- NASA Jet Propulsion Laboratory, California Institute of Technology, Pasadena, CA, United States
The search for biosignatures on Mars has been a high priority for astrobiology. The approach to detecting putative biosignatures has largely been focused on chemical analyses targeting predominantly extinct life. However, this approach has limited the characterization of extant life, preventing differentiation between extinct and extant biosignatures. Detecting an extant martian lifeform requires approaches focused on identification of biological features. Identifying potential features of life, such as growth or reproduction, can contribute evidence necessary to identify extant biosignatures. While an unambiguous extant biosignature might not be possible with biologically focused approaches, the combined data can provide supporting evidence to attribute a biosignature to an extinct or extant lifeform in conjunction with flight tested instrumentation. With upcoming initiatives, such as the planned Mars Sample Return campaign and the Mars Life Explorer mission concept, the incorporation of extant life specific analysis is paramount for the future of Mars exploration.
1 Introduction
Our neighbor, Mars, once had habitable conditions before the surface became inhospitable (e.g., Westall et al., 2013; Cockell, 2014; Grotzinger et al., 2014; Cockell et al., 2016; Cabrol, 2018). Given this context, the search for martian life has typically focused on detecting ancient “extinct” life, but recent research has identified potential “special regions” where temperature and water availability may be suitable for extant life (Rummel et al., 2014). To persist, potential extant martian life would need to co-evolved with its environment and evolved favorable traits for harsh present-day conditions.
The detection of biosignatures guide the search for life (NASEM, 2022). For this perspective, we adopt the definition “A biosignature is any phenomenon for which biological processes are a known possible explanation and whose potential abiotic causes have been reasonably explored and ruled out” (NASEM, 2022; Gillen et al., 2023). Notably, none of the common definitions of biosignatures differentiate between extinct and extant biosignatures (Gillen et al., 2023). We define extinct life as geologically preserved life that can no longer exhibit characteristics of metabolism, reproduction, and growth. We define extant life as systems that have the potential to perform metabolic redox reactions, reproduction, and growth (Conrad and Nealson, 2001; Benner, 2010; Westall and Cavalazzi, 2011; Neveu et al., 2018). Therefore, a confirmed detection of extant martian life requires evidence of an organism carrying out metabolism-like redox reactions, or evidence of current growth or reproduction (Conrad and Nealson, 2001; Benner, 2010; Westall and Cavalazzi, 2011; Carrier et al., 2020).
The ladder of life detection (LoLD) (Neveu et al., 2018), outlines a framework wherein a biosignature’s utility depends on its detectability and survivability, differentiability from abiotic sources, and whether it is agnostic. Additionally, the instrumental methods must be sensitive enough for accurate detection, free of contamination, and the measurements must be repeatable in triplicate (Neveu et al., 2018). Specific measurable features possibly representative of life include, organics not found abiotically, macromolecule building blocks, and distribution of metals (Neveu et al., 2018).
Some rungs on the LoLD are indicative of extant life (such as Darwinian evolution, growth and reproduction). While Viking (Klein, 1978) carried out some biological experiments, the inclusion of such experimentation on subsequent mission payloads has been limited. Since Viking, mission design and instrument payloads have focused on molecular biosignatures that limit identifying biological features. Current flight-ready detection methods do not observe biological functions, but chemical or physical byproducts, which could also result from abiotic reactions. For example, instruments such as gas chromatography-mass spectrometry (GC-MS), - historically a common mission instrument - are designed to detect the compounds and chemical patterns associated with life.
Therefore, an “extant biosignature” must be indicative of life and be attributed to ongoing biology. To robustly detect extant lifeforms, the focus must be on the features and processes associated with active organisms, such as the potential to perform metabolism, growth and reproduction. Many biological and biochemical analytical techniques (e.g., those measuring turbidity, conductivity, or various microscopic observables; Casida, 1971; Richards et al., 1978; Castro et al., 1995; Madrid et al., 1999; Li et al., 2004) are tailored to detecting and qualifying active biological processes. While there are short-lived molecules which may indicate recent biotic input, (e.g., DNA), these molecules rapidly degrade under exposure to martian surface conditions (e.g., Fajardo-Cavazos et al., 2010) and can be difficult to extract from samples, even from an extant biological cell (Gates, 2009; Jiang et al., 2012; Mojarro et al., 2017). Additionally, acquisition and analysis of samples may cause alteration (e.g., as a potential source of chlorination, decarboxylation, and oxidation, Freissinet et al., 2020). Therefore, it is possible that even if a chemical biosignature is detected, it may not be possible to differentiate whether the signal is an extant or extinct biosignature.
The two Viking landers carried three biological experiments to perform a life detection mission on Mars in the 1970s (Klein et al., 1976; Levin and Straat, 1977; Brown et al., 1978; Klein, 1978). First, a gas exchange experiment showed CO2 and O2 uptake upon “wetting” of regolith, followed by a “release” of CO2. Second, labeled release experiments were designed to measure uptake of organic compounds by microorganisms by adding radioactively labeled organics to regolith samples and observe any labeled volatile compounds produced as waste from potential metabolic activity. These experiments showed immediate release of labeled organics. Finally, the pyrolytic release experiment measured carbon fixation of CO2 and CO into organic compounds, to test for potential microbial carbon fixation over a few days. Small amounts of organic matter were detected after the experiments but were not affected by heating (essentially sterilizing the sample) indicating the process that synthesized the organic matter might not be biological. The results have been highly debated, but are largely attributed to non-biologic perchlorate reactions (Hecht et al., 2009). Lessons from Viking can inform improved experimental frameworks for life detection on missions as we move forward.
