- 1PHaSER, Department of Astronomy, University of Maryland, College Park, College Park, MD, United States
- 2NASA Goddard Space Flight Center, Greenbelt, MD, United States
- 3Space Research Institute, Austrian Academy of Sciences, Graz, Styria, Austria
- 4University of Graz, Institute of Physics, Graz, Styria, Austria
- 5Department of Electrical and Computer Engineering, University of New Mexico, Albuquerque, NM, United States
- 6Air Force Research Laboratory, Kirtland AFB, NM, United States
- 7Applied Research Laboratory, Johns Hopkins University, Laurel, MD, United States
- 8Department of Physical Sciences, Embry-Riddle Aeronautical University, Daytona Beach, FL, United States
The Kelvin-Helmholtz Instability (KHI) is a large scale convective instability which occurs anywhere the velocity shear between two fluids is large, such as Earth’s magnetopause where the fast flowing magnetosheath abuts the relatively stagnant outer magnetosphere. The KHI was initially believed to contribute only to energy and momentum transfer from the solar wind to the magnetosphere, but was eventually shown to support mass transport and plasma heating. Recent advancements in in-situ observational capabilities and high scale computer modeling have once again shifted our understanding of the KHI from a large scale process, to an active environment which connects the global and kinetic scales through a variety of multi-scale processes and phenomena. In this mini-review, we provide an update on the latest findings in Kelvin-Helmholtz (KH) related processes at kinetic scales and the effects of the global environment on KH development.
1 Introduction
The solar wind must supply energy to the magnetosphere at a rate of
In the last few decades, it has been shown that the KHI is not only responsible for viscous momentum transfer (Miura, 1987), but supports myriad secondary processes down to kinetic scales including reconnection, ion and electron scale plasma waves, and plasma turbulence. These secondary processes can further enhance mixing and heating and in some cases, interact with or drive each other. Recent observations and simulations have also shown that the fluid scale KHI impacts and is impacted by global scale patterns within the magnetosphere and heliosphere. In this mini-review, we provide an overview of the most recent findings which inform our current understanding of the KHI as a phenomena connecting physics across multiple scale sizes.
2 The KHI and kinetic scale processes
Though initially believed to be responsible only for momentum and energy transfer to the magnetosphere (Miura, 1987), it is now known that the KHI is also able to drive plasma transport and heating via kinetic scale processes such as reconnection, diffusive transport, and kinetic scale wave modes. Recent technological advances in in-situ measurements (Burch et al., 2016a; Angelopoulos, 2008; Escoubet et al., 2001) and the accessibility of petascale kinetic simulations (Bowers et al., 2009), have enabled numerous studies resolving the nature of kinetic scale processes associated with the KHI. In this section we review a few of these phenomena.
2.1 KHI induced reconnection dynamics
As magnetic reconnection requires a thin current sheet, on the order of the ion inertial length, the inertia of the Kelvin-Helmholtz (KH) driven vortex motion can provide a powerful external driver for reconnection to occur. An example of one such current sheet driven by KH vortex motion is shown in Figure 1. KHI driven reconnection signatures have been detected by multiple spacecraft missions at both low and high-latitudes (Nykyri et al., 2003b; 2006b; Eriksson et al., 2016; Li et al., 2016; Burkholder et al., 2020b; Li et al., 2013).
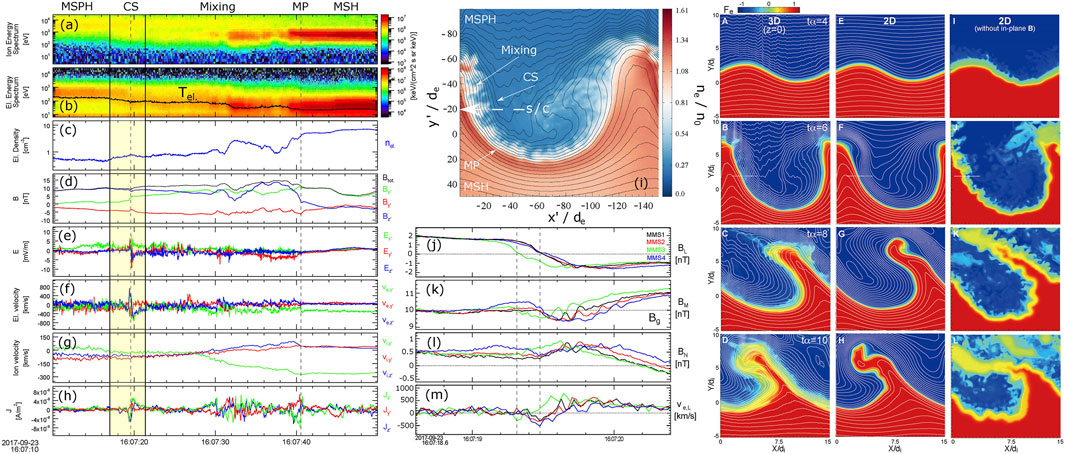
Figure 1. Kinetic scale effects of the KHI. Left, MMS1 observations of the spine region of a KH vortex with a kinetic scale current sheet (yellow shade) observed on 23 September 2017: ion (A) and electron energy spectrogram with overplotted electron temperature (B); electron density (C), magnetic field (including
The importance of KHI to reconnection was first recognized by Otto and Fairfield (2000), who compared boundary layer signatures observed by the Geotail spacecraft (Fairfield et al., 2000) during strongly northward interplanetary magnetic field (IMF) with two dimensional (2D) magnetohydrodynamic (MHD) simulations. Those simulations showed that KHI was able to grow to non-linear stages and twist the magnetic fields into an anti-parallel configuration when the IMF and geomagnetic fields were initially parallel along the shear flow direction and the Alfvén speed along the wave vector,
In three dimensions (3D), magnetic reconnection also occurs above and below the shear flow plane leading to a higher transport rate,
When magnetic fields are initially anti-parallel across the shear flow plane, magnetic reconnection can occur in both the high and low filaments of the vortex, which results in the mixing and capture of low density magnetospheric material into the magnetosheath (Nykyri et al., 2006b; Eriksson et al., 2016). In the case of a symmetric plasma density across the shear flow layer, the direction of plasma transport is determined by the strength of the current density in either spine (Nykyri and Otto, 2004).
