/planet-hunters-tess
18https://rubinobservatory.org/explore/citizen-science
References
Albareti, F. D., Prieto, C. A., Almeida, A., Anders, F., Anderson, S., Andrews, B. H., et al. (2017). The 13th data release of the sloan digital sky survey: first spectroscopic data from the sdss-iv survey mapping nearby galaxies at Apache point observatory. Astrophysical J. Suppl. Ser. 233, 25. doi:10.3847/1538-4365/aa8992
Allen, G., Andreoni, I., Bachelet, E., Berriman, G. B., Bianco, F. B., Biswas, R., et al. (2019). Deep learning for multi-messenger astrophysics: a gateway for discovery in the big data era. arXiv e-prints. doi:10.48550/arXiv.1902.00522
Anderson, D., Werthimer, D., Cobb, J., Korpela, E., Lebofsky, M., Gedye, D., et al. (2000). “SETI@home: internet distributed computing for SETI,” in 213 of astronomical Society of the pacific conference series. Editors 99 Bioastronomy, G. Lemarchand, and K. Meech, 511.
Andreoni, I., Coughlin, M. W., Almualla, M., Bellm, E. C., Bianco, F. B., Bulla, M., et al. (2021). Optimizing cadences with realistic light-curve filtering for serendipitous kilonova discovery with vera rubin observatory. Astrophysical J. Suppl. Ser. 258, 5. doi:10.3847/1538-4365/ac3baeac3bae
Arkhypov, O. V., Khodachenko, M. L., and Hanslmeier, A. (2019). Dusty phenomena in the vicinity of giant exoplanets. Astronomy and Astrophycis 631, A152. doi:10.1051/0004-6361/201936521
Arnold, L. F. A. (2005). Transit light-curve signatures of artificial objects. Astrophysical J. 627, 534–539. doi:10.1086/4304371086/430437
Arnouts, S., and Ilbert, O. (2011). LePHARE: photometric analysis for redshift estimate. Astrophys. Source Code Libr. Rec. ascl:1108.009.
Arute, F., Arya, K., Babbush, R., Bacon, D., Bardin, J., Barends, R., et al. (2019). Quantum supremacy using a programmable superconducting processor. Nature 574, 505–510. doi:10.1038/s41586-019-1666-5
Audenaert, J., Bowles, M., Boyd, B. M., Chemaly, D., Cherinka, B., Ciucă, I., et al. (2024). The multimodal universe: enabling large-scale machine learning with 100TB of astronomical scientific data. arXiv e-prints. doi:10.48550/arXiv.2412.02527
Balbi, A., and Tombesi, F. (2017). The habitability of the Milky Way during the active phase of its central supermassive black hole. Sci. Rep. 7, 16626. doi:10.1038/s41598-017-16110-01038/s41598-017-16110-0
Balcom, B. (2015). Improving crowdsourcing and citizen science as a policy mechanism for nasa. New Space 3, 98–116. doi:10.1089/space.2015.0017
Barrat, J. (2013). Our final invention: artificial intelligence and the end of the human era. 1st edn. New York: Thomas Dunne Books.
Bellagamba, F., Meneghetti, M., Moscardini, L., and Bolzonella, M. (2012). Accuracy of photometric redshifts for future weak lensing surveys from space. Mon. Notices R. Astronomical Soc. 422, 553–562. doi:10.1111/j.1365-2966.2012.20632.x1365-2966.2012.20632.x
Bellm, E. C., Burke, C. J., Coughlin, M. W., Andreoni, I., Raiteri, C. M., and Bonito, R. (2022). Give me a few hours: exploring short timescales in rubin observatory cadence simulations. Astrophysical J. Suppl. Ser. 258, 13. doi:10.3847/1538-4365/ac4602
Bello-Arufe, A., Buchhave, L. A., Mendonça, J. M., Tronsgaard, R., Heng, K., Jens Hoeijmakers, H., et al. (2022). Exoplanet atmospheres at high resolution through a modest-size telescope. Fe II in MASCARA-2b and KELT-9b with FIES on the Nordic Optical Telescope. Astronomy and Astrophysics 662, A51. doi:10.1051/0004-6361/202142787
Bianco, F. B., Željko, I., Jones, R. L., Graham, M. L., Marshall, P., Saha, A., et al. (2021). Optimization of the observing cadence for the rubin observatory legacy survey of space and time: a pioneering process of community-focused experimental design. Astrophysical J. Suppl. Ser. 258, 1. doi:10.3847/1538-4365/ac3e72
Boquien, M., Burgarella, D., Roehlly, Y., Buat, V., Ciesla, L., Corre, D., et al. (2019). CIGALE: a python code investigating GALaxy emission. Astronomy Astrophysics 622, A103. doi:10.1051/0004-6361/201834156
Borin, P., Cremonese, G., Marzari, F., and Lucchetti, A. (2017). Asteroidal and cometary dust flux in the inner solar system. Astronomy and Astrophysics 605, A94. doi:10.1051/0004-6361/201730617
Buie, M. W., Spencer, J. R., Porter, S. B., Benecchi, S. D., Parker, A. H., Stern, S. A., et al. (2024). The new horizons extended mission target: arrokoth search and discovery. Planet. Sci. J. 5, 196. doi:10.3847/psj/ad676dad676d
Campargue, A., Karlovets, E. V., Vasilchenko, S. S., and Turbet, M. (2023). The high resolution absorption spectrum of methane in the 10800 - 14000 cm-1 region: literature review new results and perspectives. Phys. Chem. Chem. Phys. 25, 32778–32799. doi:10.1039/D3CP02385K
Clarke, R., Bianco, F., Davenport, J., and Gizis, J. (2023). Every datapoint counts: constraining flare temperatures with differential chromatic refraction in the rubin LSST era. Am. Astronomical Soc. Meet. Abstr. 241.