Below, we describe approaches to life detection on Mars, and argue that additional measurements of potential biological function are necessary for extant life detection. While more recent Mars life detection approaches are largely calibrated to detect fossilized life, detection methods should also be calibrated to determine if the organism(s) are currently viable.
2 The search for martian biosignatures guided by the early Earth biosphere
Our understanding of life on Earth has developed through decades of conducting multiscale interdisciplinary investigations, guiding site selection for astrobiological investigations on Mars (Summons et al., 2011). Earth is the only data point we have for life, so we consider where life may have emerged on Earth and compare these sites to the geological settings on early Mars. For example, evidence for more abundant water on the surface of Mars exists from the late Noachian until the end of the Hesperian (3.8–3 Ga) (McKay et al., 1996; Grotzinger et al., 2014) with evidence for lakes, rivers, potential hot springs, and hydrothermalism (Ehlmann et al., 2008; Schwenzer and Kring, 2009; Grotzinger et al., 2014; Ruff and Farmer, 2016) presenting potentially habitable environments in which life could have emerged.
Analog environments from similar evolutionary eras on Earth allow researchers to observe features that may be indicative of life and form hypotheses about their origin and evolution (Preston and Dartnell, 2014). Terrestrial sites analogous to Mars are typically selected based on some level of similarity with a specific martian environment. In terrestrial analogs, microbial signatures have poor morphological preservation, and primarily depict traces of community scale interactions of microbes with sediments (e.g., stromatolites) with rare examples of possible microfossils (Sugitani et al., 2009). It can be challenging to untangle primary and secondary signals in ancient fossils, leading to common debates about the process behind the suspected biosignature (Nutman et al., 2016; Allwood et al., 2018). However, a limitation of Early Earth analogs is that Earth has plate tectonics–a primary cause of alteration - while Mars does not currently have plate tectonics and the extent to which it ever did is unknown (e.g., Zhou et al., 2022). Increasingly, laboratory studies have been utilized to understand possible martian processes that could lead to the formation of biotic and abiotic signals (McMahon, 2019; Ruff et al., 2020). No single analog represents a perfect match of another planetary body. Yet, through a combination of laboratory simulations, modelling and spaceflight data we are able to build a more complete picture of planetary conditions to direct biosignature constraints and detection methodologies during mission development.
Lipids are an organic biosignature highly resistant to degradation and preservable in deep time. During diagenesis they typically lose functional groups or become saturated, e.g., the microbial lipid hopanol loses its functional OH group to become a hopane (Ourisson et al., 1979; Ourisson and Albrecht, 1992). Although they are altered, many lipids can still be traced to particular biochemical pathways, community structures and microbial phylogenies and can provide information on the depositional environment (Vestal and White, 1989; Sturt et al., 2004; Summons et al., 2022). Because of this utility and preservability, Summons et al. (2011) proposed a strategy to look for organic matter preserved within ancient sedimentary deposits on Mars. This approach led to the detection of a variety of molecules with Sample Analysis at Mars (SAM) on Curiosity and SHERLOC on Perseverance (Freissinet et al., 2015; Eigenbrode et al., 2018; Scheller et al., 2022; Sharma et al., 2023). However, these detections have been impeded by source ambiguity where meteorites may represent a large source of potential abiotic organic matter (Summons et al., 2011; Stern et al., 2022). The presence of strong oxidants and ionizing radiation in the martian regolith further complicate interpretations, processes known to alter even the most recalcitrant polyaromatic molecules (Benner et al., 2000). For example, UV irradiation experiments show degradation of aromatic compounds after only 6 hours (Kopacz et al., 2023). However, in most organic geological studies on Earth, these compounds are highly resistant to alteration (Grotheer et al., 2015), and are detectable in highly metamorphosed ∼2.7 Ga Archean rocks (French et al., 2015). This example highlights that our understanding of what is recalcitrant on Earth may not be applicable to Mars.
3 Evolutionary constraints on potential extant martian life
Terrestrial analogs from extreme environments are limited by Earth-specific evolutionary contexts (Michalski et al., 2018), as potential martian organisms would have adapted and coevolved with a changing Mars rather than Earth. The evolutionary history of Earth can provide a framework to understand how martian organisms could have adapted as Mars transitioned to a drier, colder, geologically dead world. On Earth, life eventually adapted to almost all terrestrial environments. For example, a martian analog, the Atacama Desert, is home to modern extremophiles adapted to these hygroscopic environments (Davila and Schulze-Makuch, 2016). Like Mars, the Atacama has low water availability, high UV and large temperature fluxes. At the Atacama, microorganisms have adapted to the dry and perchlorate rich conditions (Horneck, 2000; Navarro-González et al., 2003; Bull and Asenjo, 2013; Preston and Dartnell, 2014). For example, some cyanobacteria in the Atacama incorporate pigments as a UV protectant against the high radiation levels, or colonize areas that are more likely to concentrate water (Azua-Bustos et al., 2012). In these harsh environments, life is so highly adapted to specific microenvironments (e.g., soil, inside rocks) that there is little crossover between species, resulting in highly specialized communities (Wierzchos et al., 2012). Since life on Earth had the capability to evolve and survive in harsh environments analogous to modern Mars, it is possible that potential martian organisms underwent a similar transition.
