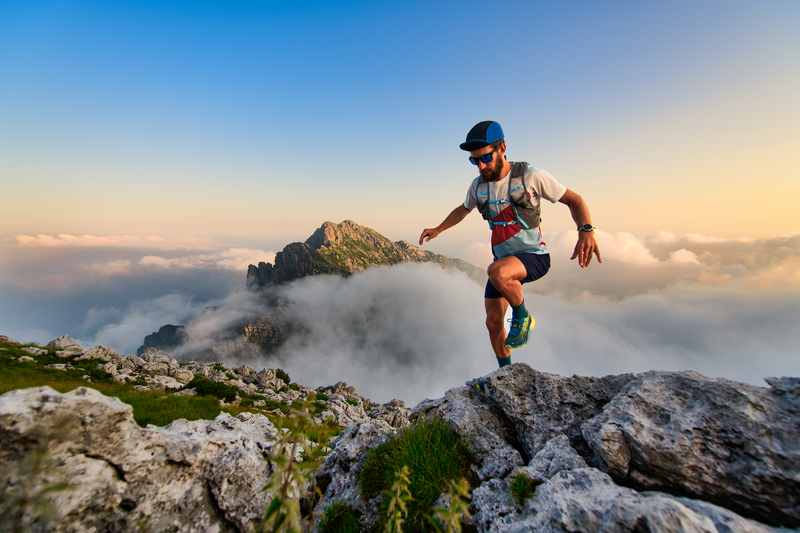
95% of researchers rate our articles as excellent or good
Learn more about the work of our research integrity team to safeguard the quality of each article we publish.
Find out more
REVIEW article
Front. Astron. Space Sci. , 19 March 2025
Sec. Astrobiology
Volume 12 - 2025 | https://doi.org/10.3389/fspas.2025.1544426
This article is part of the Research Topic Habitability Across the Solar System and Exoplanets View all articles
The factors that enable life to begin define the difference between an inhabited planet and one that is simply habitable. While used extensively in Origins, Worlds, and Life: A Decadal Strategy for Planetary Science and Astrobiology 2023–2032 (abbreviated “OWL” in this paper), the term origin of life is never mentioned in Pathways to Discovery in Astronomy and Astrophysics for the 2020s (notated as “Astro2020” in this paper). While the chapters on the search for life in the solar system in OWL treat the origin of life as a central concept, the exoplanet focused chapters of the OWL and Astro2020 reports mostly do not mention origin of life science, particularly with respect to how it intersects with biosignature identification and interpretation. To begin to fill this gap, we describe the set of conditions that are required for life to begin and suggest that they may be distinct from those that make an environment habitable. Finally, we present a putative list of origin of life processes that may be observable on exoplanets and outline the relevance of future planetary science and astrophysics missions to this topic. Given the complexity of detecting these conditions beyond the solar system, we argue that while looking for signs of the origin of life on exoplanets could be fruitful in determining which are worthy of further study, the concept is likely more appropriate for distinguishing true biosignatures from false positives. Ensuring that future facilities like the Large Interferometer For Exoplanets (LIFE) and the Habitable Worlds Observatory (HWO) can constrain origin of life conditions is necessary for life detection searches beyond the solar system.
Beginning with the Viking missions in the 1970s, the National Aeronautics and Space Administration (NASA) has spent the last 50 years in pursuit of an answer to the question of “are we alone in the universe?.” Although the Viking missions did not find unambiguous signs of life, the initiative played an important role in characterizing the atmosphere and surface of Mars (Soffen, 1976), providing the first pictures ever taken from the surface of the red planet. At the turn of the century, NASA Mars science was reclassified as the Mars Exploration Program. Aiming to develop a deeper understanding of Mars’s climate, geology, and potential to host life, the Mars Exploration Program emphasized the search for water on the planet in studying all three of these concepts, enshrining this “follow the water” approach for Martian missions (Hubbard et al., 2002).
In the same decade that the Mars Exploration Program was envisioned, transformative missions to a wide range of different planetary environments in the solar system were launched. The Galileo mission to Jupiter used magnetic data to support previous observations of Europa from Voyager 1 and Voyager 2 that suggested the moon might feature a subsurface ocean (Kivelson et al., 2000). The Cassini-Huygens orbiter and probe explored a variety of surprising environments around Saturn and its natural satellites, including discovering an active plume of water escaping from the small moon Enceladus (Parkinson et al., 2007). Evidence that moons of Saturn and Jupiter might feature global subsurface oceans bolstered NASA’s interest in the search for water on other planets, as did the discovery of water ice on Mars (Feldman et al., 2004) and evidence for past (and perhaps present) liquid water on Mars (Carr and Head, 2010). Plume signals have since been identified in Galileo data of Europa (Jia et al., 2018). The signs of complex organics and molecular hydrogen in plume material from Enceladus (Postberg et al., 2018; Waite et al., 2017) point towards life-bearing conditions in these watery environments.
In 1995, between the launch of Galileo and Cassini, astrophysicists discovered a Jupiter-sized planet orbiting a Sun-like star separated from it by a distance less than that of Mercury from the Sun (Mayor and Queloz, 1995) alongside, in 1992, two planets around a rapidly rotating neutron star (Wolszczan and Frail, 1992). The study of such exosolar planets (exoplanets) in the years since has led to an exploding search for life beyond the solar system. From evidence that planets resembling Earth may orbit more than a fifth of Sun-like stars (Petigura et al., 2013) to the detection of sulfur dioxide produced by the influence of stellar radiation on WASP-39b (Tsai et al., 2023), exoplanet science has lead to a range of fascinating results about the distribution and atmospheric composition of planets in the galaxy. Through the combination of theoretical population synthesis studies like the Generation III Bern Model (Emsenhuber et al., 2021) and observational surveys from the Kepler (Fabrycky et al., 2014) and Transiting Exoplanet Survey Satellite (Yee et al., 2022) missions, there is a growing understanding of how systems, as well as individual planets, behave.
Spectroscopy provided by the James Webb Space Telescope (JWST) is radically changing exoplanetary science. A burgeoning group of planets has been categorized as plausibly Venus-like based on their incident flux (Kane et al., 2019) with some of these objects under study by JWST (Ostberg C. et al., 2023), including TRAPPIST-1 b, which has a surprisingly minimal or nonexistent atmosphere (Greene et al., 2023). In addition to the distinct classes of super-Earths and sub-Neptunes described in Fulton et al. (2017), the bulk density of some compact sub-Neptunes are consistent with a molecular hydrogen rich atmosphere on top of a global liquid water ocean and rocky core (Madhusudhan et al., 2021). These “water worlds” are being observed by a range of JWST programs like Madhusudhan et al. (2023), which reported the first robust detection of methane in a cool exoplanet atmosphere.
Similar to the early “follow the water” principle of the Mars program, exoplanet astrophysics has concentrated on the relevance of the “habitable zone” for life detection and characterization of Earth-like objects. Originating with Kasting et al. (1993), the habitable zone defines the region of space around a given star where liquid water could be stable on a planetary surface, and, depending on its precise definition, encompasses a range of incident fluxes roughly equivalent to that between Venus and Mars. Alongside a hunt for habitable zone exoplanets is the search for signs of life on those objects (Kiang et al., 2007a; Catling et al., 2018; Schwieterman et al., 2018, for instance). The combination of an exoplanet in its star’s habitable zone with distinct lifelike signatures might be indicative of the presence of life on another planet.
Pathways to Discovery in Astronomy and Astrophysics for the 2020s (NASEM, 2023b, Astro 2020) recommends the development a large space observatory capable of characterizing Earth-like planets around Sun-like stars, which is now known as the Habitable Worlds Observatory (HWO). By seeking life on nearby terrestrial planets, this mission aims to put in Earth in context. The search for life within our Solar System has also been emphasized in Origins, Worlds, and Life: A Decadal Strategy for Planetary Science and Astrobiology 2023–2032 (NASEM, 2023a, OWL), which prioritizes a flagship Orbilander mission to Enceladus amongst other mission concepts.
As called out in the NASA Astrobiology Strategy (NASA, 2015), studies of the habitable zone and the “follow the water” approaches previously utilized are not sufficient to fully characterize habitable environments. As studies continue around the plausibility for life to exist across the solar system, increasing attention has been given to which of these environments life would be able of beginning on and how conditions relevant to this process might be detected on terrestrial objects in the solar system (Wong et al., 2022; Deamer et al., 2022; Longo and Damer, 2020). OWL, for instance, prioritizes research around how life started (the origin of life), particularly in “Question 9: Insights from Terrestrial Life.” The study of the origin of life potential of planetary objects is a parallel avenue of inquiry to the direct search for life beyond our planet (Szostak, 2017).
While historical arguments around the origin of life can be traced back to Aristotle (Bailey, 1938), Anaxagoras (Kolb and Clark III, 2020), Darwin (Darwin, 1871), and Arrhenius (Arrhenius, 1908), the contemporary start of origin of life research lies with Oparin and Haldane’s theories for life’s emergence in the 1920s (Lazcano, 2016; Tirard, 2017). The creation of amino acids when sparks and ultraviolet radiation interacted with a hypothetical planetary atmosphere (Miller, 1953; 1955) connected the field of origin of life science to the comparative chemistries of living and nonliving things. Proposals like Woese (1979) and Wächtershäuser (1990) alongside the similarities between the acetyl-CoA metabolic pathway and serpentinization reactions at alkaline hydrothermal vents (Russell and Martin, 2004) have led to a range of theories on if life began capable of transforming simple carbon compounds into complex organics it could use as a food source (autotrophy). Conversely, discoveries of life-relevant chemistry tied to hydrogen cyanide has lead to theories which contend that life emerged using larger carbon molecules that formed geologically (heterotrophy) (Sutherland, 2016). Connected to the question of life’s initial metabolism are those around the environment it emerged in, with proposals including alkaline hydrothermal vents at the ocean floor (Russell, 2007), hot springs on volcanic islands (Damer and Deamer, 2020), and surface alkaline lakes (Toner and Catling, 2019) amongst others (Rodriguez et al., 2024a).
As the origin and evolution of life beyond Earth may require different conditions and show different signatures than that on our own planet (Grefenstette et al., 2024), it is important that we begin our discussion with definitions of life, habitability, the origin of life, and biosignatures.
Definition 1.1. (Life). Coming up with a specific and universally applicable definition for life is one of the overarching goals of astrobiology (Schaible et al., 2024a). While we do not seek to define life here, we choose to adopt NASA’s working definition for life as that of “a self-sustaining chemical system capable of Darwinian evolution.”
Definition 1.2. (Habitability). A habitable environment has (a) the appropriate sources and quantity of energy, solvents, and nutrients in (b) the right range of life-favorable conditions (temperature, pressure, pH, water activity etc.) over (c) time (Hoehler, 2007; Cockell et al., 2016). We adopt this definition of habitability but neglect the localized and temporal conditions (b) and (c) for reasons we describe in Section 3. In this framework, a planet could be declared plausibly habitable after detections of sources of energy, solvent, and nutrients relevant to life’s survival. As detections of conditions like these are required for a habitability diagnosis, Earth remains the only confirmed habitable object (Styczinski et al., 2024). This definition of habitability is distinct from that of an object in the habitable zone, which only requires that the body exist in the region where liquid water could be stable on its surface (Kasting et al., 1993).
Definition 1.3. (The Origin of Life). The origin of life on Earth (sometimes called abiogenesis) is a subject of competing contemporary research efforts that may need to be combined to build a fuller understanding of life’s beginnings (Lane and Xavier, 2024). As life is yet undefined, it is impossible to precisely label the first forms of life and thus the origin of life. Instead, we adopt the premise of A Strategy for Origins of Life Research (Scharf et al., 2015), which contextualizes the origin of life by suggesting that all origin of life research concerns itself with the “the onset of the various organizational phenomena that we associate with the living world.” To provide further intuition, we summarize some of the general steps proposed for the formation of biology on Earth based on Russell (2007) and Damer and Deamer (2020).
1. In the Hadean period 4.56 to 4 billion years ago, the ocean condensed out of Earth’s then carbon dioxide rich atmosphere. Pre-existing volcanism enabled the formation of a range of land and oceanic environments, including hydrothermal environments fueled by magmatic interaction and water/rock reactions. Large scale collisions throughout the solar system during the Hadean period provided the earth with a large flux of organics that were modified by photochemical and geochemical interactions.
2. Reactions in the atmosphere, deep sea hydrothermal vents, surface warm ponds, and/or other related environments enabled the production of complex organic structures relevant to life, which are called prebiotic compounds and comprise prebiotic chemistry.
3. Through some geological or atmospheric process like rehydration and dehydration due to precipitation or absorption inside a hydrothermal vent, these compounds were concentrated, leading to further relevant prebiotic chemistry and eventually the formation of primitive biopolymers including some similar to ribonucleic acid (RNA).
4. These structures were contained into the first membrane-like materials, which are called protocells. The confluence of complex prebiotic chemistry contained in separated units that could divide make up the first protocells.
5. Be it in an ocean floor, pond on a volcanic island, and/or other environment, these protocells then spread from their host environment, adapted to an increasingly broad range of other environments, and intermixed with other lifelike bodies along the way.
6. This adaptation and combination enabled differentiation and evolution to occur in diverse settings. Different metabolisms developed, eventually resulting in the rise of photosynthesis.