Clarke, R. W., Davenport, J. R. A., Gizis, J., Graham, M. L., Li, X., Fortino, W., et al. (2024). Every data point counts: stellar flares as a case study of atmosphere-aided studies of transients in the lsst era. Astrophysical J. Suppl. Ser. 272, 41. doi:10.3847/1538-4365/ad4110
Collaboration, V. C. R. O. L. S. S. S., Jones, R. L., Bannister, M. T., Bolin, B. T., Chandler, C. O., Chesley, S. R., et al. (2021). The scientific impact of the vera C. Rubin Observatory’s legacy survey of space and time (LSST) for solar system science. Bull. AAS 53. doi:10.3847/25c2cfeb.d8909f28
Conrad, A., and Veillet, C. (2018). “Simultaneous ground- and space-based observations in the JWST era,”. Optical and infrared interferometry and imaging VI. Editors M. J. Creech-Eakman, P. G. Tuthill, and A. Mérand (Austin, TX, United States: SPIE - International Society for Optics and Photonics), 10701. doi:10.1117/12.2314164
Covone, G., and Giovannelli, D. (2022). Stellar metallicity is a key parameter for the search of Life in the Universe. :doi:10.48550/arXiv.2207.03748
Crockford, S. J. (2009). Evolutionary roots of iodine and thyroid hormones in cell–cell signaling. Integr. Comp. Biol. 49, 155–166. doi:10.1093/icb/icp053
Cuoco, E., Patricelli, B., Iess, A., and Morawski, F. (2022). Computational challenges for multimodal astrophysics. Nat. Comput. Sci. 2, 479–485. doi:10.1038/s43588-022-00288-zs43588-022-00288-z
Davenport, J. R. A. (2016). The kepler catalog of stellar flares. Astrophysical J. 829, 23. doi:10.3847/0004-637x/829/1/230004-637X/829/1/23
Davenport, J. R. A. (2019). SETI in the spatio-temporal survey domain. arXiv e-prints, arXiv:1907, 04443. doi:10.48550/arXiv.1907.04443
Dayal, P., Cockell, C., Rice, K., and Mazumdar, A. (2015). The quest for cradles of life: using the fundamental metallicity relation to hunt for the most habitable type of galaxy. Astrophysical J. Lett. 810, L2. doi:10.1088/2041-8205/810/1/l22041-8205/810/1/L2
Deeg, H. J., and Alonso, R. (2018). “Transit photometry as an exoplanet discovery method,” in Handbook of exoplanets. Editors H. J. Deeg, and J. A. Belmonte (Springer International Publishing AG), 117. doi:10.1007/978-3-319-55333-7_117
de Jong, R. S., Agertz, O., Berbel, A. A., Aird, J., Alexander, D. A., Amarsi, A., et al. (2019). 4MOST: project overview and information for the first call for proposals. Messenger 175, 3–11. doi:10.18727/0722-6691/5117
Do, T., Witzel, G., Gautam, A. K., Chen, Z., Ghez, A. M., Morris, M. R., et al. (2019). Unprecedented near-infrared brightness and variability of Sgr A. Astrophysical J. Lett. 882, L27. doi:10.3847/2041-8213/ab38c3
Döbler, N. A. (2020). The concept of developmental relativity: thoughts on the technological synchrony of interstellar civilizations. Space Policy 54, 101391. doi:10.1016/j.spacepol.2020.101391
Doner, A., Horányi, M., Bagenal, F., Brandt, P., Grundy, W., Lisse, C., et al. (2024). New Horizons Venetia Burney Student Dust Counter Observes Higher than Expected Fluxes Approaching 60 au. Astrophysical J. Lett. 961, L38. doi:10.3847/2041-8213/ad18b0
Douglas, E. S., Debes, J., Mennesson, B., Nemati, B., Ashcraft, J., Ren, B., et al. (2022). Sensitivity of the roman coronagraph instrument to exozodiacal dust. Publ. Astronomical Soc. Pac. 134, 024402. doi:10.1088/1538-3873/ac3f7b
Du, P., Egaña-Ugrinovic, D., Essig, R., Fragione, G., and Perna, R. (2022). Searching for ultra-light bosons and constraining black hole spin distributions with stellar tidal disruption events. Nat. Commun. 13, 4626. doi:10.1038/s41467-022-32301-41038/s41467-022-32301-4