Compounds important to metabolisms have been detected on ancient and modern Mars. For example, redox important elements used on Earth by phototrophs (such as Fe2+ as observed in photoferrotrophy) and chemotropism (H2/SO42- for sulfate reduction) have been hypothesized and detected on Mars (Cockell, 2014; Westall et al., 2015; Tarnas et al., 2018). The evolution of heterotrophs, including chemolithotrophs and chemoorganotrophs, would have been possible within ancient martian hydrothermal systems (Schulze-Makuch et al., 2005; Schwenzer and Kring, 2009; Ruff and Farmer, 2016), where they could utilize inorganic (such as ferrous-bearing minerals like olivine) and organic resources. While these metabolisms might have emerged, a changing martian environment would have driven some organisms to extinction, at least near the surface. For example, increasing scarcity of liquid water on the surface, along with the increasing radiation, would have caused extinction events for potential photosynthetic organisms as access to light would have required their presence on the uninhabitable surface (Horneck, 2000; Schulze-Makuch et al., 2005; Westall et al., 2013; 2015; Michalski et al., 2018). However, other autotrophic metabolisms, such as methanogenesis which is anaerobic and non-photosynthetic, would have been possible within more habitable locations on modern Mars. Some methanogenic species are capable of surviving in the presence of perchlorates, common compounds in modern martian regolith (Shcherbakova et al., 2015; Kral et al., 2016).
One potential environment of sustained martian habitability is the subsurface, specifically at depths where water, lack of radiation, and connected environments (e.g., caves or lava tubes), would allow for a more global distribution of extant life (Horneck, 2000; Schulze-Makuch et al., 2005; Cabrol, 2018; 2021). While the depth would vary within the specific era in the evolution of the martian surface, any depth that could maintain habitable environments (protection from radiation, increased water activity) could harbor life. On Earth, organisms adapt to similarly niche environments. The Antarctic Dry Valleys also have an arid climate and high UV irradiance (Preston and Dartnell, 2014), and organisms here employ a similar method of survival, colonizing under rocks to block high UV radiation (Cary et al., 2010). While the potential for continued evolution within these environments is low (Warren-Rhodes et al., 2006; Goordial et al., 2016), these examples show mitigation strategies biology can employ to survive. Investigating biological life within these environments also informs best practices for observing extremophiles. For example, specifically choosing locations in which life could be protected (i.e., under ice/rocks), could be translated into mission architecture and landing site selection, such as in the Mars Life Explorer (MLE) mission concept, which would target low latitude ices to look for extant martian life (NASEM, 2022).
4 Approaches to detecting extant martian life
When the Viking spacecrafts landed on Mars, they discovered an apparently barren wasteland (Soffen and Snyder, 1976). Because of this, instrumentation on subsequent missions has focused on understanding the geological and environmental history of Mars. The Sojourner rover detected geological evidence of a large flood (Rieder et al., 1997), while Spirit and Opportunity demonstrated that water activity was widespread (Squyres et al., 2006). More recently, Curiosity has revealed that Gale Crater records a 3.6 Ga habitable shallow lake environment (Grotzinger et al., 2014), that preserves a variety of hydrocarbons (e.g., Freissinet et al., 2015; Millan et al., 2016; 2022; Eigenbrode et al., 2018; Stern et al., 2022) similar to early life environments on Earth (Allwood et al., 2007; Marshall et al., 2007). Since 2020, the Perseverance rover has been studying a deltaic system for signs of ancient biosignatures (Farley et al., 2020), and has revealed fluorescence signals consistent with aromatic hydrocarbons in several samples which are being cached to be brought back to Earth in the proposed Mars Sample Return campaign (Scheller et al., 2022; Sharma et al., 2023). While this approach has provided essential data in our understanding of Mars, in general, there has been a lack of mission focus on the detection of extant life due to the hostile martian surface conditions. However, some recently proposed missions have incorporated instruments for extant life detection. For example, the MLE mission concept prioritized by the 2023–2032 Planetary Decadal Survey, would look for extant life by investigating organics within martian ice deposits (NASEM, 2022).
As we move to investigating a potential extant martian biosphere, we must investigate methods to differentiate between extant and extinct biosignatures, and assess if current mission architecture is capable of distinguishing between the two. Extant biosignature detection depends on our ability to classify whether a signature is indicative of extant biology, paleobiology, or purely geological (Figure 1). Within current mission architectures, features identified during the search for biosignatures are largely attributed to either geological or biological sources. However, the search for extant life introduces additional complexity, wherein potential geological, biological and paleobiological sources need to be untangled.
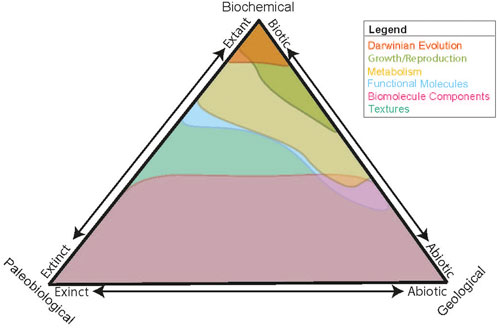
Figure 1. A ternary diagram depicting the rungs described in the Ladder of Life Detection (Neveu et al., 2018) and where they plot in their likelihood of being extant, extinct, biotic or abiotic. To detect extant life, potential biosignatures must be differentiated from biotic and abiotic sources and must also distinguish between extant (biological) and extinct life (paleobiological). Within this new framework, potential biosignatures can be recontextualized to support evidence of geological, paleobiological, and biological sources, and are depicted on this diagram in terms of their relationship to each category.
Molecules retaining structure and function can be preserved across the geological record, unaltered eukaryotic lipids (sterols) have been detected in the Devonian (∼380 Ma) (Melendez et al., 2013). Analytical methods common on mission instrumentation, such as GC-MS, are often used to detect and identify these compounds, and data obtained are interpreted to determine origin. However, information gained from this approach is often limited as many compounds can also be produced abiotically (e.g., Barge et al., 2022). Additionally, while growth and reproduction are primarily regulated to extant biology; there are some abiotic processes which exhibit growth, (e.g., chemical gardens, crystals), and thus are depicted in Figure 1 as being representative of extant and abiotic processes, but not paleobiological ones (McMahon and Cosmidis, 2021).