Definition 1.4. (Biosignatures). A biosignature is a detectable sign of that is unique to life and can be used to identify life on the early Earth or other worlds. These markers of life’s processes and characteristics can be used to substantiate claims of the presence of a biological process (Neveu et al., 2018). Biosignatures are broadly classified as extant, those indicative of present living material, or extinct, signs of what was once living. In the solar system, a wide range of biosignatures can be studied. Physical biosignatures are micro- and macroscopic evidence of the structure of lifeforms like signs of movement inconsistent with Brownian motion, fossilized remains, and films or other structures tied to life. Chemical biosignatures are markers of life’s influence on local chemistry, including (among others) molecules or remains of molecules tied to biological pathways, an excess of heavy isotopes in an environment plausibly connected to life’s preferential usage of lighter isotopes, minerals corresponding to biological processes like building bones or shells, gases produced by living organisms, and chemical and thermodynamic free energy gradients incapable of occurring geologically. A subset of chemical biosignatures, remote biosignatures are signals of the impact of life across a planet’s atmosphere or surface and are the only kind of biosignature that could be detected on exoplanets. The distinctions between these categories are reviewed in Chou et al. (2024). While chemical and physical biosignatures are produced by life on Earth, life beyond Earth may leave very different traces behind. Proposed agnostic biosignatures aim to be indicative of life as a whole by emphasizing “what life does” instead of “what life looks like” and include highly complex chemistry and polymer-like structures (Grefenstette et al., 2024) alongside measurements of the statistical complexity of observations (Bartlett et al., 2022).
Geological or atmospheric processes can masquerade as life in remote detections. This class of observation is called a false positive. Conversely, seemingly lifeless environments may in fact feature biology. A null detection of biosignatures when life is present is termed a false negative. Differentiating biosignatures from false positives without missing false negatives is a fundamental goal in life detection research. Distinguishing the signs of extant life from cases where life is forming (prebiotic environments) and where life has gone extinct (postbiotic environments) is also essential to properly diagnose a plausible biosignature (Hendrix et al., 2019; Barge et al., 2022).
In this paper, we describe how origin of life concepts might assist exoplanet life detection searches. In Section 2, we contrast how OWL and Astro2020 discuss the origin of life, habitability, and biosignatures. In Section 3, we survey the intersection between the conditions required for planetary habitability and life’s emergence, including references to major open-ended questions about the origin of life on Earth. Based on these constraints, we characterize observables that might indicate that life could begin on an exoplanet in Section 4. In Section 5, we overview developing missions in NASA’s Astrophysics Division (APD) that could detect the observables described in Section 4 and Planetary Science Division (PSD) that may further our understanding of the conditions required for habitability and origin of life explored in Section 3. These results are summarized in Section 6.
Neither OWL nor Astro2020 explicitly define the origin of life. It is worth emphasizing that this topic is central to OWL’s framework for Earth and planetary science. The ninth science question of the survey, “Insights from Terrestrial Life,” asks what a deeper understanding of the processes that led to life on Earth might tell us about how life could emerge and survive on other planets. The origin of life is similarly important to other planetary science and astrobiology science documents like the NASA Astrobiology Strategy (NASA, 2015). The first three chapters of NASA (2015), “Identifying Sources of Abiotic Compounds,” “Synthesis and Function of Macromolecules in the Origin of Life,” and “Early Life and Increasing Complexity,” prioritize deepening research into (a) abiotic chemistry, (b) the development of the nucleic acids and proteins shared by all life on Earth, and (c) the early history of life on Earth. The document posits that this research could reveal what materials life emerged from on Earth, understand how those materials went on to make up the shared building blocks of life, and inform predictions on the broader processes required for life’s emergence and evolution on other astronomical bodies. In addition, origin of life research is highlighted in two of the guiding questions for the future in the final chapter “Challenges and Opportunities in Astrobiology,” “What is life?” and “Can we draw the boundary between prebiotic chemistry and life?”
When noting that different telescopes are needed to answer different questions in astrophysics, the synonym “emergence of life” is mentioned once at the beginning of “Appendix J: Report of the Panel on Electromagnetic Observations from Space 2.” In other exoplanet strategy documents, the origin of life is never described and infrequently referenced. The Exoplanet Science Strategy NASEM (2018) mentions the origin of life in passing when discussing the development of interdisciplinary postdoctoral positions. More recently, the Exoplanet Exploration Program Science Gap List 2023 (Staplefelt and Mamajek, 2023) notes that a gap exists in understanding the range of possible biosignatures (Gap 16, “Complete the Inventory of Remotely Observable Exoplanet Biosignatures and their False Positives”), but they do not explicitly mention the value of origin of life based mitigations to this gap.
Astro2020 focuses extensively on habitability but does not define the term. The survey defines the habitable zone as the region around the star where liquid water could be present, which is in line with the definition presented in OWL. The habitable zone is described as a first order assessment of planetary habitability with a multi-parameter framework (orbital distance, planetary radius, and beyond) deemed necessary (NASA, 2015; NASEM, 2018; 2019; Staplefelt and Mamajek, 2023). Although this concept is not specifically called out in Astro2020, the survey discusses the prevalence of Earth-sized planets in the habitable zone, which Staplefelt and Mamajek (2023) presents as the definition of a potentially habitable exoplanet. In Astro2020, the temporal variation of habitability is alluded to when describing the role stellar evolution, alongside factors like the rise of oxygenic photosynthesis and the delivery of volatiles, can play in planetary habitability.
Habitability is defined in OWL as “the measure of a body’s potential to develop and sustain life,” including “the presence of liquid water, conditions favorable for the assembly of complex organic molecules at some time during the planet’s history, and energy sources to sustain metabolism.” Using the definition presented in An Astrobiology Search for the Search for Life in the Universe (NASEM, 2019), OWL defines dynamic habitability as the set of parameters that influence habitability and vary with time on both the local and global scales. Although exomoons are discussed elsewhere in the document and in NASA (2015), OWL limits the discussion of exoplanet habitability to planets with surface habitability.
Both surveys consider biosignatures. While OWL also examines physical and chemical biosignatures unobservable in the exoplanet context, they do discuss the search for biosignatures beyond the solar system. Astro2020 and OWL both define the ideal biosignature as one that is reliable, made easily by life, survivable, preserved by the environment for enough time to be detected, and detectable, present technology would be able to see evidence of it. The surveys acknowledge the complexities induced by false positives and negatives.
Each survey also defines agnostic biosignatures. Astro2020 claims that an agnostic biosignature is an ideal biosignature that is unlike signatures produced by processes tied to oxygenic photosynthesis and likely manifests through an atmospheric chemical network or signs of disequilibrium. OWL also highlights atmospheric disequilibrium as an example of a potential agnostic biosignature, which it defines as observed atmospheric quantities that are surprisingly complex.
Finally, Astro2020 and OWL prioritize the formulation of standardized methods for assessing the validity of biosignatures like Meadows et al. (2022). In such “biosignature interpretation frameworks,” OWL mentions the importance of environmental characterization, laboratory science, theoretical modeling, and field studies. Astro2020 also calls out environmental (stellar and planetary) characterization and theoretical modeling while incorporating comparison with solar system data in lieu of field work.
The questions and sub-questions posed by “Appendix E: Report for the Panel on Exoplanets, Astrobiology, and the Solar System” in Astro2020 are concentrated in the intersection between planetary habitability, biosignature detection, and biosignature interpretation relevant sections highlighted in Table 1 and Table 2. While the exoplanet focused chapter of OWL “Question 12: Exoplanets” addresses conditions relevant to life and thus both habitability and the origin of life, it also does not specifically call out the importance of origin of life research to exoplanetary science. The sub-questions addressed in each chapter are presented in Table 2. This is reflected in discussions in the Exoplanet Science Strategy and An Astrobiology Search for the Search for Life in the Universe surveys, which frame the overarching goals in exoplanet astrobiology as improving our understanding of habitability, planet evolution, the relationship between planets and systems, and the search for life (NASEM, 2018; 2019).
Table 1. Sections relevant to exoplanets, the origin of life, habitability, and biosignatures in OWL and Astro 2020. These section abbreviations are used when labeling questions in Figure 1; Table 2.
Table 2. Questions relevant to habitability, biosignatures, the origin of life, and exoplanets in Astro2020 and OWL. The section labels for these questions are defined in Table 1.
By contrast, the chapters of OWL that are focused on life and habitability in the solar system (“Question 9: Insights from Terrestrial Life,” “Question 10: Dynamic Habitability,” and “Question 11: The Search for Life Elsewhere”) address how origin of life considerations are implicit in discussions of habitability and biosignatures. These questions are included in Table 2, but, unlike those from the exoplanet relevant sections, we suppress the sub-questions of each topic for clarity.
The overarching questions posed by the Life and Habitability theme in OWL and the priority area, “Pathways to Habitable Worlds,” of the Worlds and Suns in Context theme of Astro2020 are similarly tied to the emergence of life and are included in Table 2. The framing question of “Pathways to Habitable Worlds,” for example, is “Are there habitable planets harboring life elsewhere in the universe?.” However, it is yet unknown what causes life to start on a habitable planet and/or what causes life to form a habitable environment as it evolves. The section titles and abbreviations for all relevant chapters in OWL and Astro2020 are located in Table 1.
In Figure 1, the questions listed in Table 2 are plotted on a Venn diagram where each circle represents the relevance of each question to habitability, the origin of life, and biosignatures. These questions both include ones that are broadly relevant to each of these topics (like PHW-Q2) and those that are tied to specific science objectives around a subset of habitability, biosignatures, and/or the origin of life (see for instance EQ-4a). Figure 1 has some expected features. It is apparent that questions around biosignatures and habitability are plentiful. It is perhaps unsurprising that the origin of life is not discussed independently of habitability and biosignatures in surveys concentrated on the search for life beyond Earth. While the central intersection in Figure 1 is well populated, it is filled mostly with questions that are only implicitly relevant to the origin of life. Excluding this region, the overlap between habitability and the origin of life is only explored in OWL and is not addressed in Astro 2020. This matches the findings of section 2.1. In addition to these anticipated results, Figure 1 shows a gap in the intersection between origin of life considerations and those around biosignatures and habitability. In other words, neither survey quantifies the connection between the origin of life and biosignatures. This region is worthy of further study for two reasons, which are described below.
Figure 1. The questions posed by the themes and sections of OWL and Astro2020 relevant to exoplanet astrobiology are placed in a Venn Diagram to display their relationship to research around habitability (orange), biosignatures (green), and the origin of life (blue). These topics are drawn from chapters in each survey, which are noted in Table 1. In Table 2, we provide the question corresponding to each label in the figure.
The intersection between biosignature research and the origin of life is particularly relevant because habitable planets may not show signs of biosignatures as the conditions necessary for life to begin may be different from those required for life’s survival (see Section 3). Given that the time to detect only water vapor with HWO may be on the order of days or longer (Stark et al., 2024), selecting targets that maximize the chances of finding signs of life is essential. The possibility that the potential for life to start on an exoplanet could be gleaned before a detailed biosignature search is performed on that planet is thus worthy of further investigation.
Life detection searches should be structured around observability, capacity of the object to support life, and its tendency to produce life (NASA, 2015). While doing detailed characterization studies only of planets that show the potential for life to begin and survive would be ideal, it may be difficult to know this information a priori. However, an understanding of the possibility that a certain planet has had an origin of life event could influence the interpretation of potential biosignatures on a habitable world by distinguishing true markers of life from false positives. Conditions that would indicate that an environment has the potential for life to emerge increase confidence that observed biosignatures could have instead been produced by life (Meadows et al., 2022) and provide the context necessary for life detection searches with Bayesian analysis (Catling et al., 2018). This strategy has been used to show that a biotic explanation of observations of Enceladus from the Cassini-Huygens mission to Saturn and its moons is favored, even if the origin of life potential of the moon is unconstrained (Barge and Rodriguez, 2021). Biosignature searches may also provide advances in our understanding of the origin of life on Earth (Rimmer et al., 2021). Recent work suggests that signatures of prebiotic chemistry may be detectable on exoplanets (Claringbold et al., 2023), which could help narrow the parameter space in understanding how chemical processes can lead to biological outcomes.
While habitability refers to a set of conditions related to life’s survival, an origin of life event is a process by which life can begin. These two distinctions (conditions vs. process, survival vs. emergence) distinguish the concept of the origin of life from habitability. While the process(es) that led to life on Earth is/are uncertain, the conditions required for life to start can be broadly constrained in a manner similar to how the requirements for habitability inform where life could thrive. This is the subject of Section 3 and Figure 2. In the following, we describe the conditions required for habitability, detail the relevance of a related set of conditions to the origin of life, and connect these conditions to modern origin of life on Earth theories.
Figure 2. Eight planetary bodies are categorized by plausible present habitability and potential to host an origin of life event based on the conditions discussed in Section 3. The rationale for their positions is described in Section 3.5.
However, as is apparent in both of the recent decadal surveys, clarifying the specific meaning of these terms is difficult. The definition of habitability is not uniform between astrophysicists, planetary scientists, and astrobiologists. In exoplanet astrobiology literature, habitability and the habitable zone are often synonymous (Schwieterman et al., 2018, for example), while solar system astrobiologists often see these concepts as distinct (Wong et al., 2022, for instance). Even within the solar system astrobiological community, the term is used inconsistently (Cockell et al., 2022). As defined in Section 1, we deem a planet habitable if it contains an environment with a relevant source of solvent, nutrients, and energy. At the highest level, the origin of life will also require a source of energy to power the process, a solvent for life to emerge in, and components (both elemental and prebiotic) for life to be composed of.