The 3D dynamics of magnetic reconnection driven by the KHI during southward IMF have been associated with Alfvénic plasma jets propagating perpendicular to the initial shear flow plane and the escape of magnetospheric electrons into the magnetosheath (Li et al., 2023). Simulations of this geometry have shown that the KHI can increase the reconnection rate even when the Hall term is switched off (Ma et al., 2014a; b).
Reconnection in KH vortices often leads to the mixing of plasmas with different energies and densities which can lead to anisotropic velocity distribution functions (Nykyri et al., 2006b; Moore et al., 2016) and the generation of ion and electron scale plasma waves which can in turn lead to ion and electron heating (Moore et al., 2016; 2017; Nykyri et al., 2021b). The KHI can also effectively drive plasma turbulence to further enhance plasma heating (Stawarz et al., 2016; Delamere et al., 2021). Thus, the efficiency of plasma transport due to reconnection within KHI should not be considered in isolation with regard to other kinetic scale processes, as will be discussed in the following sections.
2.2 Diffusive transport within the KHI
The large-scale KHI has been found to drive secondary instabilities like the Rayleigh-Taylor instability (Nakamura et al., 2022a) and/or secondary KHI (Matsumoto and Hoshino, 2004; Nakamura et al., 2004; Faganello et al., 2008) which interfere with the large-scale evolution of KH vortices and influence the transport of plasma. Inside fully developed vortices, turbulence (Matsumoto and Hoshino, 2004; Stawarz et al., 2016) and magnetic reconnection (see Sec.2.1) lead to diffusive particle transport. At the spine region of the vortices, magnetic reconnection can strongly influence the diffusive transport within the KHI.
Further, the multi-scale nature of the KHI has been discussed in previous studies focusing on turbulent intermittency and anisotropy related to the KHI (Stawarz et al., 2016), nonlinear wave-particle interactions (Sorriso-Valvo et al., 2019) and the distortions of the ion distribution functions due to kinetic effects (Settino et al., 2020; 2021). More recently, Blasl et al. (2022) and Nakamura et al. (2022a,b) reported the development of the Lower Hybrid Drift Instability (LHDI) and a related thickening of the spine region of KH vortices by diffusive plasma transport during southward IMF from both kinetic simulations and MMS data. Further, Blasl et al. (2023) identified small-scale current sheets and ongoing electron-only reconnection related to this diffusive process, shown in Figure 1. These results highlight the importance of a multi-scale and multi-process approach for future studies of the KHI.
2.3 Wave heating driven by the KHI
The plasma mixing, magnetic field twisting, and strong gradients of density, velocity, and pressure inherent to the KHI can support the growth of wave modes which in turn leads to plasma heating. Several wave modes associated with the KHI are known to drive plasma heating across scale sizes at the magnetopause boundary. At the ion scale the most intensely studied are Kinetic Alfvén waves (KAWs), electromagnetic ion cyclotron (EMIC) waves, and magnetosonic waves.
For example, velocity gradients both parallel and perpendicular to the background magnetic field are known to drive electrostatic and electromagnetic ion cyclotron waves (Nykyri et al., 2003a; 2006a; Peñano and Ganguli, 2002; Kim et al., 2004). Zhang et al. (2017) demonstrated that EMIC waves may also be generated in regions where hot anisotropic plasma overlaps with a separate cold and dense population as is the case in well developed KH vortices where hot magnetospheric plasma is mixed or captured into the magnetosheath.
The strong magnetic field gradients present in the KHI also give rise to Alfvén resonance regions, where the surface wave speed matches the local Alfvén speed. At these regions, surface Alfvén waves mode convert to KAWs (Chaston et al., 2007; Johnson and Cheng, 2001). KAWs energize and demagnetize ions, allowing cross field transport of sheath ions into the magnetosphere where they contribute to boundary layer formation Chaston et al. (2007); Johnson and Cheng (2001). A portion of the KAW electric field is parallel to the background magnetic field, allowing for field aligned electron heating (Hasegawa, 1976; Nykyri et al., 2021b).
Kinetic magnetosonic waves, the kinetic counterpart of fast mode MHD waves, can arise from shell distributions in the ion population, such as those produced by reconnection within KH vortices. Fast mode waves carry energy perpendicularly across field lines and can be triggered by a combination of fast sheath flows and pressure perturbations, which appear at the center of KH vortices. Fast mode and kinetic magnetosonic waves have been shown, both in theory and observation, to effectively heat ions (Lembege et al., 1983; Terasawa and Nambu, 1989; Moore et al., 2016; 2017).
In recent years, advances in in-situ instrumentation have allowed significant progress investigating the effects of KH associated waves above the ion cyclotron frequency. Observations of ion acoustic waves and turbulence within KH vortices were reported shortly after MMS’s launch (Wilder et al., 2016; Stawarz et al., 2016). Lower hybrid waves have also been observed and kinetic simulations indicate they are effectively able to heat the cold sheath plasma as it mixes with the magnetospheric population (Blasl et al., 2022; Blasl et al., 2023).