To unambiguously determine if a biosignature is from an extant source, some indication of biological activity is required. Features such as Darwinian evolution, metabolism, and reproduction can provide the necessary information to detect extant life. Previous approaches to biosignatures have focused on identifying chemical compounds and their distributions, and then extracting biological significance from the resulting data. For extant life detection, instrumentation capable of providing evidence of biological function is required. Since the Viking mission, instrumentation dedicated to observing biological features has not been prioritized. In addition, direct observations of Darwinian evolution are most likely not possible within a mission timescale, limiting our ability to detect extant life by missions. While we do not advocate for any specific biological technique, there are some existing laboratory methods that could be optimized for mission development.
As demonstrated by Viking, biologically directed measurements are not without heritage. Features of life can be observed through techniques that can be adapted into mission ready instrumentation. Detection of reproduction (and subsequently growth), require approaches sensitive to these processes, such as measurement of sample turbidity or conductivity (Richards et al., 1978; Castro et al., 1995; Madrid et al., 1999). On Earth, these data can be used to measure bacterial cultures, growth curves, activity levels, etc. Even within an optimized laboratory, microbial growth is challenging, often resulting in low yields (Wade, 2002). However, there are mitigation strategies to help improve culture rates, such as optimizing for host mineralogy and environmental context (Wilson et al., 1997), improving the utility of this technique. To translate these lessons into mission concepts, we should formulate and optimize instrumentation with slow biological functions in mind. Metabolism detection presents a more complex problem as many metabolic byproducts (such as O2 and CO2) can be produced abiotically, and their provenance is often difficult to assess. For example, measuring changes in responses to nutrients can provide evidence of a metabolic reaction. However, in lessons from the Viking mission (where the results have been attributed to geological redox reactions), we know that environmental context must also be investigated and understood to account for false detections of extant life. Lastly, growth can be observed through various biological techniques, including microscopic observation of changes in cells and cellular features, such as an increase in cell count or identification of cells at various stages of growth. Some types of microscopy (such as light-diffraction, fluorescence, and confocal laser scanning microscopy) are capable of observing cellular features in soil samples (Casida, 1971; Li et al., 2004). Importantly, a potential martian biosphere might contain microorganisms with slow metabolic, reproductive, and growth rates, necessitating instruments with sensitive detection limits. Including techniques with high signal-to-noise ratio [e.g., observing sub-micron bacterial growth through changes in microfluidic systems (Song et al., 2010; Fernandez et al., 2017)], or storing samples over a longer period of time to be reanalyzed later could help detect microorganisms with slower, smaller biological responses.
Building on Viking’s foundation can provide a framework to help distinguish extant and extinct life. The ambiguous results from the biological experiments were the result of unexpected environmental factors (perchlorates) (Hecht et al., 2009) stemming from a lack of knowledge of the martian surface conditions. Since Viking, we have gained a better understanding of complementary biosignatures, martian environments and improved flight ready technology. In the future, incorporating biologically focused instrumentation that expands on the potential biological functions of the molecule could provide more evidence for extant life. In particular, multiple lines of evidence will be needed for robust interpretations, such as classifying attributes of functional molecules (such as polymer length and shape) in addition to detecting chemical biomolecular components could provide an indication of additional complexity missed by classic chemical techniques (such as GC-MS).
5 Conclusion
Life may have emerged on early Mars and adapted to persist into the present day. The last National Academies Planetary Science Strategy prioritized a Mars mission to seek extant life, yet, current missions are not designed to differentiate between extinct and extant biosignatures. Data indicating ongoing biological activity is required to identify an extant organism. While the LoLD provides some examples of indicators of extant life, current flight-ready instrumentation generally measures chemical byproducts of life, and cannot speak to the state of the original source. Previous missions, such as Viking, have shown the potential for biological investigations utilizing mission architecture. Incorporating analytical approaches specific to biological features into future missions can provide information necessary to detect extant martian biosignatures.
Data availability statement
The original contributions presented in the study are included in the article/supplementary material, further inquiries can be directed to the corresponding authors.
Author contributions
KAD: Conceptualization, Investigation, Visualization, Writing–original draft, Writing–review and editing. BLT: Conceptualization, Investigation, Visualization, Writing–original draft, Writing–review and editing.
Funding
The author(s) declare that financial support was received for the research, authorship, and/or publication of this article. BLT supported by NASA Planetary Science and Technology from Analog Research (PSTAR) grant 80NSSC18K1651. KAD was supported by the NASA Astrobiology Program under the Joint NASA-NSF Ideas Lab on the Origins of Life. This work was carried out at the Jet Propulsion Laboratory, California Institute of Technology, under a contract with NASA (80NM0018D0004).
Acknowledgments
We acknowledge helpful discussions with Laura M. Barge. We are grateful to the two reviewers and the editor for their suggestions and thoughtful comments which have substantially improved our manuscript. Copyright 2024 California Institute of Technology. Government sponsorship acknowledged. The decision to implement Mars Sample Return will not be finalized until NASA’s completion of the National Environmental Policy Act (NEPA) process. This document is being made available for information purposes only.
Conflict of interest
The authors declare that the research was conducted in the absence of any commercial or financial relationships that could be construed as a potential conflict of interest.
Publisher’s note
All claims expressed in this article are solely those of the authors and do not necessarily represent those of their affiliated organizations, or those of the publisher, the editors and the reviewers. Any product that may be evaluated in this article, or claim that may be made by its manufacturer, is not guaranteed or endorsed by the publisher.