Ertel, S., Defrère, D., Hinz, P., Mennesson, B., Kennedy, G. M., Danchi, W. C., et al. (2020). The HOSTS survey for exozodiacal dust: observational results from the complete survey. Astronomical J. 159, 177. doi:10.3847/1538-3881/ab7817
Fischbach, E., Barnes, V., Cinko, N., Heim, J., Kaplan, H., Krause, D., et al. (2018). Indications of an unexpected signal associated with the gw170817 binary neutron star inspiral. Astropart. Phys. 103, 1–6. doi:10.1016/j.astropartphys.2018.06.001
Fraser, W. C., Porter, S. B., Peltier, L., Kavelaars, J., Verbiscer, A. J., Buie, M. W., et al. (2024). Candidate distant trans-neptunian objects detected by the new horizons Subaru TNO survey. Publ. Astronomical Soc. Jpn. 5, 227. doi:10.3847/PSJ/ad6f9e
Gaia, C., Bailer-Jones, C. A. L., Teyssier, D., Delchambre, L., Ducourant, C., Garabato, D., et al. (2023). Gaia data release 3. The extragalactic content. Astronomy astrophysics 674, A41. doi:10.1051/0004-6361/202243232
Garai, Z. (2018). Light-curve analysis of KOI 2700b: the second extrasolar planet with a comet-like tail. Astronomy and Astrophysics 611, A63. doi:10.1051/0004-6361/201629676
Gilster, P. A. (2013). Slow boat to Centauri: a millennial journey exploiting resources along the Way. J. Br. Interplanet. Soc. 66, 302–311.
Ginsburg, I., Lingam, M., and Loeb, A. (2018). Galactic panspermia. Astrophysical J. Lett. 868, L12. doi:10.3847/2041-8213/aaef2d
Glein, C. R., Grundy, W. M., Lunine, J. I., Wong, I., Protopapa, S., Pinilla-Alonso, N., et al. (2024). Moderate d/h ratios in methane ice on eris and makemake as evidence of hydrothermal or metamorphic processes in their interiors: geochemical analysis. Icarus 412, 115999. doi:10.1016/j.icarus.2024.115999
Greene, T. P., Line, M. R., Montero, C., Fortney, J. J., Lustig-Yaeger, J., and Luther, K. (2016). Characterizing transiting exoplanet atmospheres with JWST. Astrophysical J. 817, 17. doi:10.3847/0004-637X/817/1/17
Gris, P., Regnault, N., Awan, H., Hook, I., Jha, S. W., Lochner, M., et al. (2023). Designing an optimal lsst deep drilling program for cosmology with type ia supernovae. Astrophysical J. Suppl. Ser. 264, 22. doi:10.3847/1538-4365/ac9e58
Guélin, M., and Cernicharo, J. (2022). Organic molecules in interstellar space: latest advances. Front. Astronomy Space Sci. 9, 787567. doi:10.3389/fspas.2022.787567
Hambleton, K. M., Bianco, F. B., Street, R., Bell, K., Buckley, D., Graham, M., et al. (2023). Rubin observatory LSST transients and variable stars roadmap. Publ. Astronomical Soc. Pac. 135, 105002. doi:10.1088/1538-3873/acdb9a
Haqq-Misra, J., Schwieterman, E. W., Socas-Navarro, H., Kopparapu, R., Angerhausen, D., Beatty, T. G., et al. (2022). Searching for technosignatures in exoplanetary systems with current and future missions. Acta Astronaut. 198, 194–207. doi:10.1016/j.actaastro.2022.05.040
Hegde, S., Paulino-Lima, I. G., Kent, R., Kaltenegger, L., and Rothschild, L. (2015). Surface biosignatures of exo-earths: remote detection of extraterrestrial life. Proc. Natl. Acad. Sci. 112, 3886–3891. doi:10.1073/pnas.1421237112
Herbst, E. (2021). Unusual chemical processes in interstellar chemistry: past and present. Front. Astronomy Space Sci. 8. doi:10.3389/fspas.2021.776942
Hinkel, N. R., Hartnett, H. E., and Young, P. A. (2020). The influence of stellar phosphorus on our understanding of exoplanets and astrobiology. Astrophysical J. Lett. 900, L38. doi:10.3847/2041-8213/abb3cb