3 KHI and global scale influence
The KHI is subject to influence by changes in the larger heliospheric environment, such as IMF orientation and seasonal variations. Consider that even though the KHI can and does occur for all IMF orientations (Kavosi and Raeder, 2015; Rice et al., 2022), including southward IMF (Hwang et al., 2012; Blasl et al., 2022; Li et al., 2023), the IMF orientation can influence its occurrence rate and location. Henry et al. (2017) found that during Parker-Spiral IMF, the KHI occurs most frequently at the dawn sector due to smaller magnetic tension (Nykyri, 2013); while at the dusk sector it is detected mostly for strongly northward IMF (Taylor et al., 2012; Henry et al., 2017). The KHI is not restricted to the equatorial plane, and has been detected at high-latitudes close to the exterior cusp (Hwang et al., 2011; Ma et al., 2016; Nykyri et al., 2021a). The KHI can also, in turn, affect global scale processes, such as the development of the magnetospheric plasma sheet. In this section we provide an overview of the global scale processes affecting and affected by the KHI.
3.1 Comparison of plasma transport at the cusps and flanks
During extended periods of northward IMF, solar wind entry into the magnetosphere leads to the formation of a cold, dense plasma sheet (CDPS) (Wing et al., 2014). The CDPS exhibits a characteristic dawn-dusk asymmetry in density and temperature (Wing et al., 2005). In general, the dawnside plasma sheet is denser than the duskside and exhibits a broad thermal distribution while the duskside plasma sheet comprises disjoint hot and cold populations (Nishino et al., 2007). The two primary entry paths of the solar wind into the magnetosphere during northward IMF are the KHI at the low-latitude magnetospheric flanks and double cusp reconnection at high-latitudes.
Only recently has it become feasible for global modeling to directly study the relative importance of cusp and flank entry to the formation of the CDPS and the development of dawn-dusk asymmetry. Sorathia et al. (2019) used a combination of high-resolution global MHD and test particle simulations during a synthetic interval of northward IMF to track the entry of solar wind plasma into the magnetosphere and its acceleration on its way to the central plasma sheet. They found comparable contributions from flank-entering (KHI) and cusp-entering (high-latitude reconnection) plasma, but very different effects on the entering plasma. Flank-entering plasma was largely cold and dawn-dusk symmetric, while cusp-entering plasma was predominantly deflected dawnward and accelerated through its interaction with the dusk-dawn directed high-latitude electric field (Burke et al., 1979). The net impact is that the dawnside plasma contains the cold flank-entering plasma and a wide range of accelerated cusp-entering plasma, consistent with the broad thermal distribution observed, as shown in Figure 2. The duskside plasma consists of cold flank-entering plasma and a high-energy subset of the dawnside cusp-entering plasma that is able to drift to the duskside. In this way, it is found that the observed dawn-dusk thermodynamic asymmetries are a consequence of the combined cusp- and flank-entering populations.
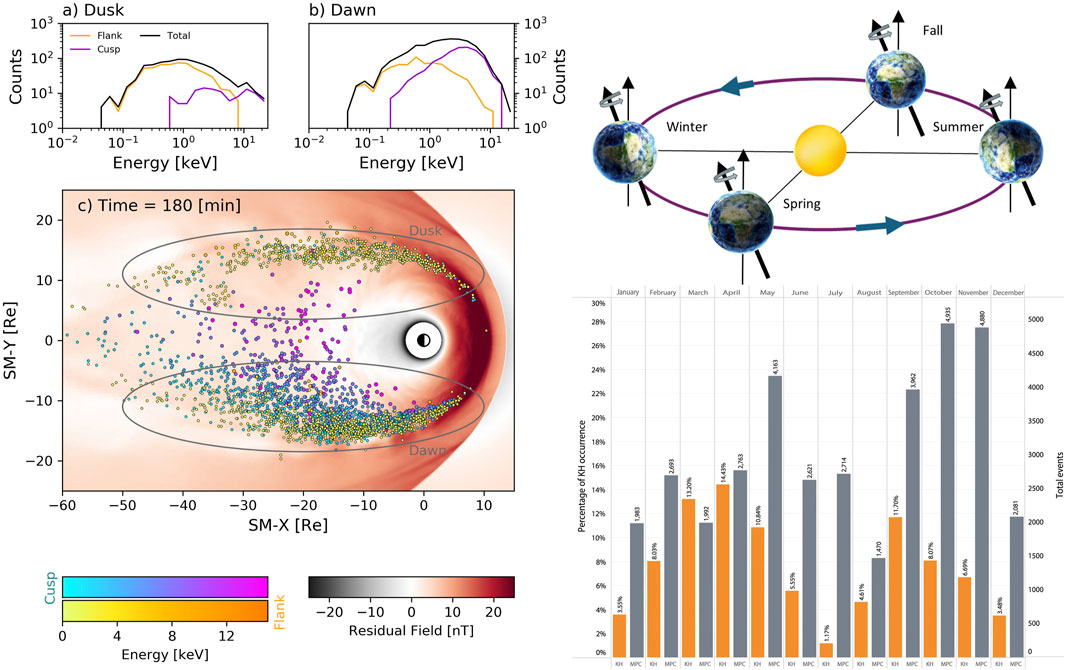
Figure 2. KHI on a Global Scale. At left, from Sorathia et al. (2019), global simulations indicate KHI driven flank entry and reconnection driven cusp entry of solar wind plasma contribute to dawn-dusk asymmetries in the magnetopshere. Energy distributions of test particles that reside in the dusk (A) and dawn (B) flank; and equatorial projections of test particles initialized in the solar wind colored by their energy, using separate color maps based their entry either through the cusp or flank (C). At top right, adapted from Kavosi et al. (2023), the relative orientation of Earth’s rotation axis (black arrow) to the ecliptic plane is subject to seasonal variation. A survey of in situ observations, bottom right, from Kavosi et al. (2023), shows KHI occurrence rate (orange bars) is maximized(minimized) at equinoxes(solstices).