The requirements for habitability and the origin of life include some source of solvents, nutrients, and energy. While the definition of habitability in OWL also includes constraints on the local environment, we exclude this factor in this work because these conditions are unobservable in the context of HWO. The conditions that make an environment habitable as well as those that could make an origin of life event possible may not be present for the whole planet’s lifetime and inherently may occur in different periods. While both Astro2020 and OWL emphasize how habitability changes with time, we do not include this consideration in our manuscript because the timeline of the origin of life is currently unconstrained, which makes the topic out of scope. We now explore how these components form habitable environments and how those environments might be distinct from ones where life can begin.
The presence of a solvent, particularly water, is the connecting thread between all definitions of habitability and encompasses a key component of life detection searches in the solar system and beyond (see Section 1). The specific characterization of solvents enables the formation of structures. For life to use a solvent, it needs to have a high dielectric constant to enable regulatory processes, promote hydrophobic reactions to encourage the interactions between life-forming compounds, and have a consistent shape across a range of conditions (Pohorille and Pratt, 2012).
Used by all known life, water fulfills all three of the requirements specified by Pohorille and Pratt (2012) while no other solvent does. These factors amongst others make water useful in proton transport, protein folding, and salt ion separation at cell surfaces (Schwieterman et al., 2018). Water also participates in most biochemical pathways (Brack, 1993) and provides the protons and electrons necessary for many important reactions (do Nascimento Vieira et al., 2020). Due to water’s polarity, hydrocarbons (molecules made of just hydrogen and carbon) tend to repel water while molecules containing carbon, hydrogen, nitrogen, oxygen, and sulfur often are attracted to water. Interactions between these hydrophobic compounds (those that repel water), hydrophilic compounds (those that mix with water), and water itself are complex and useful to life (Brack, 1993). Water is also incredibly cosmically common, being composed of hydrogen and oxygen, the first and third most abundant elements in the universe. Like other volatiles (compounds that are easily vaporized), the distribution of water on planets is determined by accretion as well as impacts. To stay in its liquid form, however, water must be exposed to temperatures within a range from
The presence of water is the one shared requirement of all major origin of life theories. Although different perspectives on the environment life emerged in rely on a variety of local conditions for water that may not be habitable to a wide range of contemporary organisms on Earth, like temperature and pH ranges (Deamer et al., 2022), they assume the presence of substantial bodies of water for life to interact with. Reactions and energy sources commonly suggested as those used by the first forms of life like serpentinization (Russell, 2007) and hydrogen cyanide production (Sutherland, 2016) also must occur in bodies of water, be they the deep ocean or intermixing streams on a planetary surface.
The interaction between water and biologically relevant compounds leads to hydrolysis, a process by which a water molecule divides to split an organic compound into two sub-compounds attached to a hydroxyl group and proton, respectively. It is worth noting that this property can challenge the survivability of life (do Nascimento Vieira et al., 2020). It then seems possible that life elsewhere might use other (potentially more ideal) solvents. With the discovery of Titan’s active hydrologic methane cycle (as reviewed in Hayes, 2016), methane has become another astrobiologically relevant solvent, although it lacks the polarity of water. Like water, methane is a volatile and can be delivered to a planet or generated through geo- and biochemical processes. Other potentially relevant solvents include ammonia, sulfuric acid, and formamide (Grefenstette et al., 2024). If solvents like methane prove useful to life, alternative habitable zone definitions could be considered for exoplanetary systems that encompass insolation fluxes appropriate to keep other solvents in their liquid forms (Rimmer et al., 2021).
Hydrolysis is actively managed by living things. Before life established itself on Earth, however, there were no such living structures to control water’s tendency to split compounds that could be relevant to biology. Thus, the effects water has on the survivability of life are likely especially pronounced at its origin and may require a mechanism to counteract (do Nascimento Vieira et al., 2020). While less discussed than water, lifelike components can emerge in other solvents, such as the formation of reverse lipid bilayer vesicles in the non-polar solvent decane (Kunieda et al., 1991).
As elucidated by OWL, all known life is made up of compounds and molecules that stem from carbon, hydrogen, nitrogen, oxygen, phosphorus, and sulfur (CHNOPS). Certain metals are also essential to terrestrial life, including sodium, potassium, magnesium, calcium, iron and others (Colón-Santos et al., 2024; Scharf, 2009). Although arguments have been made for other basic building blocks including silicon, boron, metal-oxides, and sulfur (Grefenstette et al., 2024), carbon chemistry is versatile and carbon itself is incredibly abundant (Schwieterman et al., 2018). The relevance of simple and complex building blocks to life on Earth is described in further detail in Colón-Santos et al. (2024) and Rodriguez et al. (2024a).
In addition to being important for life’s processes once they get started, CHNOPS elements are likely key to reactions that bridge the gap between geochemistry and biochemistry (Russell et al., 2010, for example). Complex prebiotic compounds made of CHNOPS elements would be highly relevant to forming the physical components of life and may also be required as a fuel source for early life. However, the full suite of CHNOPS compounds may not be needed for these processes to start. The quantity of required nutrients can be described mathematically and depends on the probability of an origin of life event over time (Scharf and Cronin, 2016).
Both decadal surveys address sources of the compounds containing elemental building blocks. As pointed out in Astro 2020, stellar type, stellar metallicity, disk composition, and planet migration likely impact the accretion of both volatiles and organics by planetary bodies. After accretion, volatile delivery continues through chondrite and comet impacts (see OWL). Nearly half of Earth’s water by mass may have come from cometary impacts (Chyba, 1987), alongside other sources like asteroids (Schaible et al., 2024b). In addition to their presence, the quantity of these compounds is essential to forming a biosphere (Scharf, 2009).
While phosphate, the primary accessible form of phosphorus, is essential to modern life (and thus habitability), it is rare in most geochemical environments. This fact has lead origin of life scientists to suggest that phosphate (and thus phosphorus) might not have played a role in life’s emergence (Wächtershäuser, 1990, for instance). Computational methods have verified that a proto-metabolism that relies on thioester (organic sulfur) compounds could function and replicate the advantages of phosphorus chemistry, including the role phosphate plays in facilitating energetically unfavorable reactions (Goldford et al., 2017). According to theories like these, life requires CHNOS compounds to begin instead of the full CHNOPS set. There are origin of life theories like those that depend on hydrogen cyanide chemistry that suggest that phosphorus is necessary (Powner et al., 2011) and provide environmental explanations for its presence. Alkaline lakes where water only enters via precipitation and escapes via evaporation feature high geologically produced phosphate concentrations due to chemical weathering (Toner and Catling, 2020; Haas et al., 2024), which could be ideal for life’s emergence (Toner and Catling, 2019; 2020; Liu et al., 2021).
Once volatiles arrive at the surface of a planet, the question becomes how they form the complex organics and prebiotic compounds used by life on Earth. As pointed out by Schaible et al. (2024a), while simple CHNOPS bearing compounds would have been available on the early Earth, they would have been in fairly unreactive forms like
All (Earth) origin of life theories converge on the same endpoints: genes, proteins, and cells (Lane and Xavier, 2024). Regardless of mechanism, the complex prebiotic compounds that form these structures must be created to arrive at life as we know it. However, the ways those structures might be made depend on the conditions life began in and may require intensities that are inhospitable to modern life. Beyond the basic need for complex building blocks to form life’s physical structures, different metabolisms that proto-life could have employed present different requirements on prebiotic chemistry. As described in Thweatt et al. (2024), a metabolism is a set of chemical processes that turn nutrients into cellular energy. While some metabolisms create complex organics, others require them for fuel, so different quantities of relevant compounds might be required for life to begin depending on its initial metabolic strategy. We next review the two families of metabolism for life as we know it.
Autotrophs are life forms that reduce compounds with one carbon, generally carbon dioxide, to make organics and, ultimately, power their cellular functionality. Photosynthesis is a classic autotrophic process where carbon dioxide is reduced to sugars like glucose. If the first life was autotrophic, life would have begun with the capacity to reduce at least one single inorganic carbon
Heterotrophs are life forms that use larger carbon molecules present in their environment as fuel. While many life forms, like humans, are heterotrophs, life today has the advantage of being able to use complex organics, like carbohydrates, generated by autotrophic forms of life as a source of energy. If the first forms of life were heterotrophs, they would need to use geochemically produced organics or those delivered from space for biosynthesis. The heterotrophic origin of life hypothesis is appealing because the prebiotic compounds needed to feed heterotrophs can be produced in a laboratory and initially formed organisms would be less complex than an autotrophic counterpart (Lazcano and Miller, 1996). Early heterotrophic metabolisms could include glycolysis and the fermentation of glycine (Lazcano and Miller, 1999), sunlight to gather relevant organics (Blankenship, 2010), or hydrogen cyanide enabled chemistry (Sutherland, 2016). Through reactions with hydrogen cyanide, for example, prebiotic chemists have been able to produce an array of compounds relevant to the origin of life, including 12 amino acids, 2 ribonucleotides, hydrophilic lipids, and simple sugars (Sutherland, 2016; Liu et al., 2021; Ritson and Sutherland, 2013). However, heterotrophy requires that molecules be small enough to pass into the cell membrane, mandating exoenzymes, enzymes that work outside the cell, which were a later evolution on Earth. Thus, a heterotrophic biosphere may have had its difficulties, and life would need to quickly evolve autotrophy in order to thrive. The Last Universal Common Ancestor (LUCA) is thought to have been autotrophic (Weiss et al., 2016).
If the first forms of life were heterotrophs, there must have been a larger flux of complex biologically relevant compounds than if they were autotrophs. There are a range of different manners in which complex building blocks relevant to the origin of life might be generated. We summarize these in the following sections below. See Rodriguez et al. (2024a) for a more detailed review of chemical methods to generate biologically relevant molecules.
Prebiotic chemistry could occur in space with its products falling into a planetary atmosphere (Anders, 1989) or emerge in the atmosphere as the result of an impact (Chyba and Sagan, 1992). In fact, the production of hydrogen cyanide and subsequent organics seems to require a high impact flux like the late heavy bombardment (Ritson et al., 2018). In meteorites, large abundances of amino acids (reviewed in Pizzarello and Shock, 2010), biologically relevant sugars including ribose (Furukawa et al., 2019), and nucleobases (Martins et al., 2008) of extraterrestrial origin have been detected. There is also observational evidence for organics on asteroids (Kaplan et al., 2021). The infall of these compounds into volcanic islands would concentrate them in small pools where life could begin (Deamer et al., 2022). Prebiotic compounds can also be created due to atmospheric effects. One example of this phenomenon is described in Section 3.5.
Photochemical and/or radiation chemistry could also have contributed to the inventory of prebiotic compounds on the early Earth. While Stanley Miller first used electric discharges to promote the production of amino acids (Miller, 1953), he later tested the effectiveness of ultraviolet light for creating organics (Miller, 1955). Given that the early Sun was more active and the early Earth lacked an ozone layer, ultraviolet light could conceivably provide a fairly continuous source of energy to create prebiotic compounds like cyanide from atmospheric gases (Lane, 2015). Ultraviolet light could drive prebiotic chemistry during an M dwarf flare by forming RNA nucleotides and the RNA pyramidine monomers, cytosine and uracil (Ranjan et al., 2017). Hydrogen cyanide chemistry is also enabled via ultraviolet radiation (Powner et al., 2009). While it may lead to prebiotic chemistry, ultraviolet light is much more effective at disassembling matter than it is at creating it (Lane, 2015) although this same intensity could promote variation in prebiotic compounds and mutation of the resultant life (Cnossen et al., 2007). OWL mentions that other potential sources like cosmic rays and charged particles could facilitate the creation of further prebiotic compounds (see Section 3.4.3).
At the turn of the century, the Lost City hydrothermal vent system was discovered, which, unlike other known aquatic hydrothermal systems at the time, is basic (pH
Energy considerations can be seen as the governing principle of habitability because they regulate the availability of solvents and nutrients (Hoehler, 2007; Hand et al., 2007). In oxidation-reduction (redox) reactions, reactants are either oxidized (lose electrons) or reduced (gain electrons) in the process of forming products. One way to make redox reactions run is through the use of proton gradients, where the concentration of protons differs across a membrane measured by a change in pH. All known life uses redox chemistry by forming proton gradients across membrane structures (Lane, 2015). Through this process, life transforms environmental energy into chemically available energy sources.
These reactions allow energy to be exchanged in the form of electrons and maintain the disequilibrium in pH or equivalently charge that enables life and its formation processes. These principles underlie all modern metabolisms, including oxygenic photosynthesis, motion capabilities like bacterial motility, and broad biological principles such as homeostasis. Alongside fermentation used by heterotrophs (Schönheit et al., 2016), OWL outlines three fundamental sources of energy that life exploits through redox reactions, photosynthesis, chemosynthesis, and radiolysis. While unseen on Earth, life may be able to use sources of thermal, gravitational, mechanical, and/or osmotic energy to create alternative gradients. For example, an organism might be capable of using the flow of a solvent to rotate part of its body to activate a protein that could generate ATP (Bartlett and Wong, 2020). Although we focus on the energy sources used by known life forms on Earth, Grefenstette et al. (2024) contrasts these sources with other forms of potentially relevant energy.
A keystone to habitability, energy availability also restricts how life can originate and evolve (Lane, 2015). Like with other relevant conditions, the quality and quantity of an energy source determines its usefulness in proto- and early life metabolic processes (Boiteau and Pascal, 2011). The energy provided by photosynthesis vastly outweighs that generated through other strategies (Deamer and Weber, 2010), and life even at subsurface locations like hot hydrothermal vents directly employs photosynthesis (Beatty et al., 2005) or is otherwise reliant on photosynthesizers (Lane, 2015). However, other energy sources may be significant to life’s origin, given the complexity of the machinery required to make photosynthesis efficient.