References
Allwood, A. C., Rosing, M. T., Flannery, D. T., Hurowitz, J. A., and Heirwegh, C. M. (2018). Reassessing evidence of life in 3,700-million-year-old rocks of Greenland. Nature 563, 241–244. doi:10.1038/s41586-018-0610-4
Allwood, A. C., Walter, M. R., Burch, I. W., and Kamber, B. S. (2007). 3.43 billion-year-old stromatolite reef from the Pilbara Craton of Western Australia: ecosystem-scale insights to early life on Earth. Precambrian Res. 158, 198–227. doi:10.1016/j.precamres.2007.04.013
Azua-Bustos, A., Urrejola, C., and Vicuña, R. (2012). Life at the dry edge: microorganisms of the Atacama Desert. FEBS Lett. 586, 2939–2945. doi:10.1016/j.febslet.2012.07.025
Barge, L. M., Rodriguez, L. E., Weber, J. M., and Theiling, B. P. (2022). Determining the “biosignature threshold” for life detection on biotic, abiotic, or prebiotic worlds. Astrobiology 22, 481–493. doi:10.1089/ast.2021.0079
Benner, S. A., Devine, K. G., Matveeva, L. N., and Powell, D. H. (2000). The missing organic molecules on Mars. Proc. Natl. Acad. Sci. 97, 2425–2430. doi:10.1073/pnas.040539497
Brown, F. S., Adelson, H. E., Chapman, M. C., Clausen, O. W., Cole, A. J., Cragin, J. T., et al. (1978). The biology instrument for the Viking Mars mission. Rev. Sci. Instrum. 49, 139–182. doi:10.1063/1.1135378
Bull, A. T., and Asenjo, J. A. (2013). Microbiology of hyper-arid environments: recent insights from the Atacama Desert, Chile. Antonie Leeuwenhoek 103, 1173–1179. doi:10.1007/s10482-013-9911-7
Cabrol, N. A. (2018). The coevolution of life and environment on mars: an ecosystem perspective on the robotic exploration of biosignatures. Astrobiology 18, 1–27. doi:10.1089/ast.2017.1756
Cabrol, N. A. (2021). Tracing a modern biosphere on Mars. Nat. Astron 5, 210–212. doi:10.1038/s41550-021-01327-x
Carrier, B. L., Beaty, D. W., Meyer, M. A., Blank, J. G., Chou, L., DasSarma, S., et al. (2020). Mars extant life: what’s next? Conference report. Astrobiology 20, 785–814. doi:10.1089/ast.2020.2237
Cary, S. C., McDonald, I. R., Barrett, J. E., and Cowan, D. A. (2010). On the rocks: the microbiology of Antarctic Dry Valley soils. Nat. Rev. Microbiol. 8, 129–138. doi:10.1038/nrmicro2281
Casida, L. E. (1971). Microorganisms in unamended soil as observed by various forms of microscopy and staining. Appl. Microbiol. 21, 1040–1045. doi:10.1128/am.21.6.1040-1045.1971
Castro, G. R., Andribet, E. P., Ducrey, L. M., Garro, O. A., and Siñeriz, F. (1995). Modelling and operation of a turbidity-meter for on-line monitoring of microbial growth in fermenters. Process Biochem. 30, 767–772. doi:10.1016/0032-9592(95)00002-X
Cockell, C. S. (2014). Trajectories of martian habitability. Astrobiology 14, 182–203. doi:10.1089/ast.2013.1106
Cockell, C. S., Bush, T., Bryce, C., Direito, S., Fox-Powell, M., Harrison, J. P., et al. (2016). Habitability: a review. Astrobiology 16, 89–117. doi:10.1089/ast.2015.1295
Conrad, P. G., and Nealson, K. H. (2001). A non-earthcentric approach to life detection. Astrobiology 1, 15–24. doi:10.1089/153110701750137396
Davila, A. F., and Schulze-Makuch, D. (2016). The last possible outposts for life on mars. Astrobiology 16, 159–168. doi:10.1089/ast.2015.1380
Ehlmann, B. L., Mustard, J. F., Murchie, S. L., Poulet, F., Bishop, J. L., Brown, A. J., et al. (2008). Orbital identification of carbonate-bearing rocks on mars. Science 322, 1828–1832. doi:10.1126/science.1164759
Eigenbrode, J. L., Summons, R. E., Steele, A., Freissinet, C., Millan, M., Navarro-González, R., et al. (2018). Organic matter preserved in 3-billion-year-old mudstones at Gale crater, Mars. Science 360, 1096–1101. doi:10.1126/science.aas9185
Fajardo-Cavazos, P., Schuerger, A. C., and Nicholson, W. L. (2010). Exposure of DNA and Bacillus subtilis spores to simulated martian environments: use of quantitative PCR (qPCR) to measure inactivation rates of DNA to function as a template molecule. Astrobiology 10, 403–411. doi:10.1089/ast.2009.0408
Farley, K. A., Williford, K. H., Stack, K. M., Bhartia, R., Chen, A., de la Torre, M., et al. (2020). Mars 2020 mission overview. Space Sci. Rev. 216, 142. doi:10.1007/s11214-020-00762-y
Fernandez, R. E., Rohani, A., Farmehini, V., and Swami, N. S. (2017). Review: microbial analysis in dielectrophoretic microfluidic systems. Anal. Chim. Acta 966, 11–33. doi:10.1016/j.aca.2017.02.024
Freissinet, C., Glavin, D. P., Mahaffy, P. R., Miller, K. E., Eigenbrode, J. L., Summons, R. E., et al. (2015). Organic molecules in the sheepbed mudstone, Gale Crater, mars. J. Geophys. Res. Planets 120, 495–514. doi:10.1002/2014JE004737
Freissinet, C., Knudson, C. A., Graham, H. V., Lewis, J. M. T., Lasue, J., McAdam, A. C., et al. (2020). Benzoic acid as the preferred precursor for the chlorobenzene detected on mars: insights from the unique cumberland analog investigation. Planet. Sci. J. 1, 41. doi:10.3847/PSJ/aba690
French, K. L., Hallmann, C., Schoon, P. L., Zumberge, J. A., Hoshino, Y., Peters, C. A., et al. (2015). Reappraisal of hydrocarbon biomarkers in Archean rocks. Proc. Natl. Acad. Sci. 112, 5915–5920. doi:10.1073/pnas.1419563112
Gates, K. S. (2009). An overview of chemical processes that damage cellular DNA: spontaneous hydrolysis, alkylation, and reactions with radicals. Chem. Res. Toxicol. 22, 1747–1760. doi:10.1021/tx900242k