3.2 Dipole tilt effects
New results highlight KHI as an important factor in enhanced geomagnetic activity around the equinox. Kavosi et al. (2023) used data from THEMIS (Angelopoulos, 2008) and MMS (Burch et al., 2016a) over a full solar cycle to investigate the impact of Earth’s dipole orientation on the formation and occurrence of KHI and found that the KHI exhibits seasonal variation. The KHI is more commonly produced during the equinoxes when Earth’s dipole is not tilted toward or away from the Sun, which favors the formation of the KHI at the magnetopause. During solstices, when Earth’s dipole is tilted at extremes toward or away from the Sun, Earth’s magnetic field suppresses KH wave activity (Kavosi et al., 2023).
At Earth’s magnetopause the KHI is primarily driven by a velocity shear aligned with the wave vector,
The tilt of Earth’s dipole axis towards or away from the sun modulates the magnetic tension due to the orientation of the magnetospheric field relative to the shear flow in the GSM-X-Y plane. At equinoxes, the dipole axis is aligned with the GSM-Z axis, perpendicular to the velocity, and the magnetospheric magnetic tension diminishes to 0. Consequently, the dipole field lines exert no stabilizing influence on the KHI and the probability of KHI occurrence increases. At solstices, when the dipole is at its maximum angle relative to the GSM-Z axis, the magnetospheric magnetic tension is also maximized and exerts more stabilizing force on the KHI, which decreases the probability of KHI occurrence. These effects have been confirmed in observations by Kavosi et al. (2023), as shown in Figure 2.
The dominance of the shear flow in the X direction suggests that the magnetosphere’s magnetic tension, influenced by the dipole’s tilt towards or away from the Sun, plays a significant role in stabilizing KHI. Consequently, the equinoctial effect exerts a significant influence on the seasonal and daily variations of KHI, as corroborated by KHI occurrence rate analysis in Kavosi et al. (2023).
4 Discussion and conclusion
As recounted here, recent advancements in observational and computational capabilities have expanded our understanding of the KHI as an active environment which influences and is influenced by processes across scales sizes. At kinetic scales, reconnection, diffusive transport, and wave activity contribute to enhanced plasma transport and heating. These small scale processes can also interrupt the development of KH vortices from linear to non-linear and rolled up stages. At global scales, the location and occurrence of KHI is modulated by IMF orientation and seasonal dipole tilt effects. The KHI contributes to the formation of the CDPS during northward IMF and its pronounced dawn-dusk asymmetry. Overall, the results summarized here paint a picture of the magnetopause not as a static boundary that is merely traversed by solar wind plasma, but as an active participant in the transfer of mass between the magnetosphere and solar wind, a process in which the KHI plays a significant role.
Questions still remain about the nature and development of the KHI. What are the origins of energetic particles within the KHI? How might geomagetic storm conditions affect the development of the KHI? What effects might heavy ion species have on the behavior of the KHI? What influence does the KHI exert throughout the heliosphere, such as at the edges of coronal mass ejections, co-rotating interaction regions, or other planetary magnetospheres? Evidence of KH activity has been observed at Mercury (Paral and Rankin, 2013), Mars (Ruhunusiri et al., 2016; Poh et al., 2021; Wang et al., 2022), Saturn (Ma et al., 2015; Dialynas, 2018; Burkholder et al., 2020a), and Jupiter (Ranquist et al., 2019; Montgomery et al., 2023), but it is not yet known how the scale size of the planetary magnetosphere affects the multi-scale nature of the KHI.
The above questions and our new understanding of the KHI as a multi-scale process should inform the direction of future research. New studies on the diffusive transport within the KH vortices should consider the multi-scale and multi-effect nature of the KHI rather than focusing on single effects and scales. Efforts should be made to relate the KHI to global parameters, such as the solar wind conditions and season, in order to obtain a global picture of the instability, its influences, and the implications for global solar wind transport. Simulation studies should consider the role of more realistic solar wind IMF orientations or the role of kinetic physics (e.g., Ma et al., 2019) in KHI development and its effects. Space weather forecasters may incorporate dipole tilt effects on KHI into their models, resulting in more accurate and reliable forecasting.
Author contributions
RR: Conceptualization, Writing–original draft, Writing–review and editing. KB: Writing–original draft, Writing–review and editing. KN: Conceptualization, Writing–original draft, Writing–review and editing. SK: Conceptualization, Writing–original draft, Writing–review and editing. KS: Writing–original draft, Writing–review and editing. Y-LL: Writing–review and editing.
Funding
The author(s) declare that financial support was received for the research, authorship, and/or publication of this article. Work by RCR is funded via MMS Early Career Grant #80NSSC23K1601. KB is supported by the Austrian Science Fund (FWF): P32175-N27. KN is supported by NASA LWS Grant #80NSSC23K0899. SK is funded by NSF award #2307203. KAS acknowledges support by the NASA DRIVE Science Center for Geospace Storms (CGS) under award 80NSSC22M0163 and NASA grants #80NSSC19K0241, #80NSSC20K1833, #80NSSC17K0679, and #80NSSC24K1106. Y-LL is funded by NASA LWS Grant #80NSSC23K0899 and NSF Grant #2308853. This review was supported by the International Space Science Institute (ISSI) in Bern, through ISSI International Team project #546 “Magnetohydrodynamic Surface Waves at Earth’s Magnetosphere (and Beyond).”
Conflict of interest
The authors declare that the research was conducted in the absence of any commercial or financial relationships that could be construed as a potential conflict of interest.
The author(s) declared that they were an editorial board member of Frontiers, at the time of submission. This had no impact on the peer review process and the final decision.
Publisher’s note
All claims expressed in this article are solely those of the authors and do not necessarily represent those of their affiliated organizations, or those of the publisher, the editors and the reviewers. Any product that may be evaluated in this article, or claim that may be made by its manufacturer, is not guaranteed or endorsed by the publisher.