Photosynthetic life uses pigments to capture specific wavelengths of stellar energy to form carbohydrates by reducing carbon dioxide. As reviewed by Blankenship (2010), phototrophy generally requires the interaction of several subsystems. Pigments are responsible for absorbing sunlight and sometimes regulation functions and have prebiotic as well as biotic signatures (Rodriguez et al., 2024b). The antennae are groups of pigments used to collect light. Electron transport chains move electrons inside and outside the reaction center through redox reactions. Carbon fixation pathways use solar energy to convert inorganic compounds into organic compounds. Finally, reaction centers use these other components to convert sunlight into complex organics. Nature demonstrates the prevalence of several types of photosynthesis. Green bacteria with pigments like chlorobactene and isorenieratene use iron-sulfur compounds as electron acceptors, while purple bacteria (producing okenane) oxidize water with organic compounds called quinones.
As phylogenetic evidence suggests that LUCA was not a photosynthesizer (Weiss et al., 2016), it seems unlikely that the first forms of life were. Whether or not life emerged capable of some type of proto-photosynthesis, the diverse range of theories for how photosynthesis might have evolved tend to assume that photosynthetic organisms first formed in water based environments (Kiang et al., 2007b) based on the true distribution of photosynthesizers in a variety of watery homes (Nowicka and Kruk, 2016). This fact again points to the importance of a solvent to early and contemporary life. Even though life on Earth probably did not begin capable of photosynthesis, it may have benefited from photosynthetic pigments (Damer and Deamer, 2020) or byproducts of photochemistry (Gaidos et al., 1999). Specifically, reactions caused by stellar energy might have enabled the formation of life’s structures and provided nutrients for heterotrophs (see Section 3.3).
Instead of using the Sun as an input energy source, chemosynthetic organisms use a range of redox reactions enabled by geochemistry. While the quantity of energy available to chemosynthesizers may not be as significant as the Sun on Earth, chemotrophic sources of energy are potentially accessible in a plethora of environments throughout the solar system, supplying energy through redox chemistry with a wide variety of readily accessible compounds (OWL). For a review of plausible chemosynthetic metabolisms on the ocean worlds of Europa and Enceladus, see Weber et al. (2023).
Depending on the specific strategy, life could emerge heterotrophically or autotrophically using a chemosynthetic metabolism, although many origin of life theories that reference chemosynthesis prefer an autotrophic origin. Wächtershäuser (1990) is positioned as directly counter to the heterotrophic emergence theories pioneered by Oparin in the 1920s. Martin et al. (2008) suggests that life began autotrophically with heterotrophs emerging to fill an ecological niche as the consumer of autotrophic organisms using amino acid and purine fermentation (Schönheit et al., 2016).
Radiolytic organisms rely on the redox couples generated by radioactive sources, including elements like uranium and thorium (endogenic radiolysis) and high energy particles (exogenic radiolysis). As described in Bouquet et al. (2017), the production of diatomic hydrogen through the decomposition of water due to radiation has the potential to provide an alternative or additional source of energy for life forms using chemosynthetic sources of energy, like serpentinization and high temperature hydrothermalism. Weber et al. (2023), for example, discusses the potential for radiolytic products created by interactions with ices to enable aerobic metabolisms on Enceladus. Cosmic rays, another source of ionizing radiation, produce important molecules to life in molecular clouds, including water, methanol, carbon dioxide, formaldehyde, and potentially sugars and amino acids (Dartnell, 2011).
Exogenic radiolysis should be readily available on planetary surfaces if the sources have enough energy to pass through the planet’s atmosphere and magnetic fields (Atri, 2016; Grießmeier et al., 2016). While galactic cosmic radiation particles may have a lower flux than other available forms of radiolytic energy, they have energies exceeding 10 GeV, allowing secondary particles to reach the subsurface and provide energy even to planets without a host star (Atri, 2016). High energy particles may also be able to filter to the subsurface. For example, particles accelerated by Jupiter’s magnetic field could enable the production of oxidants like hydrogen peroxide and diatomic oxygen at Europa (Chyba and Hand, 2001).
High energy radiation can generate the molecular hydrogen and complex building blocks used by life while simultaneously damaging living organisms. Atri and Melott (2014), for example, details how muons can damage deoxyribonucleic acid (DNA) while also noting that cosmic rays, including ones from other galaxies (Atri, 2016), could generate biologically relevant compounds. If life emerges heterotrophically, radiolysis could contribute to a prebiotic feedstock for the first forms of life. In life on Earth, radiation has acted as an evolutionary pressure for organisms like radiation-resistant extremophiles (Cavicchioli et al., 2011). This fact suggests that some dose of radiation may not have long term deleterious effects on the survival and propagation of life.
To build further intuition about the intersections between habitability and the origin of life, we categorize eight planetary bodies by plausible habitability and potential to host an origin of life event based on the conditions discussed in Section 3. The temporal nature of each of these factors is important but poorly constrained and thus will be ignored. Earth is the only confirmed habitable object (Styczinski et al., 2024), as well as the only known location that hosts life (and thus must have had an origin of life event). These worlds are compared in Figure 2.
Present Venus is uninhabitable (or at the very least less habitable) and likely cannot host an origin of life event due to factors like its apparent lack of a large body of water. Although there are arguments that life may be possible in the Venusian clouds (Bains et al., 2024), there is a dearth of observational evidence for these scenarios. We classify Venus not habitable or suitable for an origin of life event.
While pure liquid water is likely unstable on Mars’s surface due to its temperature and pressure, there is a plethora of evidence for its presence in ice and gaseous forms, and it may be ubiquitous in the subsurface. The inhospitable nature of the Martian surface suggests that life might need to exist in its subsurface as autotrophs due to the lack of photochemically produced complex organics. Without access to sunlight for photosynthesis, Martian life would likely be chemotrophs. This description of Mars’s habitability is reviewed by McCollom (2006). Studies since have extended the plausible habitability of Mars. Subsurface life on Mars would likely also have access to the products of endogenic radiolysis (Tarnas et al., 2021), for example,. While Mars may have had the potential to form life in the past, it seems likely that life would only exist on the planet if now extinct surface life had migrated to the subsurface (Westall et al., 2013). Thus, we label present Mars as habitable but without the potential for life to begin.
Due to its active plume, direct measurements of water (Parkinson et al., 2007), sizable carbon compounds (Postberg et al., 2018), and other CHNOPS compounds like sodium phosphates (Postberg et al., 2023) have been found on Enceladus. Molecular hydrogen in the plume of Enceladus points to the presence of hydrothermal activity (or potentially water radiolysis) under the surface of the moon (Waite et al., 2017). The combination of these features suggest that Enceladus may have the conditions required for habitability and an origin of life.
On Europa, there are also multiple lines of evidence for the presence of plumes (Jia et al., 2018; Sparks et al., 2016). Furthermore, the moon should have access to a range of energy sources from radiolysis (Hand et al., 2007) and tidal heating (Styczinski et al., 2024). Observations, laboratory experiments, and modeling also suggest that Europa could feature relevant species including
Although the “pale orange dot” (Chicarro, 2009) looks different from potential ocean worlds like Europa and Enceladus, solvents may be prevalent on Titan. Titan shows signs of surface methane lakes (Hayes, 2016) that participate in an active hydrological cycle (Hayes et al., 2018) as well as a subsurface ocean (Nimmo and Pappalardo, 2016). Several relevant energy sources and at least CHNO compounds are also present on the moon (MacKenzie et al., 2021). Titan’s nitrogen-methane atmosphere forms an organic haze, which could yield a higher flux of prebiotic compounds than delivery from space or hydrothermal vents (Trainer et al., 2006). We suggest that Titan could feature habitable and origin of life relevant conditions.
While Earth sized planets around Sun-like stars cannot be rigorously characterized with current observatories, other types of worlds conducive to life have been hypothesized. Many habitable zone planets may be “water worlds,” planets with orders of magnitude more water than Earth, which may be significantly more observable than other terrestrial planets due to their larger sizes and broader range of habitable zones (Madhusudhan et al., 2021). While water detections in potential water world atmospheres would not guarantee the presence of an ocean (Madhusudhan et al., 2021), atmospheric carbon dioxide gas could keep liquid water stable for billions of years (Kite and Ford, 2018). Access to origin of life-relevant hydrothermalism would be limited in these worlds due to the presence of high pressure ices predicted to form at the ocean floor, but the molecular hydrogen rich atmospheres and oceans of these worlds could plausibly contain the components necessary for life to emerge (Madhusudhan, 2024). Life could start on a hypothetical water world but would not have access to sources of energy and nutrients enabled by deep sea hydrothermalism. We suggest that water worlds may be habitable and capable of forming life.
Terrestrial planets around small stars pose another opportunity to observe plausibly life-bearing environments in the short term. However, observations of planets like TRAPPIST-1 b show that atmospheres (and thus oceans) may not exist on these planets (Greene et al., 2023), likely due to the high stellar activity of their host stars (Roettenbacher and Kane, 2017). Without atmospheres or oceans, these planets could be bare rocks (Zieba et al., 2023) and thus would also be unsuitable for the formation or proliferation of life.
When planning future missions designed to characterize exoplanets where life could develop, the capacity to detect relevant factors to the origin of life is essential. While this subject is worthy of its own paper, we outline a few important detection metrics to prioritize when planning exoplanet characterization missions below. Many of these observations are very difficult to perform and could require a significant amount of observing time, which suggests that an origin of life metric may not be an effective tool for target selection but instead a useful technique for separating out false positives from true biosignatures.
Given its relevance to all origin of life conditions, direct detection of exoplanet (sub)surface oceans are essential for an analysis of the planet’s origin of life potential. Detection possibilities include direct imaging (Robinson, 2018), ocean glint (Robinson et al., 2010), polarization measurements (Zugger et al., 2010), and surface mapping (Cowan et al., 2009). Glint techniques have been used in the solar system to determine that Titan’s Kraken Mare is consistent with an ethane or methane lake (Stephan et al., 2010). Because the presence of a solvent, likely water, is crucial to both the emergence and maintenance of life, the capacity to make direct measurements of large bodies of liquid is critical for future life detection and evaluation missions.
Depending on the structure of a given exoplanet system, features like these may be hidden behind the Habitable Worlds Observatory coronagraph. Through an analysis of the initial target star list for HWO (Mamajek and Stapelfeldt, 2024), Vaughan et al. (2023) determined that liquid water could be detected on
By stabilizing surface water amongst other properties, atmospheres are thought to help to facilitate origin of life processes (Schaible et al., 2024b). Signs of volatiles and organics on planets may be visible as potential biosignatures in planetary atmospheres through disk averaged photometry, time-resolved photometry, transmission spectroscopy, and direct imaging over a variety of wavelength ranges (Schwieterman et al., 2018).
Potential biosignatures contain many of the CHNOPS elements in compounds directly relevant to constraining the possibility of origin of life considerations like hydrogen cyanide formation (Pearce et al., 2022). An important aspect of life detection is determining what chemistries and properties are unique to life in order to definitively detect it. Understanding abiotic processes (Barge et al., 2022) is critical for this. In particular, “prebiosignatures” may make up a separate class of observables which indicate that prebiotic chemistry could be favorable on an exoplanet (Rimmer et al., 2021). Claringbold et al. (2023) has found that a range of prebiosignatures, including
Due to its role in absorbing incident radiation, atmospheric composition and extent is more important than stellar emission in distinguishing ultraviolet radiation levels on modern and Archean Earth (Cnossen et al., 2007) and may, alongside clouds, form the primary restriction on the availability of photons for photosynthesis (Kiang et al., 2007a). Signs of atmospheric chemical disequilibrium could indicate potential agnostic biosignatures and, in pair with appropriate stellar irradiation, the availability of free energy gradients (Wong et al., 2022).
Volcanism provides access to free energy gradients and propensity to trigger hydrothermal activity (Schaible et al., 2024b). Many energy sources used by chemosynthetic organisms depend on forms of volcanic activity (Gaidos et al., 1999) and can change in intensity depending on geological parameters like higher mantle temperatures (McCollom and Seewald, 2013). Volcanism may be directly measured in different spectral modes for a range of planet types in the coming years. Ostberg C. M. et al. (2023), for example, found that the shape of
Stellar radiation is essential for photosynthesis and can create relevant prebiotic compounds. While studying the high energy radiation density is important for the latter, measurements of the surface incident spectral photon flux density are critical for diagnosing the photosynthetic potential of an exoplanet (Kiang et al., 2007a). Radiolysis from high energy photons is also constrained by solar energy as well as orbital distance and albedo, factors that Hand et al. (2007) used to model Europa’s energy budget.
The presence and strength of planetary magnetic fields play a role in determining the impact of stellar processes on the planetary environment (Ehlmann et al., 2016). Studying star-planet interactions, such as the Calcium II emission line, has allowed scientists to detect planetary magnetic fields (Cauley et al., 2019). While likely proving of secondary importance to atmospheric extent (Atri, 2016; Grießmeier et al., 2016), planetary fields inhibit the capacity for external radiolytic sources to penetrate a planet’s atmosphere.
We provide a brief overview of the astrophysics missions that are currently under development and have the potential to influence our ability to detect signatures of the origin of life described in Section 4. As highlighted throughout Astro 2020, each mission provides complementary science to multiple subfields of astrophysics. In Table 3, we catagorize how these missions might help answer the overarching questions raised in Astro2020 and OWL described in Section 2.2.