Hodgkin, S. T., Harrison, D. L., Breedt, E., Wevers, T., Rixon, G., Delgado, A., et al. (2021). Gaia early data release 3. Gaia photometric science alerts. Astronomy and Astrophysics 652, A76. doi:10.1051/0004-6361/202140735
Holmbeck, E. M., Barnes, J., Lund, K. A., Sprouse, T. M., McLaughlin, G. C., and Mumpower, M. R. (2023). Superheavy elements in kilonovae. Astrophysical J. Lett. 951, L13. doi:10.3847/2041-8213/acd9cb
Horner, J., Kane, S. R., Marshall, J. P., Dalba, P. A., Holt, T. R., Wood, J., et al. (2020). Solar system physics for exoplanet research. Publ. Astronomical Soc. Pac. 132, 102001. doi:10.1088/1538-3873/ab8eb9
Ivezić, Ž., Beers, T. C., and Jurić, M. (2012). Galactic stellar populations in the era of the sloan digital sky survey and other large surveys. Annu. Rev. Astronomy Astrophysics 50, 251–304. doi:10.1146/annurev-astro-081811-125504
Ivezić, Z., Kahn, S. M., Tyson, J. A., Abel, B., Acosta, E., Allsman, R., et al. (2019). Lsst: from science drivers to reference design and anticipated data products. Astrophysical J. 873, 111. doi:10.3847/1538-4357/ab042c
Jacklin, S., Lund, M. B., Pepper, J., and Stassun, K. G. (2015). Transiting planets with LSST. II. Period detection of planets orbiting 1 M⊙ hosts. Astrophysical J. 150, 34. doi:10.1088/0004-6256/150/1/34
Jiménez, M., Alfaro, E. J., Torres Torres, M., and Triguero, I. (2023). Czsl: learning from citizen science, experts, and unlabelled data in astronomical image classification. Mon. Notices R. Astronomical Soc. 526, 1742–1756. doi:10.1093/mnras/stad2852
Jonathan, G. B., Ryan, J. M., Nikole, K. L., and Lisa, K. (2023). In search of the edge: a bayesian exploration of the detectability of red edges in exoplanet reflection spectra. Astrophysical J. 946, 96. doi:10.3847/1538-4357/acaf59
Jones, R. L., Slater, C. T., Moeyens, J., Allen, L., Axelrod, T., Cook, K., et al. (2018). The large synoptic survey telescope as a near-earth object discovery machine. Icarus 303, 181–202. doi:10.1016/j.icarus.2017.11.033
Kaltenegger, L. (2017). How to characterize habitable worlds and signs of life. Annu. Rev. Astronomy Astrophysics 55, 433–485. doi:10.1146/annurev-astro-082214-122238
Kaltenegger, L., and Faherty, J. K. (2021). Past, present and future stars that can see earth as a transiting exoplanet. Nature 594, 505–507. doi:10.1038/s41586-021-03596-y
Kempton, E. M. R., Lupu, R., Owusu-Asare, A., Slough, P., and Cale, B. (2017). Exo-transmit: an open-source code for calculating transmission spectra for exoplanet atmospheres of varied composition, Publ. Astron. Soc. Pac., 129, 044402. doi:10.1088/1538-3873/aa61ef
Kopparapu, R. K., Ramirez, R., Kasting, J. F., Eymet, V., Robinson, T. D., Mahadevan, S., et al. (2013). Habitable zones around main-sequence stars: new estimates. Astrophysical J. 765, 131. doi:10.1088/0004-637X/765/2/131
Kovačević, A. B., Dizdarević, V., Lazić, J., and Jevremović, I. (2024). “Quantum neural processes for astrophysical signal detection in red noise environments,” in Book of abstracts of the artificial intelligence conference, 24. Department of Technical Sciences SASA, Mathematical Institute SASA, and the Academy Committee for Artificial Intelligence SASA Belgrade, Serbia: Serbian Academy of Sciences and Arts SASA. Available online at: https://www.mi.sanu.ac.rs/ai_conf/previous_editions/2024/AI_Conference_Book_of_Abstracts.pdf; Publisher: Serbian academy of sciences and arts, Location: Belgrade.