References
Angelopoulos, V. (2008). The THEMIS mission. Space Sci. Rev. 141, 5–34. doi:10.1007/s11214-008-9336-1
Angelopoulos, V., Kennel, C. F., Coroniti, F. V., Pellat, R., Kivelson, M. G., Walker, R. J., et al. (1994). Statistical characteristics of bursty bulk flow events. J. Geophys. Res. 99, 21257–21280. doi:10.1029/94JA01263
Axford, W. I., and Hines, C. O. (1961). A unifying theory of high latitude geophysical phenomena and geomagnetic storms. Can. J. Phys. 39, 1433–1464. doi:10.1139/p61-172
Blasl, K. A., Nakamura, T. K. M., Nakamura, R., Settino, A., Hasegawa, H., Vörös, Z., et al. (2023). Electron-scale reconnecting current sheet formed within the lower-hybrid wave-active region of kelvin-helmholtz waves. Geophys. Rev. Lett. 50, e2023GL104309. doi:10.1029/2023GL104309
Blasl, K. A., Nakamura, T. K. M., Plaschke, F., Nakamura, R., Hasegawa, H., Stawarz, J. E., et al. (2022). Multi-scale observations of the magnetopause Kelvin–Helmholtz waves during southward IMF. Phys. Plasmas 29, 012105. doi:10.1063/5.0067370
Bowers, K. J., Albright, B. J., Yin, L., Daughton, W., Roytershteyn, V., Bergen, B., et al. (2009) “Advances in petascale kinetic plasma simulation with VPIC and Roadrunner,” in Journal of physics conference series. doi:10.1088/1742-6596/180/1/012055012055
Burch, J. L., Moore, T. E., Torbert, R. B., and Giles, B. L. (2016a). Magnetospheric multiscale overview and science objectives. Space Sci. Rev. 199, 5–21. doi:10.1007/s11214-015-0164-9
Burch, J. L., Torbert, R. B., Phan, T. D., Chen, L.-J., Moore, T. E., Ergun, R. E., et al. (2016b). Electron-scale measurements of magnetic reconnection in space. Science 352, aaf2939. doi:10.1126/science.aaf2939
Burke, W. J., Kelley, M. C., Sagalyn, R. C., Smiddy, M., and Lai, S. T. (1979). Polar cap electric field structures with a northward interplanetary magnetic field. Geophys. Res. Lett. 6, 21–24. doi:10.1029/GL006i001p00021
Burkholder, B. L., Delamere, P. A., Johnson, J. R., and Ng, C.-S. (2020a). Identifying active kelvin–helmholtz vortices on saturn’s magnetopause boundary. Geophys. Res. Lett. 47. doi:10.1029/2019GL084206
Burkholder, B. L., Nykyri, K., Ma, X., Rice, R., Fuselier, S. A., Trattner, K. J., et al. (2020b). Magnetospheric multiscale observation of an electron diffusion region at high latitudes. Geophys. Res. Lett. 47, e2020GL087268. doi:10.1029/2020GL087268
Chaston, C. C., Wilber, M., Fujimoto, M., Goldstein, M. L., Acuna, M., Réme, H., et al. (2007). Mode conversion and anomalous transport in kelvin-helmholtz vortices and kinetic alfvén waves at the earth’s magnetopause. Phys. Rev. Lett. 99, 175004. doi:10.1103/physrevlett.99.175004
Delamere, P. A., Ng, C. S., Damiano, P. A., Neupane, B. R., Johnson, J. R., Burkholder, B., et al. (2021). Kelvin–helmholtz-related turbulent heating at saturn’s magnetopause boundary. J. Geophys. Res. Space Phys. 126, e2020JA028479. doi:10.1029/2020JA028479
Dialynas, K. (2018). Cassini/mimi observations on the dungey cycle reconnection and kelvin-helmholtz instability in saturn’s magnetosphere. J. Geophys. Res. Space Phys. 123, 7271–7275. doi:10.1029/2018JA025840
Dungey, J. W. (1961). Interplanetary magnetic field and the auroral zones. Physcical 6, 47–48. doi:10.1103/PhysRevLett.6.47
Eriksson, S., Lavraud, B., Wilder, F. D., Stawarz, J. E., Giles, B. L., Burch, J. L., et al. (2016). Magnetospheric Multiscale observations of magnetic reconnection associated with Kelvin-Helmholtz waves. Geophys. Rev. Lett. 43, 5606–5615. doi:10.1002/2016GL068783
Escoubet, C. P., Fehringer, M., and Goldstein, M. (2001). Introduction: the cluster mission. Ann. Geophys. 19, 1197–1200. doi:10.5194/angeo-19-1197-2001
Faganello, M., Califano, F., and Pegoraro, F. (2008). Competing mechanisms of plasma transport in inhomogeneous configurations with velocity shear: the solar-wind interaction with earth’s magnetosphere. Phys. Rev. Lett. 100, 015001. doi:10.1103/PhysRevLett.100.015001
Fairfield, D. H., Otto, A., Mukai, T., Kokubun, S., Lepping, R. P., Steinberg, J. T., et al. (2000). Geotail observations of the Kelvin-Helmholtz instability at the eqatorial magnetotail boundary for parallel northward fields. J. Geophys. Res. 105, 21159–21174. doi:10.1029/1999JA000316
Hasegawa, A. (1976). Particle acceleration by mhd surface wave and formation of aurora. J. Geophys. Res. 81, 5083–5090. doi:10.1029/ja081i028p05083
Henry, Z. W., Nykyri, K., Moore, T. W., Dimmock, A. P., and Ma, X. (2017). On the dawn-dusk asymmetry of the kelvin-helmholtz instability between 2007 and 2013. J. Geophys. Res. Space Phys. 122 (11), 888–11,900. doi:10.1002/2017JA024548