Gillen, C., Jeancolas, C., McMahon, S., and Vickers, P. (2023). The call for a new definition of biosignature. Astrobiology 23, 1228–1237. doi:10.1089/ast.2023.0010
Goordial, J., Davila, A., Lacelle, D., Pollard, W., Marinova, M. M., Greer, C. W., et al. (2016). Nearing the cold-arid limits of microbial life in permafrost of an upper dry valley, Antarctica. ISME J. 10, 1613–1624. doi:10.1038/ismej.2015.239
Grotheer, H., Robert, A. M., Greenwood, P. F., and Grice, K. (2015). Stability and hydrogenation of polycyclic aromatic hydrocarbons during hydropyrolysis (HyPy) – relevance for high maturity organic matter. Org. Geochem. 86, 45–54. doi:10.1016/j.orggeochem.2015.06.007
Grotzinger, J. P., Sumner, D. Y., Kah, L. C., Stack, K., Gupta, S., Edgar, L., et al. (2014). A habitable fluvio-lacustrine environment at yellowknife bay, Gale Crater, mars. Science 343, 1242777. doi:10.1126/science.1242777
Hecht, M. H., Kounaves, S. P., Quinn, R. C., West, S. J., Young, S. M. M., Ming, D. W., et al. (2009). Detection of perchlorate and the soluble chemistry of martian soil at the Phoenix lander site. Science 325, 64–67. doi:10.1126/science.1172466
Horneck, G. (2000). The microbial world and the case for Mars. Planet. Space Sci. 48, 1053–1063. doi:10.1016/S0032-0633(00)00079-9
Jiang, S., Zhuang, J., Wang, C., Li, J., and Yang, W. (2012). Highly efficient adsorption of DNA on Fe3+–iminodiacetic acid modified silica particles. Colloids Surfaces A Physicochem. Eng. Aspects 409, 143–148. doi:10.1016/j.colsurfa.2012.05.051
Klein, H. P. (1978). The Viking biological experiments on Mars. Icarus 34, 666–674. doi:10.1016/0019-1035(78)90053-2
Klein, H. P., Lederberg, J., Rich, A., Horowitz, N. H., Oyama, V. I., and Levin, G. V. (1976). The viking mission search for life on mars. Nature 262, 24–27. doi:10.1038/262024a0
Kopacz, N., Corazzi, M. A., Poggiali, G., von Essen, A., Kofman, V., Fornaro, T., et al. (2023). The photochemical evolution of polycyclic aromatic hydrocarbons and nontronite clay on early Earth and Mars. Icarus 394, 115437. doi:10.1016/j.icarus.2023.115437
Kral, T. A., Goodhart, T. H., Harpool, J. D., Hearnsberger, C. E., McCracken, G. L., and McSpadden, S. W. (2016). Sensitivity and adaptability of methanogens to perchlorates: implications for life on Mars. Planet. Space Sci. 120, 87–95. doi:10.1016/j.pss.2015.11.014
Levin, G. V., and Straat, P. A. (1977). Recent results from the viking labeled release experiment on mars. J. Geophys. Res. 82, 4663–4667. doi:10.1029/JS082i028p04663
Li, Y., Dick, W. A., and Tuovinen, O. H. (2004). Fluorescence microscopy for visualization of soil microorganisms—a review. Biol. Fertil. Soils 39, 301–311. doi:10.1007/s00374-004-0722-x
Madrid, R. E., Felice, C. J., and Valentinuzzi, M. E. (1999). Automatic on-line analyser of microbial growth using simultaneous measurements of impedance and turbidity. Med. & Biol. Eng. & Comput. 37, 789–793. doi:10.1007/BF02513383
Marshall, C. P., Love, G. D., Snape, C. E., Hill, A. C., Allwood, A. C., Walter, M. R., et al. (2007). Structural characterization of kerogen in 3.4Ga archaean cherts from the pilbara craton, western Australia. Precambrian Res. 155, 1–23. doi:10.1016/j.precamres.2006.12.014
McKay, D. S., Gibson, E. K., Thomas-Keprta, K. L., Vali, H., Romanek, C. S., Clemett, S. J., et al. (1996). Search for past life on mars: possible relic biogenic activity in martian meteorite ALH84001. Science 273, 924–930. doi:10.1126/science.273.5277.924
McMahon, S. (2019). Earth’s earliest and deepest purported fossils may be iron-mineralized chemical gardens. Proc. R. Soc. B Biol. Sci. 286, 20192410. doi:10.1098/rspb.2019.2410
McMahon, S., and Cosmidis, J. (2021). False biosignatures on Mars: anticipating ambiguity. J. Geol. Soc. 179, jgs2021–050. doi:10.1144/jgs2021-050
Melendez, I., Grice, K., and Schwark, L. (2013). Exceptional preservation of Palaeozoic steroids in a diagenetic continuum. Sci. Rep. 3, 2768. doi:10.1038/srep02768
Michalski, J. R., Onstott, T. C., Mojzsis, S. J., Mustard, J., Chan, Q. H. S., Niles, P. B., et al. (2018). The Martian subsurface as a potential window into the origin of life. Nat. Geosci. 11, 21–26. doi:10.1038/s41561-017-0015-2
Millan, M., Szopa, C., Buch, A., Coll, P., Glavin, D. P., Freissinet, C., et al. (2016). In situ analysis of martian regolith with the SAM experiment during the first mars year of the MSL mission: identification of organic molecules by gas chromatography from laboratory measurements. Planet. Space Sci. 129, 88–102. doi:10.1016/j.pss.2016.06.007
Millan, M., Williams, A. J., McAdam, A. C., Eigenbrode, J. L., Steele, A., Freissinet, C., et al. (2022). Sedimentary organics in glen torridon, Gale Crater, mars: results from the SAM instrument suite and supporting laboratory analyses. J. Geophys. Res. Planets 127, e2021JE007107. doi:10.1029/2021JE007107
Mojarro, A., Ruvkun, G., Zuber, M. T., and Carr, C. E. (2017). Nucleic acid extraction from synthetic mars analog soils for in situ life detection. Astrobiology 17, 747–760. doi:10.1089/ast.2016.1535
NASEM (2022). Origins, Worlds, and life: a decadal strategy for planetary science and astrobiology 2023-2032. Washington, D.C.: National Academies Press.