Kume, A. (2017). Importance of the green color, absorption gradient, and spectral absorption of chloroplasts for the radiative energy balance of leaves. J. Plant Res. 130, 501–514. doi:10.1007/s10265-017-0910-z
Lacki, B. C. (2021). Life in elliptical galaxies: hot spheroids, fast stars, deadly comets? Astrophysical J. 919, 8. doi:10.3847/1538-4357/ac0e31
Lahat, D., Adali, T., and Jutten, C. (2015). Multimodal data fusion: an overview of methods, challenges, and prospects. Proc. IEEE 103, 1449–1477. doi:10.1109/JPROC.2015.2460697
Lampton, M. (2000). Optical SETI: the next search frontier. Editors G. Lemarchand, and K. Meech (San Francisco, California: Astronomical Society Pacific Conference Series), 565.
Lauretta, D. S., Connolly Jr, H. C., Aebersold, J. E., Alexander, C. M. O., Ballouz, R.-L., Barnes, J. J., et al. (2024). Asteroid (101955) bennu in the laboratory: properties of the sample collected by osiris-rex. Meteorit. and Planet. Sci. 59, 2453–2486. doi:10.1111/maps.14227
Learned, J. G., Pakvasa, S., and Zee, A. (2009). Galactic neutrino communication. Phys. Lett. B 671, 15–19. doi:10.1016/j.physletb.2008.11.057
Lehmer, O. R., Catling, D. C., Parenteau, M. N., Kiang, N. Y., and Hoehler, T. M. (2021). The peak absorbance wavelength of photosynthetic pigments around other stars from spectral optimization. Front. Astronomy Space Sci. 8, 111. doi:10.3389/fspas.2021.689441
Levan, A. J., Gompertz, B. P., Salafia, O. S., Bulla, M., Burns, E., Hotokezaka, K., et al. (2024). Heavy-element production in a compact object merger observed by jwst. Nature 626, 737–741. doi:10.1038/s41586-023-06759-1
Levasseur-Regourd, A. C., Baruteau, C., Lasue, J., Milli, J., and Renard, J. B. (2020). Linking studies of tiny meteoroids, zodiacal dust, cometary dust and circumstellar disks. Planet. Space Sceince 186, 104896. doi:10.1016/j.pss.2020.104896
Li, W., Yin, Z., Li, X., Ma, D., Yi, S., Zhang, Z., et al. (2024). A hybrid quantum computing pipeline for real world drug discovery. Sci. Rep. 14, 16942. doi:10.1038/s41598-024-67897-8
Liu, W., Werthimer, D., Lee, R., Antonio, F., Aronson, M., Brown, A., et al. (2020). “Panoramic SETI: overall focal plane electronics and timing and network protocols. In Ground-based and Airborne Instrumentation for Astronomy VIII,” in 11447 of Society of photo-optical instrumentation engineers (SPIE) conference series. Editors C. J. Evans, J. J. Bryant, and K. Motohara doi:10.1117/12.2561203114477G
Lund, M. B., Pepper, J., and Stassun, K. G. (2015). Transiting planets with LSST. I. Potential for LSST exoplanet detection. Astrophyscal J. 149, 16. doi:10.1088/0004-6256/149/1/16
Madhusudhan, N. (2019). Exoplanetary atmospheres: key insights, challenges, and prospects. Annu. Rev. Astronomy Astrophysics 57, 617–663. doi:10.1146/annurev-astro-081817-051846
Majewski, S. R., Schiavon, R. P., Frinchaboy, P. M., Allende Prieto, C., Barkhouser, R., Bizyaev, D., et al. (2017). The Apache point observatory galactic evolution experiment (APOGEE). Astronomical J. 154, 94. doi:10.3847/1538-3881/aa784d
Marshall, P. J., Lintott, C. J., and Fletcher, L. N. (2015). Ideas for citizen science in astronomy. Annu. Rev. Astronomy Astrophysics 53, 247–278. doi:10.1146/annurev-astro-081913-035959
Masters, K. L.Galaxy Zoo Team (2020). “Twelve years of galaxy Zoo,”. Galactic dynamics in the era of large surveys. Editors M. Valluri, and J. A. Sellwood (Cambridge, United Kingdom: Cambridge University Press), 205–212. doi:10.1017/S1743921319008615
Megias Homar, G., Meyers, J. M., and Kahn, S. M. (2023). Prompt detection of fast optical bursts with the vera C. Rubin observatory. Astrophysical J. 950, 21. doi:10.3847/1538-4357/accb93
Mesarcik, M., Boonstra, A. J., Iacobelli, M., Ranguelova, E., de Laat, C. T. A. M., and van Nieuwpoort, R. V. (2023). The ROAD to discovery: machine-learning-driven anomaly detection in radio astronomy spectrograms. Astronomy and Astrophysics 680, A74. doi:10.1051/0004-6361/202347182