Table 3. How the missions described in Section 5 map to a subset of the questions from Astro2020 and OWL emphasized in 2.2. The labels for questions in this table are given in Tables 1, 2. Some mission and telescope names are abbreviated in this table’s heading. They are: Habitable Worlds Observatory (HWO), Nancy Grace Roman Space Telescope (Roman), next-generation Very Large Array (ngVLA), Extremely Large Telescopes (ELTs), MSR (Mars Sample Return), and Europa Clipper (Clipper).
While missions like JWST have proven capable of studying the thermal emission spectra of small planets (Zieba et al., 2023, for example), gaining a more complete picture of terrestrial exoplanets requires the capacity to characterize Earth-sized planets around Sun-like stars. Proposed by Astro 2020, the Habitable Worlds Observatory (HWO) would be a 6-m class telescope capable of observing in the optical, ultraviolet, and near-infrared. Through the use of an advanced coronagraph, the mission would be capable of directly imaging Earth-sized planets around approximately 100 Sun-like stars and searching for biosignatures in the spectra of about 25 of the most promising planets. An analysis of the habitability and origin of life potential of these planets could feed into planet target selection and provide the context necessary to distinguish abiosignatures, prebiosignatures, and true biosignatures (see Section 2.2). In particular, the Habitable Worlds Observatory may provide access to a suite of prebiosignatures that compliment those observable with JWST (Claringbold et al., 2023) and find surface oceans (Vaughan et al., 2023).
While incredible advances have been made in understanding the statistical distribution of exoplanets (Petigura et al., 2013; Dressing and Charbonneau, 2013; Fulton et al., 2017, for example), gaps in our knowledge remain due to observational biases. One such area is the paucity of known low mass planets that orbit beyond one AU from their host stars. Seeking to rectify this discrepancy alongside tackling other astronomical efforts, the Nancy Grace Roman Space Telescope (Roman) will make use of gravitational microlensing to search for planets, including Earth sized ones, near the Milky Way’s bulge. After its initial proposal in NASEM (2010), Roman’s design was modified to include a coronagraph instrument, which will characterize nearby exoplanets (Carrión-González et al., 2021), protoplanetary disks (Anche et al., 2023), and exozodical dust (Douglas et al., 2022). By illuminating types of planets we could expect to see at moderate planet-star separations and testing the effectiveness of space born coronagraph imaging, the Roman Coragraph will contribute to precursor science and mission design for HWO.
Constraining the evolution of volatiles in protoplanetary disks (see Pontoppidan et al., 2014, for a review of this topic) may allow us to better understand the underlying principles of volatile delivery that make up the first steps towards the formation of prebiosignatures and life itself. With the Atacama Large Millimeter/submillimeter Array (ALMA), planetary disk detections have become increasingly detailed, enabling the study of disk evolution (Yen et al., 2017). Able to view the closer in (10 AU) region of disks where planets likely form, JWST has augmented science done by ALMA by detecting new species in planetary disks, like 13CO2 (Grant et al., 2023), work that will be further bolstered by Roman. This work also connects to studies of the interstellar medium, which has informed our understanding of the generation of prebiotic compounds and their incorporation into the comets and asteroids that create habitable environments (Schaible et al., 2024b). Featuring higher sensitivity and frequency range, the next-generation Very Large Array (ngVLA) would be the successor to the Karl Jansky Very Large Array (JVLA) and the Very Long Baseline Array (VLBA). This program has the potential to constrain the properties of protoplanetary disks at a 20 times finer scale than ALMA, hopefully enabling the detection of planet formation in real time.
Funded by the National Sciences Foundation, the construction of a ground based telescope with mirror diameters of around 30 m would enable incredible advances in high resolution spectroscopy. Current generation very large telescopes like the Keck Telescope have enabled us to directly image and study the spectra of large distant exoplanets like those in the HR 8799 system (Wang et al., 2021). Future extremely large telescopes (ELTs) have the potential to observe and characterize a range of exoplanets, especially if full sky coverage is achieved by building an ELT in each hemisphere. The development of an ELT would feed into the design of HWO’s coronagraph system and contribute to a broader understanding of the diversity of exoplanet atmospheres and system architectures. While HWO may be able to study atmospheres in wavelengths inaccessible to ground based observing, ELTs may still prove effective at finding life-relevant molecules like
Several of the Flagship, New Frontiers, and Discovery class missions currently under development by NASA’s Planetary Science Division are relevant to the origin of life and exoplanet science. We note that, although the missions discussed in Section 5.1 are of interest to origin of life questions, plans to target origin of life markers and exploration strategies pertinent to origin of life science are absent. In Table 3, we also catagorize how these missions might help answer the overarching questions raised in Astro2020 and OWL described in Section 2.2.
The detection (or lack thereof) of life in future missions to solar system objects will provide ground truth information on how well life can begin and survive in different planetary environments. By deepening our understanding of the processes that might underlie the formation of life, upcoming solar system missions could help answer open-ended questions in origin of life research and distinguish the conditions that define habitable environments and those where life can begin. By exploring Venus, solar system science can help develop knowledge and characterize exoplanet classes like exo-Venuses (Ostberg C. et al., 2023), including their potential to host life. Missions to Venus and Mars can provide empirical constraints for habitable zone exoplanets by highlighting the role of divergent evolution on these bodies (NASEM, 2019; Staplefelt and Mamajek, 2023), while missions to potential ocean moons of giant planets may shed light on objects similar to water rich exoplanets (Hendrix et al., 2019).
While a plethora of NASA missions have studied Mars over the years, far fewer have ever reached Venus, especially its surface. While Venus and Earth look very different today, they may have been much more similar in the past, and questions remain on the capability of Venus to host life then or in the present (Kotsyurbenko, 2023). Finding the fundamental distinctions between these planets over time through missions to Venus could help distinguish Venus and Earth-like exoplanets (Styczinski et al., 2024). Furthermore, Venus science provides the opportunity to gain information on the diversity of terrestrial atmospheres and how surface properties can influence these novel atmospheres, topics that are essential in developing more detailed models of exoplanet climates (Kane et al., 2019). DAVINCI (Deep Atmosphere Venus Investigation of Noble gases, Chemistry, and Imaging) will study the formation and evolution of Venus’s atmosphere alongside searching for evidence of a past surface ocean (Garvin et al., 2022). VERITAS (Venus Emissivity, Radio Science, InSAR, Topography, and Spectroscopy) will constrain surface conditions on Venus to evaluate its potential volcanism and plate tectonics as well as studying the rock types present on Venus today (Smrekar et al., 2022).
Titan has liquid hydrocarbon lakes, 1.5 bar nitrogen and methane atmosphere, a wide range of volatiles, a surface rich with organics including key metals, and a likely subsurface liquid water ocean (NASEM, 2019), making the moon of Saturn of immense astrobiological interest. By studying Titan, scientists could develop a better understanding of the range of conditions life might be capable of emerging in, particularly with respect to the usefulness of alternative solvents like methane and atmospheric sources of prebiotic chemistry. The Dragonfly mission is an octocopter which will repeatedly land and take off on Titan to study its surface and atmosphere with the intent of, amongst other science goals, determine the extent of prebiotic chemistry on the moon (Barnes et al., 2021).
As pointed out in Grefenstette et al. (2024), Mars has a compelling set of energy sources, which make it a laboratory to test origin of life hypotheses. Discoveries made from samples returned from Mars’s surface could constrain the prevalence of life on Mars over time, which could lead to radical changes in theories of how life is capable of beginning. Furthermore, missions to Mars seek to understand the potentially biological origin of Mars’s methane, which could inform the role of methane as a biosignature in the studies of exoplanets (NASEM, 2019). Mars Sample Return encompasses a series of missions designed to cache and bring samples back to Earth, beginning with the Mars Perseverance Rover, which is currently collecting and storing samples from Mars’s Jezero Crater. Of particular relevance to this study, the 27 currently cached samples could help determine how water-rock interactions could have acted on the crater floor and their role in forming small habitable regions (Herd et al., 2025).
Well beyond the habitable zone, the ocean moons of giant planets in the solar system form a new class of potentially habitable objects. Missions to these objects could broaden our understanding of habitability and potentially find life, informing our understanding of how life can begin in environments unlike Earth. Europa, which orbits Jupiter, is one plausibly habitable ocean world with interesting properties (see Section 3.5). Seeking to understand the ice and oceans of Europa as well as its geology and composition, the Europa Clipper mission was initially proposed as the Jupiter Europa Orbiter in NASEM (2011) and was adjusted to be a repeated flyby mission. While not a search for life mission, Europa Clipper aims to evaluate the habitability of Europa through a characterization of the ice shell, surface and atmospheric composition, and surface geology (Pappalardo et al., 2024) in a manner which could inform the habitability and origin of life parameter space. For example, the mission will hopefully answer whether seafloor activity has ever been present on Europa and sample plume material for large carbon species (NASEM, 2019), which could determine the energy and nutrient sources possible on the moon.
Exoplanet astrophysics is becoming increasingly linked to solar system planetary science, particularly in the context of the search for life in the universe. The number of mentions of the word “exoplanet” more than tripled between the 2015 and 2019 astrobiology strategy documents (NASA, 2015; NASEM, 2019). The latest astrobiology strategy and exoplanet strategy documents were written in tandem so that leaders on each report could consult on descriptions of habitability and detecting signatures of life (NASEM, 2018; 2019). The most recent planetary science decadal survey, OWL, included a science chapter on exoplanets, which it frames as the connecting thread between all other planetary science research topics. In this review, we have provided an introduction to the fundamental concepts in the study of the origin of life to motivate further research into the study of how life might emerge on an exoplanet, a topic that is already of significant importance to solar system astrobiology.
By comparing the descriptions of habitability, the origin of life, and biosignatures in the astrophysics and planetary science decadal surveys in Section 2, we found that the origin of life is a concept missing from Astro2020 and recent astrophysics strategy documents as a whole, near excluded across the exoplanet chapters of OWL and Astro 2020, and yet central to discussions of the search for life in the solar system in OWL. While the exoplanet chapter in OWL captures some of the implicit relationships between habitability and origin of life, both surveys fail to address the value of origin of life science in validiating plausible biosignatures.
In Section 3, we define a habitable environment as one that contains the appropriate amount of relevant solvent(s), nutrients, and energy source(s). We similarly suggest that a planet has the potential for life to begin on it if it has access to these three fundamental requirements, while noting that the quantity, quality, and type of each could be distinct. In a manner similar to how the conditions required for an environment to be habitable inform whether life could survive on a planet, the conditions required for an origin of life event represent a first attempt at an Earth-informed framework that could be used to describe if life could begin on a planet.
By suggesting a set of observable characteristics of conditions tied to the origin of life in Section 4, we establish a preliminary list of markers that would inform whether an exoplanet could be inhabited. Discussing, expanding, and attempting to simulate and detect these “origin of life observables” is an essential next step in this work. Already, it is apparent that these observables will require time-consuming measurements with highly optimized telescopes. As a result, diagnosing the origin of life potential of an exoplanet may not be an effective tool for choosing which planets to do long term observations on but could be an important step in classifying potential biosignatures and assessing their significance. In Section 5, we summarize upcoming and current Astrophysics and Planetary Science Division missions that could measure these observables or constrain our understanding of how life can begin.
With the development of large space telescopes to search for habitable exoplanets, the discovery of life beyond the solar system is becoming more feasible. By incorporating an understanding of how life emerged on Earth and how it might begin elsewhere, we can improve the odds that we understand the signs of life that we may detect around other stars.
FK: Writing–original draft, Writing–review and editing. TK: Conceptualization, Funding acquisition, Project administration, Supervision, Writing–original draft, Writing–review and editing. LB: Conceptualization, Funding acquisition, Writing–original draft, Writing–review and editing. PC: Conceptualization, Funding acquisition, Writing–original draft, Writing–review and editing. YY: Writing–original draft, Writing–review and editing. JW: Funding acquisition, Supervision, Writing–original draft, Writing–review and editing.
The author(s) declare that financial support was received for the research, authorship, and/or publication of this article. The authors acknowledge funding from the JPL Strategic Research and Technology Development, “Assessing Origin of Life (OOL) Scenarios for Exoplanet Studies.”
This research was carried out at the Jet Propulsion Laboratory, California Institute of Technology, under a contract with NASA (80NM0018D004). Reference herein to any specific commercial product, process, or service by trade name, trademark, manufacturer, or otherwise does not constitute or imply its endorsement by the United States Government or the Jet Propulsion Laboratory, California Institute of Technology. Government sponsorship acknowledged. 2024. All rights reserved.
The authors declare that the research was conducted in the absence of any commercial or financial relationships that could be construed as a potential conflict of interest.
The author(s) declare that no Generative AI was used in the creation of this manuscript.
All claims expressed in this article are solely those of the authors and do not necessarily represent those of their affiliated organizations, or those of the publisher, the editors and the reviewers. Any product that may be evaluated in this article, or claim that may be made by its manufacturer, is not guaranteed or endorsed by the publisher.