Hwang, K.-J., Goldstein, M. L., Kuznetsova, M. M., Wang, Y., Vikas, A. F., and Sibeck, D. G. (2012). The first in situ observation of kelvin-helmholtz waves at high-latitude magnetopause during strongly dawnward interplanetary magnetic field conditions. J. Geophys. Res. Space Phys. 117, 2156–2202. doi:10.1029/2011JA017256
Hwang, K.-J., Kuznetsova, M. M., Sahraoui, F., Goldstein, M. L., Lee, E., and Parks, G. K. (2011). Kelvin-Helmholtz waves under southward interplanetary magnetic field. J. Geophys. Res. Space Phys. 116, A08210. doi:10.1029/2011JA016596
Johnson, J. R., and Cheng, C. (2001). Stochastic ion heating at the magnetopause due to kinetic alfvén waves. Geophys. Res. Lett. 28, 4421–4424. doi:10.1029/2001gl013509
Kavosi, S., and Raeder, J. (2015). Ubiquity of Kelvin-Helmholtz waves at Earth/’s magnetopause. Nat. Commun. 6, 7019. doi:10.1038/ncomms8019
Kavosi, S., Reader, J., Johnson, J. R., Nykyri, K., and Farrugia, C. J. (2023). Seasonal and diurnal variations of Kelvin-Helmholtz Instability at terrestrial magnetopause. Nat. Commun. 14, 2513. doi:10.1038/s41467-023-37485-x
Kim, S.-H., Agrimson, E., Miller, M. J., D’Angelo, N., Merlino, R. L., and Ganguli, G. I. (2004). Amplification of electrostatic ion-cyclotron waves in a plasma with magnetic-field-aligned ion flow shear and no electron current. Phys. plasmas 11, 4501–4505. doi:10.1063/1.1780531
Lembege, B., Ratliff, S., Dawson, J., and Ohsawa, Y. (1983). Ion heating and acceleration by strong magnetosonic waves. Phys. Rev. Lett. 51, 264–267. doi:10.1103/physrevlett.51.264
Li, S.-S., Angelopoulos, V., Runov, A., Kiehas, S. A., and Zhou, X.-Z. (2013). Plasmoid growth and expulsion revealed by two-point ARTEMIS observations. J. Geophys. Res. Space Phys. 118, 2133–2144. doi:10.1002/jgra.50105
Li, T., Li, W., Tang, B., Khotyaintsev, Y. V., Graham, D. B., Ardakani, A., et al. (2023). Kelvin-helmholtz waves and magnetic reconnection at the earth’s magnetopause under southward interplanetary magnetic field. Geophys. Res. Lett. 50, e2023GL105539. doi:10.1029/2023GL105539
Li, W., André, M., Khotyaintsev, Y. V., Vaivads, A., Graham, D. B., Toledo-Redondo, S., et al. (2016). Kinetic evidence of magnetic reconnection due to Kelvin-Helmholtz waves. Geophys. Rev. Lett. 43, 5635–5643. doi:10.1002/2016GL069192
Ma, X., Delamere, P., Otto, A., and Burkholder, B. (2017). Plasma transport driven by the three-dimensional kelvin-helmholtz instability. J. Geophys. Res. Space Phys. 122 (10), 10382–10395. doi:10.1002/2017JA024394
Ma, X., Delamere, P. A., Nykyri, K., Burkholder, B., Neupane, B., and Rice, R. C. (2019). Comparison between fluid simulation with test particles and hybrid simulation for the kelvin-helmholtz instability. J. Geophys. Res. Space Phys. 124, 6654–6668. doi:10.1029/2019JA026890
Ma, X., Delamere, P. A., and Otto, A. (2016). Plasma transport driven by the Rayleigh-taylor instability. J. Geophys. Res. Space Phys. 121, 5260–5271. doi:10.1002/2015JA022122
Ma, X., Otto, A., and Delamere, P. A. (2014a). Interaction of magnetic reconnection and Kelvin-Helmholtz modes for large magnetic shear: 1. Kelvin-Helmholtz trigger. J. Geophys. Res. Space Phys. 119, 781–797. doi:10.1002/2013JA019224
Ma, X., Otto, A., and Delamere, P. A. (2014b). Interaction of magnetic reconnection and Kelvin-Helmholtz modes for large magnetic shear: 2. reconnection trigger. J. Geophys. Res. Space Phys. 119, 808–820. doi:10.1002/2013JA019225
Ma, X., Stauffer, B., Delamere, P. A., and Otto, A. (2015). Asymmetric kelvin-helmholtz propagation at saturn’s dayside magnetopause. J. Geophys. Res. Space Phys. 120, 1867–1875. doi:10.1002/2014JA020746
Matsumoto, Y., and Hoshino, M. (2004). Onset of turbulence induced by a Kelvin-Helmholtz vortex. Geophys. Rev. Lett. 31, L02807. doi:10.1029/2003GL018195
Miura, A. (1987). Simulation of Kelvin-Helmholtz instability at the magnetospheric boundary. J. Geophys. Res. 92, 3195–3206. doi:10.1029/JA092iA04p03195
Montgomery, J., Ebert, R. W., Allegrini, F., Bagenal, F., Bolton, S. J., DiBraccio, G. A., et al. (2023). Investigating the occurrence of kelvin-helmholtz instabilities at jupiter’s dawn magnetopause. Geophys. Res. Lett. 50. doi:10.1029/2023GL102921
Moore, T. W., Nykyri, K., and Dimmock, A. P. (2016). Cross-scale energy transport in space plasmas. Nat. Phys. 12, 1164–1169. doi:10.1038/NPHYS3869
Moore, T. W., Nykyri, K., and Dimmock, A. P. (2017). Ion-scale wave properties and enhanced ion heating across the low-latitude boundary layer during kelvin-helmholtz instability. J. Geophys. Res. Space Phys. 122, 11,128–11,153. doi:10.1002/2017JA024591