Navarro-González, R., Rainey, F. A., Molina, P., Bagaley, D. R., Hollen, B. J., Rosa, J. de la, et al. (2003). Mars-like soils in the Atacama Desert, Chile, and the dry limit of microbial life. Science 302, 1018–1021. doi:10.1126/science.1089143
Neveu, M., Hays, L. E., Voytek, M. A., New, M. H., and Schulte, M. D. (2018). The ladder of life detection. Astrobiology 18, 1375–1402. doi:10.1089/ast.2017.1773
Nutman, A. P., Bennett, V. C., Friend, C. R. L., Van Kranendonk, M. J., and Chivas, A. R. (2016). Rapid emergence of life shown by discovery of 3,700-million-year-old microbial structures. Nature 537, 535–538. doi:10.1038/nature19355
Ourisson, G., and Albrecht, P. (1992). Hopanoids. 1. Geohopanoids: the most abundant natural products on Earth? Acc. Chem. Res. 25, 398–402. doi:10.1021/ar00021a003
Ourisson, G., Albrecht, P., and Rohmer, M. (1979). The Hopanoids: palaeochemistry and biochemistry of a group of natural products. Pure Appl. Chem. 51, 709–729. doi:10.1351/pac197951040709
Preston, L. J., and Dartnell, L. R. (2014). Planetary habitability: lessons learned from terrestrial analogues. Int. J. Astrobiol. 13, 81–98. doi:10.1017/S1473550413000396
Richards, J. C. S., Jason, A. C., Hobbs, G., Gibson, D. M., and Christie, R. H. (1978). Electronic measurement of bacterial growth. J. Phys. E Sci. Instrum. 11, 560–568. doi:10.1088/0022-3735/11/6/017
Rieder, R., Economou, T., Wänke, H., Turkevich, A., Crisp, J., Brückner, J., et al. (1997). The chemical composition of martian soil and rocks returned by the mobile alpha proton X-ray spectrometer: preliminary results from the X-ray mode. Science 278, 1771–1774. doi:10.1126/science.278.5344.1771
Ruff, S. W., Campbell, K. A., Van Kranendonk, M. J., Rice, M. S., and Farmer, J. D. (2020). The case for ancient hot springs in gusev crater, mars. Astrobiology 20, 475–499. doi:10.1089/ast.2019.2044
Ruff, S. W., and Farmer, J. D. (2016). Silica deposits on Mars with features resembling hot spring biosignatures at El Tatio in Chile. Nat. Commun. 7, 13554. doi:10.1038/ncomms13554
Rummel, J. D., Beaty, D. W., Jones, M. A., Bakermans, C., Barlow, N. G., Boston, P. J., et al. (2014). A new analysis of mars “special regions”: findings of the second MEPAG special regions science analysis group (SR-SAG2). Astrobiology 14, 887–968. doi:10.1089/ast.2014.1227
Scheller, E. L., Hollis, J. R., Cardarelli, E. L., Steele, A., Beegle, L. W., Bhartia, R., et al. (2022). Aqueous alteration processes in Jezero crater, Mars−implications for organic geochemistry. Science 378, 1105–1110. doi:10.1126/science.abo5204
Schulze-Makuch, D., Irwin, L. N., Lipps, J. H., LeMone, D., Dohm, J. M., and Fairén, A. G. (2005). Scenarios for the evolution of life on Mars. J. Geophys. Res. 110, 2005JE002430. doi:10.1029/2005JE002430
Schwenzer, S. P., and Kring, D. A. (2009). Impact-generated hydrothermal systems capable of forming phyllosilicates on Noachian Mars. Geology 37, 1091–1094. doi:10.1130/G30340A.1
Sharma, S., Roppel, R. D., Murphy, A. E., Beegle, L. W., Bhartia, R., Steele, A., et al. (2023). Diverse organic-mineral associations in Jezero crater, Mars. Nature 619, 724–732. doi:10.1038/s41586-023-06143-z
Shcherbakova, V., Oshurkova, V., and Yoshimura, Y. (2015). The effects of perchlorates on the permafrost methanogens: implication for autotrophic life on mars. Microorganisms 3, 518–534. doi:10.3390/microorganisms3030518
Soffen, G. A., and Snyder, C. W. (1976). The first viking mission to mars. Science 193, 759–766. doi:10.1126/science.193.4255.759
Song, Y., Zhang, H., Chon, C. H., Chen, S., Pan, X., and Li, D. (2010). Counting bacteria on a microfluidic chip. Anal. Chim. Acta 681, 82–86. doi:10.1016/j.aca.2010.09.035
Squyres, S. W., Knoll, A. H., Arvidson, R. E., Clark, B. C., Grotzinger, J. P., Jolliff, B. L., et al. (2006). Two years at meridiani planum: results from the opportunity rover. Science 313, 1403–1407. doi:10.1126/science.1130890
Stern, J. C., Malespin, C. A., Eigenbrode, J. L., Webster, C. R., Flesch, G., Franz, H. B., et al. (2022). Organic carbon concentrations in 3.5-billion-year-old lacustrine mudstones of Mars. Proc. Natl. Acad. Sci. 119, e2201139119. doi:10.1073/pnas.2201139119