Muller, R. A., and MacDonald, G. J. (1997). Glacial cycles and astronomical forcing. Science 277, 215–218. doi:10.1126/science.277.5323.215
Murgas, F., Chen, G., Nortmann, L., Palle, E., and Nowak, G. (2020). The GTC exoplanet transit spectroscopy survey. XI. Possible detection of Rayleigh scattering in the atmosphere of the Saturn-mass planet WASP-69b. Astronomy Astrophysics 641, A158. doi:10.1051/0004-6361/202038161
NASA Technosignatures Workshop Participants (2018). NASA and the search for technosignatures: a report from the NASA technosignatures Workshop. doi:10.48550/arXiv.1812.08681
Nilsson, R., Liseau, R., Brandeker, A., Olofsson, G., Pilbratt, G. L., Risacher, C., et al. (2010). Kuiper belts around nearby stars. Astronomy and Astrophysics 518, A40. doi:10.1051/0004-6361/201014444
Nishino, Y., and Seto, N. (2018). The search for extra-galactic intelligence signals synchronized with binary neutron star mergers. Astrophysical J. Lett. 862, L21. doi:10.3847/2041-8213/aad33d
Ohashi, N., Tobin, J. J., Jørgensen, J. K., Takakuwa, S., Sheehan, P., Aikawa, Y., et al. (2023). Early planet formation in embedded disks (edisk). i. overview of the program and first results. Astrophysical J. 951, 8. doi:10.3847/1538-4357/acd384
O’Malley-James, J. T., and Kaltenegger, L. (2018). The vegetation red edge biosignature through time on earth and exoplanets. Astrobiology 18, 1123–1136. PMID: 30204495. doi:10.1089/ast.2017.1798
O’Malley-James, J. T., and Kaltenegger, L. (2019). Expanding the timeline for earth’s photosynthetic red edge biosignature. Astrophysical J. Lett. 879, L20. doi:10.3847/2041-8213/ab2769
Perkins, H. M. L., Ellis, J., Fields, B. D., Hartmann, D. H., Liu, Z., McLaughlin, G. C., et al. (2024). Could a kilonova kill: a threat assessment. Astrophysical J. 961, 170. doi:10.3847/1538-4357/ad12b7
Pham, D., and Kaltenegger, L. (2021). Color classification of Earth-like planets with machine learning. Mon. Notices R. Astronomical Soc. 504, 6106–6116. doi:10.1093/mnras/stab1144
Puzzarini, C., and Alessandrini, S. (2024). Interstellar ices: a factory of the origin-of-life molecules. ACS Central Sci. 10, 13–15. doi:10.1021/acscentsci.3c01636
Quanz, S. P., Ottiger, M., Fontanet, E., Kammerer, J., Menti, F., Dannert, F., et al. (2022). Large Interferometer for Exoplanets (LIFE). I. Improved exoplanet detection yield estimates for a large mid-infrared space-interferometer mission. Astronomy and Astrophysics 664, A21. doi:10.1051/0004-6361/202140366
Ragosta, F., Ahumada, T., Piranomonte, S., Andreoni, I., Melandri, A., Colombo, A., et al. (2024). Kilonova parameter estimation with LSST at vera C. Rubin observatory. Astrophysical J. 966, 214. doi:10.3847/1538-4357/ad35c1
Ramsay, G., Kolotkov, D., Doyle, J. G., and Doyle, L. (2021). Transiting exoplanet survey satellite (TESS) observations of flares and quasi-periodic pulsations from low-mass stars and potential impact on exoplanets. Sol. Phys. 296, 162. doi:10.1007/s11207-021-01899-x
Reach, W. T., Franz, B. A., Weiland, J. L., Hauser, M. G., Kelsall, T. N., Wright, E. L., et al. (1995). Observational confirmation of a circumsolar dust ring by the COBE satellite. Nature 374, 521–523. doi:10.1038/374521a0
Renaud, N., Rodríguez-Sánchez, P., Hidding, J., and Broekema, P. C. (2024). Quantum radio astronomy: quantum linear solvers for redundant baseline calibration. Astronomy Comput. 47, 100803. doi:10.1016/j.ascom.2024.100803
Rigley, J. K., and Wyatt, M. C. (2022). Comet fragmentation as a source of the zodiacal cloud. Mon. Notices R. Astronomical Soc. 510, 834–857. doi:10.1093/mnras/stab3482
Rousselot, P., Lombard, F., and Moreels, G. (1999). Search for Trans-Neptunian objects: an automated technique applied to images obtained with the UH 8k CCD Mosaic Camera. Astronomy and Astrophysics 348, 1035–1039.