Anche, R. M., Douglas, E., Milani, K., Ashcraft, J., Millar-Blanchaer, M. A., Debes, J. H., et al. (2023). Simulation of high-contrast polarimetric observations of debris disks with the roman coronagraph instrument. Publ. Astronomical Soc. Pac. 135, 125001. doi:10.1088/1538-3873/ad0a72
Anders, E. (1989). Pre-biotic organic matter from comets and asteroids. Nature 342, 255–257. doi:10.1038/342255a0
Atri, D. (2016). On the possibility of galactic cosmic ray-induced radiolysis-powered life in subsurface environments in the universe. J. R. Soc. Interface 13, 20160459. doi:10.1098/rsif.2016.0459
Atri, D., and Melott, A. L. (2014). Cosmic rays and terrestrial life: a brief review. Astropart. Phys. 53, 186–190. doi:10.1016/j.astropartphys.2013.03.001
Bailey, C. H. (1938). The origin of life (oparin, a. i.). J. Chem. Educ. 15, 399. doi:10.1021/ed015p399.1
Bains, W., Petkowski, J. J., and Seager, S. (2024). Venus’ atmospheric chemistry and cloud characteristics are compatible with venusian life. Astrobiology 24, 371–385. doi:10.1089/ast.2022.0113
Barge, L. M., and Rodriguez, L. E. (2021). Life on enceladus? it depends on its origin. Nat. Astron. 5, 740–741. doi:10.1038/s41550-021-01382-4
Barge, L. M., Rodriguez, L. E., Weber, J. M., and Theiling, B. P. (2022). Determining the “biosignature threshold” for life detection on biotic, abiotic, or prebiotic worlds. Astrobiology 22, 481–493. doi:10.1089/ast.2021.0079
Barnes, J. W., Turtle, E. P., Trainer, M. G., Lorenz, R. D., MacKenzie, S. M., Brinckerhoff, W. B., et al. (2021). Science goals and objectives for the dragonfly titan rotorcraft relocatable lander. Planet. Sci. J. 2, 130. doi:10.3847/psj/abfdcf
Bartlett, S., Li, J., Gu, L., Sinapayen, L., Fan, S., Natraj, V., et al. (2022). Assessing planetary complexity and potential agnostic biosignatures using epsilon machines. Nat. Astron. 6, 387–392. doi:10.1038/s41550-021-01559-x
Bartlett, S., and Wong, M. L. (2020). Defining lyfe in the universe: from three privileged functions to four pillars. Life 10, 42. doi:10.3390/life10040042
Beatty, J. T., Overmann, J., Lince, M. T., Manske, A. K., Lang, A. S., Blankenship, R. E., et al. (2005). An obligately photosynthetic bacterial anaerobe from a deep-sea hydrothermal vent. Proc. Natl. Acad. Sci. 102, 9306–9310. doi:10.1073/pnas.0503674102
Blankenship, R. E. (2010). Early evolution of photosynthesis. Plant physiol. 154, 434–438. doi:10.1104/pp.110.161687
Boiteau, L., and Pascal, R. (2011). Energy sources, self-organization, and the origin of life. Orig. Life Evol. Biospheres 41, 23–33. doi:10.1007/s11084-010-9209-y
Bouquet, A., Glein, C. R., Wyrick, D., and Waite, J. H. (2017). Alternative energy: production of h2 by radiolysis of water in the rocky cores of icy bodies. Astrophysical J. Lett. 840, L8. doi:10.3847/2041-8213/aa6d56
Brack, A. (1993). Liquid water and the origin of life. Orig. Life Evol. Biosphere 23, 3–10. doi:10.1007/bf01581985
Carr, M. H., and Head, J. W. (2010). Acquisition and history of water on mars. Lakes Mars, 31–67. doi:10.1016/b978-0-444-52854-4.00002-7
Carrión-González, Ó., Muñoz, A. G., Santos, N., Cabrera, J., Csizmadia, S., and Rauer, H. (2021). Catalogue of exoplanets accessible in reflected starlight to the nancy grace roman space telescope-population study and prospects for phase-curve measurements. Astronomy and Astrophysics 651, A7. doi:10.1051/0004-6361/202039993
Catling, C., Kiang, Y., Nancy, R. D., Tyler, R. J., Andrew, G., DasSarma, S., et al. (2018). Exoplanet biosignatures: a framework for their assessment. Astrobiology 18, 709–738. doi:10.1089/ast.2017.1737
Cauley, P. W., Shkolnik, E. L., Llama, J., and Lanza, A. F. (2019). Magnetic field strengths of hot jupiters from signals of star–planet interactions. Nat. Astron. 3, 1128–1134. doi:10.1038/s41550-019-0840-x
Cavicchioli, R., Amils, R., Wagner, D., and McGenity, T. (2011). Life and applications of extremophiles. Environ. Microbiol. 13, 1903–1907. doi:10.1111/j.1462-2920.2011.02512.x
Chou, L., Grefenstette, N., Borges, S., Caro, T., Catalano, E., Harman, C. E., et al. (2024). Chapter 8: searching for life beyond earth. Astrobiology 24, S-164–S-185. doi:10.1089/ast.2021.0104
Chyba, C., and Sagan, C. (1992). Endogenous production, exogenous delivery and impact-shock synthesis of organic molecules: an inventory for the origins of life. Nature 355, 125–132. doi:10.1038/355125a0
Chyba, C. F. (1987). The cometary contribution to the oceans of primitive earth. Nature 330, 632–635. doi:10.1038/330632a0
Chyba, C. F., and Hand, K. P. (2001). Life without photosynthesis. Science 292, 2026–2027. doi:10.1126/science.1060081
Claringbold, A., Rimmer, P., Rugheimer, S., and Shorttle, O. (2023). Prebiosignature molecules can be detected in temperate exoplanet atmospheres with jwst. Astronomical J. 166, 39. doi:10.3847/1538-3881/acdacc
Cnossen, I., Sanz-Forcada, J., Favata, F., Witasse, O., Zegers, T., and Arnold, N. F. (2007). Habitat of early life: solar x-ray and uv radiation at earth’s surface 4–3.5 billion years ago. J. Geophys. Res. Planets 112. doi:10.1029/2006je002784
Cockell, C. S., Bush, T., Bryce, C., Direito, S., Fox-Powell, M., Harrison, J. P., et al. (2016). Habitability: a review. Astrobiology 16, 89–117. doi:10.1089/ast.2015.1295
Cockell, C. S., Samuels, T., and Stevens, A. H. (2022). Habitability is binary, but it is used by astrobiologists to encompass continuous ecological questions. Astrobiology 22, 7–13. doi:10.1089/ast.2021.0038
Colón-Santos, S., Vázquez-Salazar, A., Adams, A., Campillo-Balderas, J. A., Hernández-Morales, R., Jácome, R., et al. (2024). Chapter 2: what is life? Astrobiology 24, S-40–S-56. doi:10.1089/ast.2021.0116
Cowan, N. B., Agol, E., Meadows, V. S., Robinson, T., Livengood, T. A., Deming, D., et al. (2009). Alien maps of an ocean-bearing world. Astrophysical J. 700, 915–923. doi:10.1088/0004-637x/700/2/915
Damer, B., and Deamer, D. (2020). The hot spring hypothesis for an origin of life. Astrobiology 20, 429–452. doi:10.1089/ast.2019.2045
Dartnell, L. R. (2011). Ionizing radiation and life. Astrobiology 11, 551–582. doi:10.1089/ast.2010.0528
Darwin, C. (1871). Letter no. 7471. Darwin Correspondence Project; University of Cambridge; Available online: https://www.darwinproject.ac.uk/letter/DCP-LETT-7471.xml
Deamer, D., Cary, F., and Damer, B. (2022). Urability: a property of planetary bodies that can support an origin of life. Astrobiology 22, 889–900. doi:10.1089/ast.2021.0173
Deamer, D., and Weber, A. L. (2010). Bioenergetics and life’s origins. Cold Spring Harb. Perspect. Biol. 2, a004929. doi:10.1101/cshperspect.a004929
do Nascimento Vieira, A., Kleinermanns, K., Martin, W. F., and Preiner, M. (2020). The ambivalent role of water at the origins of life. FEBS Lett. 594, 2717–2733. doi:10.1002/1873-3468.13815
Douglas, E. S., Debes, J., Mennesson, B., Nemati, B., Ashcraft, J., Ren, B., et al. (2022). Sensitivity of the roman coronagraph instrument to exozodiacal dust. Publ. Astronomical Soc. Pac. 134, 024402. doi:10.1088/1538-3873/ac3f7b
Dressing, C. D., and Charbonneau, D. (2013). The occurrence rate of small planets around small stars. Astrophysical J. 767, 95. doi:10.1088/0004-637x/767/1/95
Ehlmann, B. L., Anderson, F. S., Andrews-Hanna, J., Catling, D. C., Christensen, P. R., Cohen, B. A., et al. (2016). The sustainability of habitability on terrestrial planets: Insights, questions, and needed measurements from mars for understanding the evolution of earth-like worlds. J. Geophys. Res. Planets 121, 1927–1961. doi:10.1002/2016je005134
Emsenhuber, A., Mordasini, C., Burn, R., Alibert, Y., Benz, W., and Asphaug, E. (2021). The new generation planetary population synthesis (ngpps)-i. bern global model of planet formation and evolution, model tests, and emerging planetary systems. Astronomy and Astrophysics 656, A69. doi:10.1051/0004-6361/202038553
Fabrycky, D. C., Lissauer, J. J., Ragozzine, D., Rowe, J. F., Steffen, J. H., Agol, E., et al. (2014). Architecture of kepler’s multi-transiting systems. ii. new investigations with twice as many candidates. Astrophysical J. 790, 146. doi:10.1088/0004-637x/790/2/146
Feldman, W., Prettyman, T., Maurice, S., Plaut, J., Bish, D., Vaniman, D., et al. (2004). Global distribution of near-surface hydrogen on mars. J. Geophys. Res. Planets 109. doi:10.1029/2003je002160
Fulton, B. J., Petigura, E. A., Howard, A. W., Isaacson, H., Marcy, G. W., Cargile, P. A., et al. (2017). The California-kepler survey. iii. a gap in the radius distribution of small planets. Astronomical J. 154, 109. doi:10.3847/1538-3881/aa80eb
Furukawa, Y., Chikaraishi, Y., Ohkouchi, N., Ogawa, N. O., Glavin, D. P., Dworkin, J. P., et al. (2019). Extraterrestrial ribose and other sugars in primitive meteorites. Proc. Natl. Acad. Sci. 116, 24440–24445. doi:10.1073/pnas.1907169116
Gaidos, E. J., Nealson, K. H., and Kirschvink, J. L. (1999). Life in ice-covered oceans. Science 284, 1631–1633. doi:10.1126/science.284.5420.1631
Garvin, J. B., Getty, S. A., Arney, G. N., Johnson, N. M., Kohler, E., Schwer, K. O., et al. (2022). Revealing the mysteries of venus: the davinci mission. Planet. Sci. J. 3, 117. doi:10.3847/psj/ac63c2
Goldford, J. E., Hartman, H., Smith, T. F., and Segrè, D. (2017). Remnants of an ancient metabolism without phosphate. Cell 168, 1126–1134.e9. doi:10.1016/j.cell.2017.02.001
Grant, S. L., van Dishoeck, E. F., Tabone, B., Gasman, D., Henning, T., Kamp, I., et al. (2023). Minds. the detection of 13co2 with jwst-miri indicates abundant co2 in a protoplanetary disk. Astrophysical J. Lett. 947, L6. doi:10.3847/2041-8213/acc44b
Greene, T. P., Bell, T. J., Ducrot, E., Dyrek, A., Lagage, P.-O., and Fortney, J. J. (2023). Thermal emission from the earth-sized exoplanet trappist-1 b using jwst. Nature 618, 39–42. doi:10.1038/s41586-023-05951-7
Grefenstette, N., Chou, L., Colón-Santos, S., Fisher, T. M., Mierzejewski, V., Nural, C., et al. (2024). Chapter 9: life as we don’t know it. Astrobiology 24 (S–186), S-186–S-201. doi:10.1089/ast.2021.0103
Grießmeier, J.-M., Tabataba-Vakili, F., Stadelmann, A., Grenfell, J., and Atri, D. (2016). Galactic cosmic rays on extrasolar earth-like planets-ii. atmospheric implications. Astronomy and Astrophysics 587, A159. doi:10.1051/0004-6361/201425452
Haas, S., Sinclair, K. P., and Catling, D. C. (2024). Biogeochemical explanations for the world’s most phosphate-rich lake, an origin-of-life analog. Commun. Earth and Environ. 5, 28. doi:10.1038/s43247-023-01192-8
Hand, K. P., Carlson, R. W., and Chyba, C. F. (2007). Energy, chemical disequilibrium, and geological constraints on europa. Astrobiology 7, 1006–1022. doi:10.1089/ast.2007.0156
Hartman, H. (1998). Photosynthesis and the origin of life. Orig. Life Evol. Biosphere 28, 515–521. doi:10.1023/a:1006548904157
Hayes, A. G. (2016). The lakes and seas of titan. Annu. Rev. Earth Planet. Sci. 44, 57–83. doi:10.1146/annurev-earth-060115-012247
Hayes, A. G., Lorenz, R. D., and Lunine, J. I. (2018). A post-cassini view of titan’s methane-based hydrologic cycle. Nat. Geosci. 11, 306–313. doi:10.1038/s41561-018-0103-y
Hendrix, A. R., Hurford, T. A., Barge, L. M., Bland, M. T., Bowman, J. S., Brinckerhoff, W., et al. (2019). The nasa roadmap to ocean worlds. Astrobiology 19, 1–27. doi:10.1089/ast.2018.1955
Herd, C. D., Bosak, T., Hausrath, E. M., Hickman-Lewis, K., Mayhew, L. E., Shuster, D. L., et al. (2025). Sampling mars: geologic context and preliminary characterization of samples collected by the nasa mars 2020 perseverance rover mission. Proc. Natl. Acad. Sci. 122, e2404255121. doi:10.1073/pnas.2404255121
Hoehler, T. M. (2007). An energy balance concept for habitability. Astrobiology 7, 824–838. doi:10.1089/ast.2006.0095
Hubbard, G. S., Naderi, F. M., and Garvin, J. B. (2002). Following the water, the new program for mars exploration. Acta Astronaut. 51, 337–350. doi:10.1016/s0094-5765(02)00067-x
Jia, X., Kivelson, M. G., Khurana, K. K., and Kurth, W. S. (2018). Evidence of a plume on europa from galileo magnetic and plasma wave signatures. Nat. Astron. 2, 459–464. doi:10.1038/s41550-018-0450-z
Kane, S. R., Arney, G., Crisp, D., Domagal-Goldman, S., Glaze, L. S., Goldblatt, C., et al. (2019). Venus as a laboratory for exoplanetary science. J. Geophys. Res. Planets 124, 2015–2028. doi:10.1029/2019je005939
Kaplan, H. H., Simon, A. A., Hamilton, V. E., Thompson, M., Sandford, S. A., Barucci, M. A., et al. (2021). Composition of organics on asteroid (101955) bennu. Astronomy and Astrophysics 653, L1. doi:10.1051/0004-6361/202141167
Kasting, J. F., Whitmire, D. P., and Reynolds, R. T. (1993). Habitable zones around main sequence stars. Icarus 101, 108–128. doi:10.1006/icar.1993.1010
Kelley, D. S., Karson, J. A., Blackman, D. K., FruÈh-Green, G. L., Butterfield, D. A., Lilley, M. D., et al. (2001). An off-axis hydrothermal vent field near the Mid-Atlantic Ridge at 30° N. Nature 412, 145–149. doi:10.1038/35084000
Kiang, N. Y., Segura, A., Tinetti, G., Blankenship, R. E., Cohen, M., Siefert, J., et al. (2007a). Spectral signatures of photosynthesis. ii. coevolution with other stars and the atmosphere on extrasolar worlds. Astrobiology 7, 252–274. doi:10.1089/ast.2006.0108
Kiang, N. Y., Siefert, J., and Blankenship, R. E. (2007b). Spectral signatures of photosynthesis. i. review of earth organisms. Astrobiology 7, 222–251. doi:10.1089/ast.2006.0105
Kite, E. S., and Ford, E. B. (2018). Habitability of exoplanet waterworlds. Astrophysical J. 864, 75. doi:10.3847/1538-4357/aad6e0
Kivelson, M. G., Khurana, K. K., Russell, C. T., Volwerk, M., Walker, R. J., and Zimmer, C. (2000). Galileo magnetometer measurements: a stronger case for a subsurface ocean at europa. Science 289, 1340–1343. doi:10.1126/science.289.5483.1340
Kolb, V. M., and Clark III, B. C. (2020). Astrobiology for a general reader: a questions and answers approach. Newcastle, UK: Cambridge Schloars Publishing.