Sturt, H. F., Summons, R. E., Smith, K., Elvert, M., and Hinrichs, K.-U. (2004). Intact polar membrane lipids in prokaryotes and sediments deciphered by high-performance liquid chromatography/electrospray ionization multistage mass spectrometry—new biomarkers for biogeochemistry and microbial ecology. Rapid Commun. Mass Spectrom. 18, 617–628. doi:10.1002/rcm.1378
Sugitani, K., Grey, K., Nagaoka, T., Mimura, K., and Walter, M. R. (2009). Taxonomy and biogenicity of archaean spheroidal microfossils (ca. 3.0Ga) from the mount goldsworthy–mount grant area in the northeastern pilbara craton, western Australia. Precambrian Res. 173, 50–59. doi:10.1016/j.precamres.2009.02.004
Summons, R. E., Amend, J. P., Bish, D., Buick, R., Cody, G. D., Des Marais, D. J., et al. (2011). Preservation of martian organic and environmental records: final report of the mars biosignature working group. Astrobiology 11, 157–181. doi:10.1089/ast.2010.0506
Summons, R. E., Welander, P. V., and Gold, D. A. (2022). Lipid biomarkers: molecular tools for illuminating the history of microbial life. Nat. Rev. Microbiol. 20, 174–185. doi:10.1038/s41579-021-00636-2
Tarnas, J. D., Mustard, J. F., Sherwood Lollar, B., Bramble, M. S., Cannon, K. M., Palumbo, A. M., et al. (2018). Radiolytic H2 production on Noachian Mars: implications for habitability and atmospheric warming. Earth Planet. Sci. Lett. 502, 133–145. doi:10.1016/j.epsl.2018.09.001
Vestal, J. R., and White, D. C. (1989). Lipid analysis in microbial ecology: quantitative approaches to the study of microbial communities. BioScience 39, 535–541. doi:10.2307/1310976
Wade, W. (2002). Unculturable bacteria--the uncharacterized organisms that cause oral infections. J. R. Soc. Med. 95, 81–83. doi:10.1177/014107680209500207
Warren-Rhodes, K. A., Rhodes, K. L., Pointing, S. B., Ewing, S. A., Lacap, D. C., Gómez-Silva, B., et al. (2006). Hypolithic cyanobacteria, dry limit of photosynthesis, and microbial ecology in the hyperarid Atacama Desert. Microb. Ecol. 52, 389–398. doi:10.1007/s00248-006-9055-7
Westall, F., and Cavalazzi, B. (2011). Biosignatures in rocks. Encycl. Geobiol., 189–201. doi:10.1007/978-1-4020-9212-1_36
Westall, F., Foucher, F., Bost, N., Bertrand, M., Loizeau, D., Vago, J. L., et al. (2015). Biosignatures on mars: what, where, and how? Implications for the search for martian life. Astrobiology 15, 998–1029. doi:10.1089/ast.2015.1374
Westall, F., Loizeau, D., Foucher, F., Bost, N., Betrand, M., Vago, J., et al. (2013). Habitability on mars from a microbial point of view. Astrobiology 13, 887–897. doi:10.1089/ast.2013.1000
Wierzchos, J., Ríos, A. L., and Ascaso, C. (2012). Microorganisms in desert rocks: the edge of life on Earth. doi:10.13039/501100004837
Wilson, M. J., Weightman, A. J., and Wade, W. G. (1997). Applications of molecular ecology in the characterization of uncultured microorganisms associated with human disease. Rev. Res. Med. Microbiol. 8, 91–102. doi:10.1097/00013542-199704000-00005
Keywords: astrobiology, biosignature, geobioloigy, instrumentation, Mars biosphere
Citation: Dzurilla KA and Teece BL (2024) Discriminating between extinct and extant life detection: implications for future Mars missions. Front. Astron. Space Sci. 11:1452362. doi: 10.3389/fspas.2024.1452362
Received: 20 June 2024; Accepted: 12 August 2024;
Published: 23 August 2024.
Edited by:
Jennifer Claire Stern, National Aeronautics and Space Administration, United StatesReviewed by:
Alfonso F. Davila, National Aeronautics and Space Administration, United StatesMihaela Glamoclija, Rutgers University, United States
Copyright © 2024 Dzurilla and Teece. This is an open-access article distributed under the terms of the Creative Commons Attribution License (CC BY). The use, distribution or reproduction in other forums is permitted, provided the original author(s) and the copyright owner(s) are credited and that the original publication in this journal is cited, in accordance with accepted academic practice. No use, distribution or reproduction is permitted which does not comply with these terms.
*Correspondence: Katherine A. Dzurilla, a2F0aGVyaW5lLmEuZHp1cmlsbGFAanBsLm5hc2EuZ292; Bronwyn L. Teece, YnJvbnd5bi50ZWVjZUBqcGwubmFzYS5nb3Y=
†These authors have contributed equally to this work and share first authorship