Saha, S., Basak, S., Safonova, M., Bora, K., Agrawal, S., Sarkar, P., et al. (2018). Theoretical validation of potential habitability via analytical and boosted tree methods: an optimistic study on recently discovered exoplanets. Astronomy Comput. 23, 141–150. doi:10.1016/j.ascom.2018.03.003
Santos, A. D., Fischbach, E., and Gruenwald, J. T. (2020). Synchronized neutrino communications over intergalactic distances. arXiv. doi:10.48550/arXiv.2007.05736
Schwamb, M. E., Jones, R. L., Yoachim, P., Volk, K., Dorsey, R. C., Opitom, C., et al. (2023). Tuning the legacy survey of space and time (lsst) observing strategy for solar system science. Astrophysical J. Suppl. Ser. 266, 22. doi:10.3847/1538-4365/acc173
Schwamb, M. E., Volk, K., Wen, H., Kelley, M. S. P., Bannister, M. T., Hsieh, H. H., et al. (2018). A northern ecliptic survey for solar system science. arXiv e-prints. doi:10.48550/arXiv.1812.01149
Sellers, L., Bobrick, A., Martire, G., Andrews, M., and Paulini, M. (2022). Searching for intelligent life in gravitational wave signals Part I: present capabilities and future horizons. arXiv e-prints. doi:10.48550/arXiv.2212.02065
Smith, M. J., and Geach, J. E. (2023). Astronomia ex machina: a history, primer and outlook on neural networks in astronomy. R. Soc. Open Sci. 10, 221454. doi:10.1098/rsos.221454
Stancil, D. D., Adamson, P., Alania, M., Aliaga, L., Andrews, M., Del Castillo, C. A., et al. (2012). Demonstration of communication using neutrinos. Mod. Phys. Lett. A 27, 1250077. doi:10.1142/S0217732312500770
Stark, C. C. (2011). The transit light curve of an exozodiacal dust cloud. Astronomical J. 142, 123. doi:10.1088/0004-6256/142/4/123
Stark, C. C., Boss, A. P., Weinberger, A. J., Jackson, B. K., Endl, M., Cochran, W. D., et al. (2013). A search for exozodiacal clouds with kepler. Astrophysical J. 764, 195. doi:10.1088/0004-637X/764/2/195
Stern, S. A. (1996). Signatures of collisions in the kuiper disk. Astronomy Astrophysics 310, 999–1010.
Suazo, M., Zackrisson, E., Mahto, P. K., Lundell, F., Nettelblad, C., Korn, A. J., et al. (2024). Project hephaistos – ii. dyson sphere candidates from gaia dr3, 2mass, and wise. Mon. Notices R. Astronomical Soc. 531, 695–707. doi:10.1093/mnras/stae1186
Takizawa, K., Minagawa, J., Tamura, M., Kusakabe, N., and Narita, N. (2017). Red-edge position of habitable exoplanets around M-dwarfs. Sci. Rep. 7, 7561. doi:10.1038/s41598-017-07948-5
Tarter, J. (1985). “Searching for extraterrestrials,” in Extraterrestrials. Science and alien intelligence. Editor E. Regis, 167–199.