Kotsyurbenko, O. (2023). Searching for life on venus: history of the problem and basic concepts. Sol. Syst. Res. 57, 232–247. doi:10.31857/s0320930x23030052
Kunieda, H., Nakamura, K., and Evans, D. F. (1991). Formation of reversed vesicles. J. Am. Chem. Soc. 113, 1051–1052. doi:10.1021/ja00003a054
Lane, N., and Xavier, J. C. (2024). To unravel the origin of life, treat findings as pieces of a bigger puzzle. Nature 626, 948–951. doi:10.1038/d41586-024-00544-4
Lazcano, A. (2016). Alexandr i. oparin and the origin of life: a historical reassessment of the heterotrophic theory. J. Mol. Evol. 83, 214–222. doi:10.1007/s00239-016-9773-5
Lazcano, A., and Miller, S. L. (1996). The origin and early evolution of life: prebiotic chemistry, the pre-rna world, and time. Cell 85, 793–798. doi:10.1016/s0092-8674(00)81263-5
Lazcano, A., and Miller, S. L. (1999). On the origin of metabolic pathways. J. Mol. Evol. 49, 424–431. doi:10.1007/pl00006565
Leung, M., Schwieterman, E. W., Parenteau, M. N., and Fauchez, T. J. (2022). Alternative methylated biosignatures. i. methyl bromide, a capstone biosignature. Astrophysical J. 938 (6), 6. doi:10.3847/1538-4357/ac8799
Liu, Z., Wu, L.-F., Kufner, C. L., Sasselov, D. D., Fischer, W. W., and Sutherland, J. D. (2021). Prebiotic photoredox synthesis from carbon dioxide and sulfite. Nat. Chem. 13, 1126–1132. doi:10.1038/s41557-021-00789-w
Longo, A., and Damer, B. (2020). Factoring origin of life hypotheses into the search for life in the solar system and beyond. Life 10, 52. doi:10.3390/life10050052
López-Morales, M., Currie, T., Teske, J., Gaidos, E., Kempton, E., Males, J., et al. (2019). Detecting earth-like biosignatures on rocky exoplanets around nearby stars with ground-based extremely large telescopes. arXiv preprint arXiv:1903.09523
MacKenzie, S. M., Birch, S. P., Hörst, S., Sotin, C., Barth, E., Lora, J. M., et al. (2021). Titan: earth-like on the outside, ocean world on the inside. Planet. Sci. J. 2, 112. doi:10.3847/psj/abf7c9
Madhusudhan, N. (2024). The hycean paradigm in the search for life elsewhere. arXiv preprint arXiv:2406.12794
Madhusudhan, N., Piette, A. A., and Constantinou, S. (2021). Habitability and biosignatures of hycean worlds. Astrophysical J. 918 (1), 1. doi:10.3847/1538-4357/abfd9c
Madhusudhan, N., Sarkar, S., Constantinou, S., Holmberg, M., Piette, A. A., and Moses, J. I. (2023). Carbon-bearing molecules in a possible hycean atmosphere. Astrophysical J. Lett. 956, L13. doi:10.3847/2041-8213/acf577
Mamajek, E., and Stapelfeldt, K. (2024). Nasa exoplanet exploration program (exep) mission star list for the habitable worlds observatory (2023). arXiv preprint arXiv:2402.12414
Martin, W., Baross, J., Kelley, D., and Russell, M. J. (2008). Hydrothermal vents and the origin of life. Nat. Rev. Microbiol. 6, 805–814. doi:10.1038/nrmicro1991
Martins, Z., Botta, O., Fogel, M. L., Sephton, M. A., Glavin, D. P., Watson, J. S., et al. (2008). Extraterrestrial nucleobases in the murchison meteorite. Earth Planet. Sci. Lett. 270, 130–136. doi:10.1016/j.epsl.2008.03.026
Mayor, M., and Queloz, D. (1995). A jupiter-mass companion to a solar-type star. nature 378, 355–359. doi:10.1038/378355a0
McCollom, T. M. (2006). “The habitability of mars: past and present,” in Solar system update (Springer), 159–175.
McCollom, T. M., and Seewald, J. S. (2013). Serpentinites, hydrogen, and life. Elements 9, 129–134. doi:10.2113/gselements.9.2.129
Meadows, V., Graham, H., Abrahamsson, V., Adam, Z., Amador-French, E., Arney, G., et al. (2022). Community report from the biosignatures standards of evidence workshop. arXiv preprint arXiv:2210.14293
Miller, S. L. (1953). A production of amino acids under possible primitive earth conditions. Science 117, 528–529. doi:10.1126/science.117.3046.528
Miller, S. L. (1955). Production of some organic compounds under possible primitive earth conditions1. J. Am. Chem. Soc. 77, 2351–2361. doi:10.1021/ja01614a001
NASA (2015). Astrobiology strategy 2015. National Aeronautics and Space Administration. Available online at: https://astrobiology.nasa.gov/uploads/filer_public/01/28/01283266-e401-4dcb-8e05-3918b21edb79/nasa_astrobiology_strategy_2015_151008.pdf
NASEM (2010). New worlds, new horizons in Astronomy and astrophysics. Washington, DC: The National Academies Press. doi:10.17226/12951
NASEM (2011). Vision and voyages for planetary science in the decade 2013-2022. Washington, DC: The National Academies Press. doi:10.17226/13117
NASEM (2018). Exoplanet science strategy. Washington, DC: The National Academies Press. doi:10.17226/25187
NASEM (2019). An astrobiology strategy for the search for life in the universe. Washington, DC: The National Academies Press. doi:10.17226/25252
NASEM (2023a). Origins, worlds, and life: a decadal strategy for planetary science and astrobiology 2023-2032. Washington, DC: The National Academies Press. doi:10.17226/26522
NASEM (2023b). Pathways to discovery in Astronomy and astrophysics for the 2020s. Washington, DC: The National Academies Press. doi:10.17226/26141
Neveu, M., Hays, L. E., Voytek, M. A., New, M. H., and Schulte, M. D. (2018). The ladder of life detection. Astrobiology 18, 1375–1402. doi:10.1089/ast.2017.1773
Nimmo, F., and Pappalardo, R. T. (2016). Ocean worlds in the outer solar system. J. Geophys. Res. Planets 121, 1378–1399. doi:10.1002/2016je005081
Nowicka, B., and Kruk, J. (2016). Powered by light: phototrophy and photosynthesis in prokaryotes and its evolution. Microbiol. Res. 186, 99–118. doi:10.1016/j.micres.2016.04.001
Ostberg, C., Kane, S. R., Li, Z., Schwieterman, E. W., Hill, M. L., Bott, K., et al. (2023a). The demographics of terrestrial planets in the venus zone. Astronomical J. 165, 168. doi:10.3847/1538-3881/acbfaf
Ostberg, C. M., Guzewich, S. D., Kane, S. R., Kohler, E., Oman, L. D., Fauchez, T. J., et al. (2023b). The prospect of detecting volcanic signatures on an exoearth using direct imaging. Astronomical J. 166, 199. doi:10.3847/1538-3881/acfe12
Pappalardo, R. T., Buratti, B. J., Korth, H., Senske, D. A., Blaney, D. L., Blankenship, D. D., et al. (2024). Science overview of the europa clipper mission. Space Sci. Rev. 220, 40–58. doi:10.1007/s11214-024-01070-5
Parkinson, C. D., Liang, M.-C., Hartman, H., Hansen, C. J., Tinetti, G., Meadows, V., et al. (2007). Enceladus: cassini observations and implications for the search for life. Astronomy and Astrophysics 463, 353–357. doi:10.1051/0004-6361:20065773
Pearce, B. K., Molaverdikhani, K., Pudritz, R. E., Henning, T., and Cerrillo, K. E. (2022). Toward rna life on early earth: from atmospheric hcn to biomolecule production in warm little ponds. Astrophysical J. 932 (9), 9. doi:10.3847/1538-4357/ac47a1
Petigura, E. A., Howard, A. W., and Marcy, G. W. (2013). Prevalence of earth-size planets orbiting sun-like stars. Proc. Natl. Acad. Sci. 110, 19273–19278. doi:10.1073/pnas.1319909110
Pizzarello, S., and Shock, E. (2010). The organic composition of carbonaceous meteorites: the evolutionary story ahead of biochemistry. Cold Spring Harb. Perspect. Biol. 2, a002105. doi:10.1101/cshperspect.a002105
Pohorille, A., and Pratt, L. R. (2012). Is water the universal solvent for life? Orig. Life Evol. Biospheres 42, 405–409. doi:10.1007/s11084-012-9301-6
Pontoppidan, K. M., Salyk, C., Bergin, E. A., Brittain, S., Marty, B., Mousis, O., et al. (2014). Volatiles in protoplanetary disks. Protostars and Planets VI University of Arizona Press in collaboration with the Lunar and Planetary Institute, Tuscon, Arizona USA, 363–385.