Nakamura, T. K., Hayashi, D., Fujimoto, M., and Shinohara, I. (2004). Decay of MHD-scale kelvin-helmholtz vortices mediated by parasitic electron dynamics. Phys. Rev. Lett. 92, 145001. doi:10.1103/PhysRevLett.92.145001
Nakamura, T. K. M., Blasl, K. A., Hasegawa, H., Umeda, T., Liu, Y. H., Peery, S. A., et al. (2022a). Multi-scale evolution of Kelvin-Helmholtz waves at the Earth’s magnetopause during southward IMF periods. Phys. Plasmas 29, 012901. doi:10.1063/5.0067391
Nakamura, T. K. M., Blasl, K. A., Liu, Y. H., and Peery, S. A. (2022b). Diffusive plasma transport by the magnetopause Kelvin-Helmholtz instability during southward IMF. Front. Astronomy Space Sci. 8. doi:10.3389/fspas.2021.809045
Nishino, M., Fujimoto, M., Ueno, G., Maezawa, K., Mukai, T., and Saito, Y. (2007). Geotail observations of two-component protons in the midnight plasma sheet. Ann. Geophys. Copernic. Publ. Göttingen, Ger. 25, 2229–2245. doi:10.5194/angeo-25-2229-2007
Nykyri, K. (2013). Impact of MHD shock physics on magnetosheath asymmetry and Kelvin-Helmholtz instability. J. Geophys. Res. Space Phys. 118, 5068–5081. doi:10.1002/jgra.50499
Nykyri, K., Grison, B., Cargill, P., Lavraud, B., Lucek, E., Dandouras, I., et al. (2006a). Origin of the turbulent spectra in the high-altitude cusp: cluster spacecraft observations. Ann. Geophys. Copernic. GmbH 24, 1057–1075. doi:10.5194/angeo-24-1057-2006
Nykyri, K., Lucek, P. J. C. E. A., Horbury, T. S., Balogh, A., Lavraud, B., Dandouras, I., et al. (2003a). Ion cyclotron waves in the high altitude cusp: CLUSTER observations at varying spacecraft separations. Geophys. Res. Lett. 30. doi:10.1029/2003GL018594
Nykyri, K., Ma, X., Burkholder, B., Rice, R., Johnson, J., Kim, E.-K., et al. (2021a). MMS observations of the multi-scale wave structures and parallel electron heating in the vicinity of the southern exterior cusp. J. Geophys. Res. Space Phys. 126. doi:10.1029/2019JA027698
Nykyri, K., Ma, X., and Johnson, J. (2021b). Cross-scale energy transport in space plasmas. Am. Geophys. Union AGU, 109–121. chap. 7. doi:10.1002/9781119815624.ch7
Nykyri, K., and Otto, A. (2001). Plasma transport at the magnetospheric boundary due to reconnection in Kelvin-Helmholtz vortices. Geophys. Res. Lett. 28, 3565–3568. doi:10.1029/2001GL013239
Nykyri, K., and Otto, A. (2004). Influence of the Hall term on KH instability and reconnection inside kh vortices. Ann. Geophys. 22, 935–949. doi:10.5194/angeo-22-935-2004
Nykyri, K., Otto, A., Büchner, J., Nikutowski, B., Baumjohann, W., Kistler, L. M., et al. (2003b). Equator-S observations of boundary signatures: FTE’s orKelvin-Helmholtz waves? Wash. D.C. Am. Geophys. Union Geophys. Monogr. Ser. 133, 205–210. doi:10.1029/133GM20
Nykyri, K., Otto, A., Lavraud, B., Mouikis, C., Kistler, L., Balogh, A., et al. (2006b). Cluster observations of reconnection due to the Kelvin-Helmholtz instability at the dawn side magnetospheric flank. Ann. Geophys. 24, 2619–2643. doi:10.5194/angeo-24-2619-2006
Oieroset, M., Raeder, J., Phan, T. D., Wing, S., McFadden, J. P., Li, W., et al. (2005). Global cooling and densification of the plasma sheet during an extended period of purely northward IMF on October 22–24, 2003. Geophys. Res. Lett. 32. doi:10.1029/2004GL021523
Osmane, A., Dimmock, A. P., Naderpour, R., Pulkkinen, T. I., and Nykyri, K. (2015). The impact of solar wind ULFBzfluctuations on geomagnetic activity for viscous timescales during strongly northward and southward IMF. J. Geophys. Res. Space Phys. 120, 9307–9322. doi:10.1002/2015JA021505
Otto, A., and Fairfield, D. H. (2000). Kelvin-Helmholtz instability at the magnetotail boundary: MHD simulation and comparison with Geotail observations. J. Geophys. Res. 105, 21175–21190. doi:10.1029/1999ja000312
Paral, J., and Rankin, R. (2013). Dawn–dusk asymmetry in the kelvin–helmholtz instability at mercury. Nat. Commun. 4, 1645. doi:10.1038/ncomms2676
Paschmann, G., Papamastorakis, I., Sckopke, N., Haerendel, G., Sonnerup, B. U. O., Bame, S. J., et al. (1979). Plasma acceleration at the earth’s magnetopause - evidence for reconnection. Nature 282, 243–246. doi:10.1038/282243a0
Peñano, J. R., and Ganguli, G. (2002). Generation of electromagnetic ion cyclotron waves in the ionosphere by localized transverse dc electric fields. J. Geophys. Res. Space Phys. 107, 14–17. doi:10.1029/2001JA000279
Poh, G., Espley, J. R., Nykyri, K., Fowler, C. M., Ma, X., Xu, S., et al. (2021). On the growth and development of non-linear kelvin–helmholtz instability at mars: maven observations. J. Geophys. Res. Space Phys. 126. doi:10.1029/2021JA029224