Tegmark, M. (2008). The mathematical universe. Found. Phys. 38, 101–150. doi:10.1007/s10701-007-9186-9
Tsumura, K., Matsuura, S., Sano, K., Iwata, T., Yano, H., Kitazato, K., et al. (2023). Heliocentric distance dependence of zodiacal light observed by Hayabusa2#. Earth, Planets Space 75, 121. doi:10.1186/s40623-023-01856-x
Tuchow, N., Stark, C., and Mamajek, E. (2024). HPIC: the habitable worlds observatory preliminary input catalog. Astron. J. 167, 139. doi:10.3847/1538-3881/ad25ec
Tyson, J. A., Snyder, A., Polin, D., Rawls, M. L., and Ivezic, Z. (2024). Expected impact of glints from space debris in the LSST. ApJL. 966, L38. doi:10.3847/2041-8213/ad41e6
Villarroel, B., Mattsson, L., Guergouri, H., Solano, E., Geier, S., Dom, O. N., et al. (2022). A glint in the eye: photographic plate archive searches for non-terrestrial artefacts. Acta Astronaut. 194, 106–113. doi:10.1016/j.actaastro.2022.01.039
Wagg, T., Juric, M., Yoachim, P., Kurlander, J., Cornwall, S., Moeyens, J., et al. (2024). Expected impact of rubin observatory LSST on NEO follow-up. arXiv e-prints. doi:10.48550/arXiv.2408.12517
Wehmeyer, B., López, A. Y., Côté, B., K. Pető, M., Kobayashi, C., and Lugaro, M. (2023). Inhomogeneous enrichment of radioactive nuclei in the galaxy: deposition of live 53Mn, 60Fe, 182Hf, and 244Pu into deep-sea archives. Surfing Wave? Astrophysical J. 944, 121. doi:10.3847/1538-4357/acafec
Wigner, E. P. (1995). The unreasonable effectiveness of mathematics in the natural sciences. Berlin, Heidelberg: Springer Berlin Heidelberg, 534–549. doi:10.1007/978-3-642-78374-6_41
Winter, A. J., Benisty, M., and Andrews, S. M. (2024). Planet formation regulated by galactic-scale interstellar turbulence. Astrophysical J. Lett. 972, L9. doi:10.3847/2041-8213/ad6d5d
Wright, J. T. (2018). Taxonomy and jargon in seti as an interdisciplinary field of study. arXiv Pop. Phys. doi:10.48550/arXiv.1803.06972
Wright, J. T., Haqq-Misra, J., Frank, A., Kopparapu, R., Lingam, M., and Sheikh, S. Z. (2022). The case for technosignatures: why they may be abundant, long-lived, highly detectable, and unambiguous. Astrophysical J. Lett. 927, L30. doi:10.3847/2041-8213/ac5824
Wright, S., Antonio, F. P., Aronson, M. L., Chaim-Weismann, S. A., Cosens, M., Drake, F. D., et al. (2019). Panoramic SETI: an all-sky fast time-domain observatory. Bull. Am. Astronomical Soc. 51, 264.
Wu, Z., Ouyang, G., Shi, X., Ma, Q., Wan, G., and Qiao, Y. (2014). Absorption and quantitative characteristics of C-H bond and O-H bond of NIR. Opt. Spectrosc. 117, 703–709. doi:10.1134/S0030400X1411023X
Yang, G., Boquien, M., Buat, V., Burgarella, D., Ciesla, L., Duras, F., et al. (2020). X-CIGALE: fitting AGN/galaxy SEDs from X-ray to infrared. Mon. Notices R. Astronomical Soc. 491, 740–757. doi:10.1093/mnras/stz3001
Keywords: time-domain surveys, astrobiology, exoplanet biosignatures, technosignatures detection, survey-methods:statistical
Citation: Kovačević AB, Mason NJ and Ćiprijanović A (2025) Multiscale astrobiology with the Vera C. Rubin Observatory Legacy Survey of Space and Time. Front. Astron. Space Sci. 12:1594485. doi: 10.3389/fspas.2025.1594485
Received: 16 March 2025; Accepted: 21 April 2025;
Published: 21 May 2025.
Edited by:
Josep M. Trigo-Rodríguez, Spanish National Research Council (CSIC), SpainReviewed by:
Benedetto Di Ruzza, University of Foggia, ItalyLouis Neal Irwin, The University of Texas at El Paso, United States
Copyright This work is authored in part by Andjelka B. Kovačević, Nigel J. Mason, and Aleksandra Ćiprijanović © Fermi Forward Discovery Group, LLC and Kovačević, Mason and Ćiprijanović. This is an open-access article distributed under the terms of the Creative Commons Attribution License (CC BY). The use, distribution or reproduction in other forums is permitted, provided the original author(s) and the copyright owner(s) are credited and that the original publication in this journal is cited, in accordance with accepted academic practice. No use, distribution or reproduction is permitted which does not comply with these terms.
*Correspondence: Andjelka B. Kovačević, YW5kamVsa2Eua292YWNldmljQG1hdGYuYmcuYWMucnM=