Postberg, F., Khawaja, N., Abel, B., Choblet, G., Glein, C. R., Gudipati, M. S., et al. (2018). Macromolecular organic compounds from the depths of enceladus. Nature 558, 564–568. doi:10.1038/s41586-018-0246-4
Postberg, F., Sekine, Y., Klenner, F., Glein, C. R., Zou, Z., Abel, B., et al. (2023). Detection of phosphates originating from enceladus’s ocean. Nature 618, 489–493. doi:10.1038/s41586-023-05987-9
Powner, M. W., Gerland, B., and Sutherland, J. D. (2009). Synthesis of activated pyrimidine ribonucleotides in prebiotically plausible conditions. Nature 459, 239–242. doi:10.1038/nature08013
Powner, M. W., Sutherland, J. D., and Szostak, J. W. (2011). The origins of nucleotides. Synlett 22, 1956–1964. doi:10.1055/s-0030-1261177
Preiner, M., Igarashi, K., Muchowska, K. B., Yu, M., Varma, S. J., Kleinermanns, K., et al. (2020). A hydrogen-dependent geochemical analogue of primordial carbon and energy metabolism. Nat. Ecol. and Evol. 4, 534–542. doi:10.1038/s41559-020-1125-6
Purvis, G., Šiller, L., Crosskey, A., Vincent, J., Wills, C., Sheriff, J., et al. (2024). Generation of long-chain fatty acids by hydrogen-driven bicarbonate reduction in ancient alkaline hydrothermal vents. Commun. Earth and Environ. 5, 30. doi:10.1038/s43247-023-01196-4
Quick, L. C., Roberge, A., Mlinar, A. B., and Hedman, M. M. (2020). Forecasting rates of volcanic activity on terrestrial exoplanets and implications for cryovolcanic activity on extrasolar ocean worlds. Publ. Astronomical Soc. Pac. 132, 084402. doi:10.1088/1538-3873/ab9504
Ranjan, S., Wordsworth, R., and Sasselov, D. D. (2017). The surface uv environment on planets orbiting m dwarfs: implications for prebiotic chemistry and the need for experimental follow-up. Astrophysical J. 843, 110. doi:10.3847/1538-4357/aa773e
Rimmer, P. B., Ranjan, S., and Rugheimer, S. (2021). Life’s origins and the search for life on rocky exoplanets. Elem. Int. Mag. Mineralogy, Geochem. Petrology 17, 265–270. doi:10.2138/gselements.17.4.265
Ritson, D. J., Battilocchio, C., Ley, S. V., and Sutherland, J. D. (2018). Mimicking the surface and prebiotic chemistry of early earth using flow chemistry. Nat. Commun. 9, 1821. doi:10.1038/s41467-018-04147-2
Ritson, D. J., and Sutherland, J. D. (2013). Synthesis of aldehydic ribonucleotide and amino acid precursors by photoredox chemistry. Angewandte Chemie Int. Ed. Engl. 52, 5845–5847. doi:10.1002/anie.201300321
Robinson, T. D. (2018). Characterizing exoplanet habitability. Springer International Publishing, 3137–3157. doi:10.1007/978-3-319-55333-7_67
Robinson, T. D., Meadows, V. S., and Crisp, D. (2010). Detecting oceans on extrasolar planets using the glint effect. Astrophysical J. 721, L67–L71. doi:10.1088/2041-8205/721/1/l67
Rodriguez, L. E., Altair, T., Hermis, N. Y., Jia, T. Z., Roche, T. P., Steller, L. H., et al. (2024a). Chapter 4: a geological and chemical context for the origins of life on early earth. Astrobiology 24, S-76–S-106. doi:10.1089/ast.2021.0139
Rodriguez, L. E., Weber, J. M., and Barge, L. M. (2024b). Evaluating pigments as a biosignature: abiotic/prebiotic synthesis of pigments and pigment mimics in planetary environments. Astrobiology 24, 767–782. doi:10.1089/ast.2023.0006
Roettenbacher, R. M., and Kane, S. R. (2017). The stellar activity of trappist-1 and consequences for the planetary atmospheres. Astrophysical J. 851, 77. doi:10.3847/1538-4357/aa991e
Russell, M. J. (2007). The alkaline solution to the emergence of life: energy, entropy and early evolution. Acta biotheor. 55, 133–179. doi:10.1007/s10441-007-9018-5
Russell, M. J., Hall, A. J., and Martin, W. (2010). Serpentinization as a source of energy at the origin of life. Geobiology 8, 355–371. doi:10.1111/j.1472-4669.2010.00249.x
Russell, M. J., and Martin, W. (2004). The rocky roots of the acetyl-coa pathway. Trends Biochem. Sci. 29, 358–363. doi:10.1016/j.tibs.2004.05.007
Schaible, M. J., Szeinbaum, N., Bozdag, G. O., Chou, L., Grefenstette, N., Colón-Santos, S., et al. (2024a). Chapter 1: the astrobiology primer 3.0. Astrobiology 24, S-4–S-39. doi:10.1089/ast.2021.0129
Schaible, M. J., Todd, Z. R., Cangi, E. M., Harman, C. E., Hughson, K. H., and Stelmach, K. (2024b). Chapter 3: the origins and evolution of planetary systems. Astrobiology 24, S-57–S-75. doi:10.1089/ast.2021.0127
Scharf, C. (2009). Extrasolar Planets and astrobiology. G - reference, Information and interdisciplinary subjects series. California USA: University Science Books Sausalito.
Scharf, C., and Cronin, L. (2016). Quantifying the origins of life on a planetary scale. Proc. Natl. Acad. Sci. 113, 8127–8132. doi:10.1073/pnas.1523233113
Scharf, C., Virgo, N., Cleaves, H. J., Aono, M., Aubert-Kato, N., Aydinoglu, A., et al. (2015). A strategy for origins of life research. Astrobiology 15, 1031–1042. doi:10.1089/ast.2015.1113
Schönheit, P., Buckel, W., and Martin, W. F. (2016). On the origin of heterotrophy. Trends Microbiol. 24, 12–25. doi:10.1016/j.tim.2015.10.003
Schwieterman, E. W., Kiang, N. Y., Parenteau, M. N., Harman, C. E., DasSarma, S., Fisher, T. M., et al. (2018). Exoplanet biosignatures: a review of remotely detectable signs of life. Astrobiology 18, 663–708. doi:10.1089/ast.2017.1729
Smrekar, S., Hensley, S., Nybakken, R., Wallace, M. S., Perkovic-Martin, D., You, T.-H., et al. (2022). “Veritas (venus emissivity, radio science, insar, topography, and spectroscopy): a discovery mission,” in 2022 IEEE aerospace conference (AERO) (IEEE), 1–20.
Soffen, G. A. (1976). Scientific results of the viking missions. Science 194, 1274–1276. doi:10.1126/science.194.4271.1274
Sparks, W. B., Hand, K., McGrath, M., Bergeron, E., Cracraft, M., and Deustua, S. (2016). Probing for evidence of plumes on europa with hst/stis. Astrophysical J. 829, 121. doi:10.3847/0004-637x/829/2/121
Staplefelt, K., and Mamajek, E. (2023). Exoplanet exploration program science gap list 2023. Available online at: https://exoplanets.nasa.gov/internal_resources/2749/ExEP_Science_Gap_List_2023_Final.pdf
Stark, C. C., Mennesson, B., Bryson, S., Ford, E. B., Robinson, T. D., Belikov, R., et al. (2024). Paths to robust exoplanet science yield margin for the habitable worlds observatory. J. Astron. Telesc. Instrum. Syst. 10. arXiv preprint arXiv:2405.19418. doi:10.1117/1.jatis.10.3.034006
Stephan, K., Jaumann, R., Brown, R. H., Soderblom, J. M., Soderblom, L. A., Barnes, J. W., et al. (2010). Specular reflection on titan: liquids in kraken mare. Geophys. Res. Lett. 37. doi:10.1029/2009gl042312
Styczinski, M., Cooper, Z., Glaser, D., Lehmer, O., Mierzejewski, V., and Tarnas, J. (2024). Chapter 7: assessing habitability beyond earth. Astrobiology 24, S-143–S-163. doi:10.1089/ast.2021.0097
Sutherland, J. D. (2016). The origin of life—out of the blue. Angew. Chem. Int. Ed. 55, 104–121. doi:10.1002/anie.201506585
Szostak, J. W. (2017). The origin of life on earth and the design of alternative life forms. Mol. Front. J. 1, 121–131. doi:10.1142/s2529732517400132
Tarnas, J. D., Mustard, J. F., Sherwood Lollar, B., Stamenković, V., Cannon, K., Lorand, J.-P., et al. (2021). Earth-like habitable environments in the subsurface of mars. Astrobiology 21, 741–756. doi:10.1089/ast.2020.2386
Thweatt, J. L., Harman, C., Araújo, M., Marlow, J. J., Oliver, G. C., Sabuda, M. C., et al. (2024). Chapter 6: the breadth and limits of life on earth. Astrobiology 24, S-124–S-142. doi:10.1089/ast.2021.0131
Tirard, S. (2017). Jbs haldane and the origin of life. J. Genet. 96, 735–739. doi:10.1007/s12041-017-0831-6
Toner, J. D., and Catling, D. C. (2019). Alkaline lake settings for concentrated prebiotic cyanide and the origin of life. Geochimica Cosmochimica Acta 260, 124–132. doi:10.1016/j.gca.2019.06.031
Toner, J. D., and Catling, D. C. (2020). A carbonate-rich lake solution to the phosphate problem of the origin of life. Proc. Natl. Acad. Sci. 117, 883–888. doi:10.1073/pnas.1916109117
Trainer, M. G., Pavlov, A. A., DeWitt, H. L., Jimenez, J. L., McKay, C. P., Toon, O. B., et al. (2006). Organic haze on titan and the early earth. Proc. Natl. Acad. Sci. 103, 18035–18042. doi:10.1073/pnas.0608561103
Tsai, S.-M., Lee, E. K., Powell, D., Gao, P., Zhang, X., Moses, J., et al. (2023). Photochemically produced so2 in the atmosphere of wasp-39b. Nature 617, 483–487. doi:10.1038/s41586-023-05902-2
Tuchow, N. W., Stark, C. C., and Mamajek, E. (2024). Hpic: the habitable worlds observatory preliminary input catalog. Astronomical J. 167, 139. doi:10.3847/1538-3881/ad25ec
Vaughan, S. R., Gebhard, T. D., Bott, K., Casewell, S. L., Cowan, N. B., Doelman, D. S., et al. (2023). Chasing rainbows and ocean glints: inner working angle constraints for the habitable worlds observatory. Mon. Notices R. Astronomical Soc. 524, 5477–5485. doi:10.1093/mnras/stad2127
Wächtershäuser, G. (1990). The case for the chemoautotrophic origin of life in an iron-sulfur world. Orig. Life Evol. Biosphere 20, 173–176. doi:10.1007/bf01808279
Waite, J. H., Glein, C. R., Perryman, R. S., Teolis, B. D., Magee, B. A., Miller, G., et al. (2017). Cassini finds molecular hydrogen in the enceladus plume: evidence for hydrothermal processes. Science 356, 155–159. doi:10.1126/science.aai8703
Wang, J., Mawet, D., Ruane, G., Hu, R., and Benneke, B. (2017). Observing exoplanets with high dispersion coronagraphy. i. the scientific potential of current and next-generation large ground and space telescopes. Astronomical J. 153, 183. doi:10.3847/1538-3881/aa6474
Wang, J. J., Ruffio, J.-B., Morris, E., Delorme, J.-R., Jovanovic, N., Pezzato, J., et al. (2021). Detection and bulk properties of the hr 8799 planets with high-resolution spectroscopy. Astronomical J. 162, 148. doi:10.3847/1538-3881/ac1349
Weber, J. M., Marlin, T. C., Prakash, M., Teece, B. L., Dzurilla, K., and Barge, L. M. (2023). A review on hypothesized metabolic pathways on europa and enceladus: space-flight detection considerations. Life 13, 1726. doi:10.3390/life13081726
Weiss, M. C., Sousa, F. L., Mrnjavac, N., Neukirchen, S., Roettger, M., Nelson-Sathi, S., et al. (2016). The physiology and habitat of the last universal common ancestor. Nat. Microbiol. 1, 16116–16118. doi:10.1038/nmicrobiol.2016.116
Westall, F., Loizeau, D., Foucher, F., Bost, N., Betrand, M., Vago, J., et al. (2013). Habitability on mars from a microbial point of view. Astrobiology 13, 887–897. doi:10.1089/ast.2013.1000
Woese, C. R. (1979). A proposal concerning the origin of life on the planet earth. J. Mol. Evol. 13, 95–101. doi:10.1007/bf01732865
Wolszczan, A., and Frail, D. A. (1992). A planetary system around the millisecond pulsar psr1257+ 12. Nature 355, 145–147. doi:10.1038/355145a0
Wong, M. L., Bartlett, S., Chen, S., and Tierney, L. (2022). Searching for life, mindful of lyfe’s possibilities. Life 12, 783. doi:10.3390/life12060783
Yee, S. W., Winn, J. N., Hartman, J. D., Rodriguez, J. E., Zhou, G., Quinn, S. N., et al. (2022). The tess grand unified hot jupiter survey. i. ten tess planets. Astronomical J. 164, 70. doi:10.3847/1538-3881/ac73ff
Yen, H.-W., Koch, P. M., Takakuwa, S., Krasnopolsky, R., Ohashi, N., and Aso, Y. (2017). Signs of early-stage disk growth revealed with alma. Astrophysical J. 834, 178. doi:10.3847/1538-4357/834/2/178
Zieba, S., Kreidberg, L., Ducrot, E., Gillon, M., Morley, C., Schaefer, L., et al. (2023). No thick carbon dioxide atmosphere on the rocky exoplanet trappist-1 c. Nature 620, 746–749. doi:10.1038/s41586-023-06232-z
Keywords: exoplanets, origin of life, habitability, biosignatures, life detection
Citation: Keller F, Kataria T, Barge LM, Chen P, Yung Y and Weber JM (2025) An exploration of origin of life for exoplanetary science. Front. Astron. Space Sci. 12:1544426. doi: 10.3389/fspas.2025.1544426
Received: 12 December 2024; Accepted: 25 February 2025;
Published: 19 March 2025.
Edited by:
Long Xiao, China University of Geosciences Wuhan, ChinaReviewed by:
Siteng Fan, Sorbonne Université (CNRS), FranceCopyright © 2025 Keller, Kataria, Barge, Chen, Yung and Weber. This is an open-access article distributed under the terms of the Creative Commons Attribution License (CC BY). The use, distribution or reproduction in other forums is permitted, provided the original author(s) and the copyright owner(s) are credited and that the original publication in this journal is cited, in accordance with accepted academic practice. No use, distribution or reproduction is permitted which does not comply with these terms.
*Correspondence: Jessica M. Weber, amVzc2ljYS53ZWJlckBqcGwubmFzYS5nb3Y=
Disclaimer: All claims expressed in this article are solely those of the authors and do not necessarily represent those of their affiliated organizations, or those of the publisher, the editors and the reviewers. Any product that may be evaluated in this article or claim that may be made by its manufacturer is not guaranteed or endorsed by the publisher.
Research integrity at Frontiers
Learn more about the work of our research integrity team to safeguard the quality of each article we publish.