Ranquist, D. A., Bagenal, F., Wilson, R. J., Hospodarsky, G., Ebert, R. W., Allegrini, F., et al. (2019). Survey of jupiter’s dawn magnetosheath using juno. J. Geophys. Res. Space Phys. 124, 9106–9123. doi:10.1029/2019JA027382
Rice, R. C., Nykyri, K., Ma, X., and Burkholder, B. L. (2022). Characteristics of kelvin–helmholtz waves as observed by the mms from september 2015 to march 2020. J. Geophys. Res. Space Phys. 127. doi:10.1029/2021JA029685
Ruhunusiri, S., Halekas, J. S., McFadden, J. P., Connerney, J. E. P., Espley, J. R., Harada, Y., et al. (2016). Maven observations of partially developed kelvin-helmholtz vortices at mars. Geophys. Res. Lett. 43, 4763–4773. doi:10.1002/2016GL068926
Settino, A., Malara, F., Pezzi, O., Onofri, M., Perrone, D., and Valentini, F. (2020). Kelvin-Helmholtz instability at proton scales with an exact kinetic equilibrium. Astrophysical J. 901, 17. doi:10.3847/1538-4357/abada9
Settino, A., Perrone, D., Khotyaintsev, Y. V., Graham, D. B., and Valentini, F. (2021). Kinetic features for the identification of kelvin-helmholtz vortices in in situ observations. Astrophysical J. 912, 154. doi:10.3847/1538-4357/abf1f5
Sorathia, K. A., Merkin, V. G., Ukhorskiy, A. Y., Allen, R. C., Nykyri, K., and Wing, S. (2019). Solar wind ion entry into the magnetosphere during northward imf. J. Geophys. Res. Space Phys. 124, 5461–5481. doi:10.1029/2019JA026728
Sorriso-Valvo, L., Catapano, F., Retinò, A., Le Contel, O., Perrone, D., Roberts, O. W., et al. (2019). Turbulence-driven ion beams in the magnetospheric kelvin-helmholtz instability. Phys. Rev. Lett. 122, 035102. doi:10.1103/PhysRevLett.122.035102
Stawarz, J. E., Eriksson, S., Wilder, F. D., Ergun, R. E., Schwartz, S. J., Pouquet, A., et al. (2016). Observations of turbulence in a kelvin-helmholtz event on 8 september 2015 by the magnetospheric multiscale mission. J. Geophys. Res. Space Phys. 121, 11,021–11,034. doi:10.1002/2016JA023458
Taylor, M. G. G. T., Hasegawa, H., Lavraud, B., Phan, T., Escoubet, C. P., Dunlop, M. W., et al. (2012). Spatial distribution of rolled up Kelvin-Helmholtz vortices at Earth’s dayside and flank magnetopause. Ann. Geophys. 30, 1025–1035. doi:10.5194/angeo-30-1025-2012
Terasawa, T., and Nambu, M. (1989). Ion heating and acceleration by magnetosonic waves via cyclotron subharmonic resonance. Geophys. Res. Lett. 16, 357–360. doi:10.1029/gl016i005p00357
Wang, X., Xu, X., Ye, Y., Wang, J., Wang, M., Zhou, Z., et al. (2022). Maven observations of the kelvin-helmholtz instability developing at the ionopause of mars. Geophys. Res. Lett. 49. doi:10.1029/2022GL098673
Wilder, F., Ergun, R., Schwartz, S., Newman, D., Eriksson, S., Stawarz, J., et al. (2016). Observations of large-amplitude, parallel, electrostatic waves associated with the kelvin-helmholtz instability by the magnetospheric multiscale mission. Geophys. Res. Lett. 43, 8859–8866. doi:10.1002/2016gl070404
Wing, S., Johnson, J. R., Chaston, C. C., Echim, M., Escoubet, C. P., Lavraud, B., et al. (2014). Review of solar wind entry into and transport within the plasma sheet. Space Sci. Rev. 184, 33–86. doi:10.1007/s11214-014-0108-9
Wing, S., Johnson, J. R., Newell, P. T., and Meng, C.-I. (2005). Dawn-dusk asymmetries, ion spectra, and sources in the northward interplanetary magnetic field plasma sheet. J. Geophys. Res. 110. doi:10.1029/2005JA011086
Keywords: Kelvin-Helmholtz instability, plasma transport, reconnection, multi-scale processes, diffusive transport, turbulence
Citation: Rice RC, Blasl KA, Nykyri K, Kavosi S, Sorathia KA and Liou Y-L (2024) Multi-scale processes of the Kelvin-Helmholtz instability at Earth’s magnetopause. Front. Astron. Space Sci. 11:1464010. doi: 10.3389/fspas.2024.1464010
Received: 12 July 2024; Accepted: 27 August 2024;
Published: 16 September 2024.
Edited by:
Michel Blanc, UMR5277 Institut de recherche en astrophysique et planétologie (IRAP), FranceReviewed by:
Binbin Ni, Wuhan University, ChinaCopyright © 2024 Rice, Blasl, Nykyri, Kavosi, Sorathia and Liou. This is an open-access article distributed under the terms of the Creative Commons Attribution License (CC BY). The use, distribution or reproduction in other forums is permitted, provided the original author(s) and the copyright owner(s) are credited and that the original publication in this journal is cited, in accordance with accepted academic practice. No use, distribution or reproduction is permitted which does not comply with these terms.
*Correspondence: Rachel C. Rice, cmNyaWNlQHVtZC5lZHU=