- 1INAF-Osservatorio Astrofisico di Arcetri, Florence, Italy
- 2Max-Planck-Institute for Extraterrestrial Physics, Garching, Germany
- 3Laboratoire d'Etudes du Rayonnement et de la Matière en Astrophysique et Atmosphères, Observatoire de Paris, Meudon, France
The chemistry of phosphorus (31P) in space is particularly significant due to the key role it plays in biochemistry on Earth. Utilising radio and infrared spectroscopic observations, several key phosphorus-containing molecules have been detected in interstellar clouds, circumstellar shells, and even extragalactic sources. Among these, phosphorus nitride (PN) was the first P-bearing molecule detected in space, and still is the species detected in the largest number of sources. Phosphorus oxide (PO) and phosphine (
1 Introduction
The study of interstellar chemistry, that is the chemistry occurring in the interstellar medium (ISM), is an important but difficult task owing to the huge variety of physical conditions and chemical composition in the ISM. In particular, molecular clouds, that are interstellar clouds where hydrogen is mostly in the form of
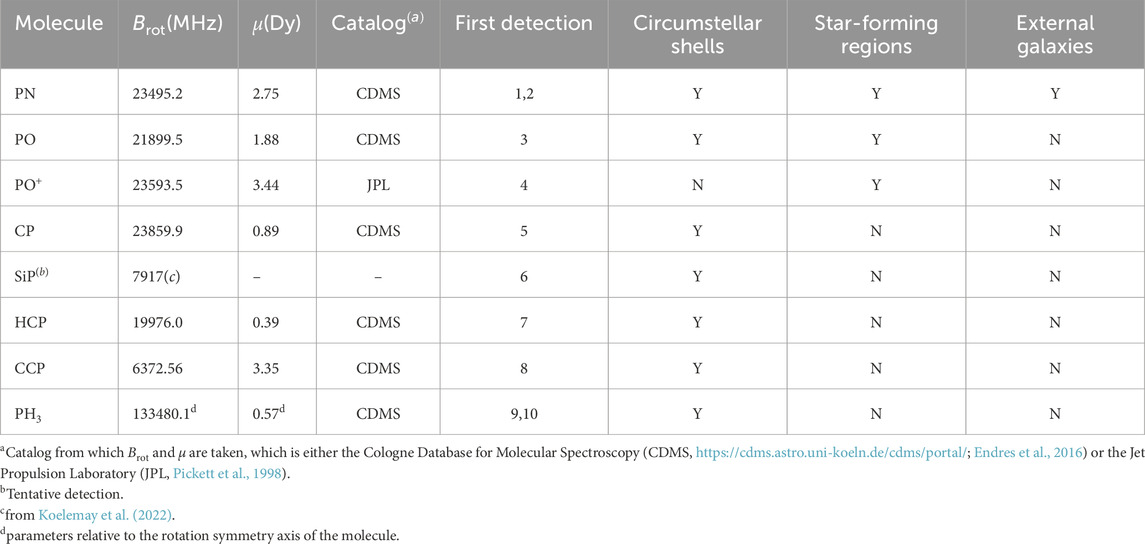
Table 1. Phosphorus-bearing molecules detected in the ISM.
All P-bearing species in Table 1, except
The objective of this article is to review the observational studies on P-bearing species in the ISM, and discuss their implications in our current understanding of the astrochemistry of this essential biogenic element. Emphasis is particularly given to observations towards star-forming regions, and their implications for pre-biotic chemistry. Even though the focus is on observations, a brief overview on how they fueled theoretical models and laboratory experiments, and vice versa, will be also presented. The structure of the article is the following: Section 2 briefly discusses the cosmic origin of P, and its measured abundance in the diffuse ISM; some basic processes in the ISM relevant for P chemistry are presented in Sections 3, 4 we give a brief overview of the detection techniques used to identify and analyse P-bearing species; in Section 5, the observations of the P-bearing molecules identified in the ISM are reviewed; the input from laboratory experiments and the implications for astrochemical models are discussed in Sections 6, 7, respectively. Conclusions and future perspectives are presented in Section 8.
2 The cosmic origin of phosphorus and its abundance in diffuse gas
Cosmic abundances of all elements are produced through three main mechanisms: Big Bang nucleosynthesis, stellar nucleosynthesis, and neutron capture. The latter occurs mostly in environments with high fluxes of neutrons, such as in supernova (SN) explosions or in the interior of red giants. The main phosphorus isotope, 31P, is traditionally believed to be mainly formed in massive
As previously said (Section 1), the present-day Solar photosphere abundance of phosphorus is
3 Basic astrochemical processes involving phosphorus in the ISM
Two types of chemical processes are invoked to explain the formation of molecules in the ISM: gas-phase processes and grain-surface processes. In this section, we give an overview of the gas-phase and grain-surface astrochemical reactions believed to be important for the formation of phosphorus molecules. Books where these processes are described in detail are, for example, Duley and Williams (1985) and Yamamoto (2017).
3.1 Gas-phase chemistry
Gas-phase reactions occur spontaneously if the Gibbs energy,
where
Once
Many neutral-neutral two-body reactions possess activation barriers exceeding
for PO formation, and:
for PN formation. The rate coefficients of all these reactions (Equation 8–11). In the original phosphorus network of Millar (1991), only
3.2 Surface chemistry
Surface chemistry, namely, the synthesis of molecules on the surfaces of dust grains, is extremely important in the ISM because dust grains can help chemical reactions to occur on their surfaces in many ways, playing the role of reactant concentrators, reactant suppliers, chemical catalysts, and third bodies (e.g., Draine and Li, 2001). Surface chemistry was invoked to explain the large amount of
Among surface processes relevant for P chemistry, hydrogenation of atomic P is considered the most relevant (Chantzos et al., 2020) to form sequentially (Equation 12):
The final product,
4 Detection techniques
As most of any other molecule (e.g., McGuire, 2022), the detection of P-bearing species was obtained using radio astronomy techniques in the centimeter and (sub-)millimeter wavelength range. Molecules can emit and absorb radiation via electronic, vibrational, and rotational transitions (e.g., Townes and Schawlow, 1975). However, at the typical conditions of the molecular gas in the ISM (see Section 1), basically only rotational levels in the ground electronic and vibrational states are populated. Thus, the molecular emission occurs via transitions between these levels only, whose wavelengths fall in the radio and (sub-)millimeter portion of the electromagnetic spectrum. This can be shown deriving the energy of the levels of the rotational spectrum, which depends on the geometrical structure of the molecule. All P-bearing molecules detected so far (Table 1), except
where
and it is hence inversely proportional to
thus also inversely proportional to
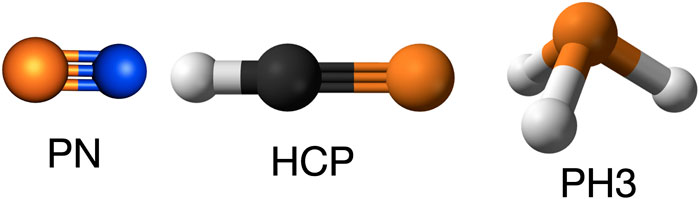
Figure 1. Geometrical structure of PN, HCP, and
In single-dish radio telescopes, usually the intensity of a molecular transition is expressed in temperature units through the main beam brightness temperature,
5 Observations of phosphorus-bearing molecules in the ISM
The first molecule containing P detected in space is phosphorus nitride, PN, towards three star-forming regions: Ori (KL), W51, and Sgr B2, by observations of its
5.1 Star-forming regions
The first detection of PN in Ori (KL) already suggested that the molecule probably could not be formed in cold gas, because the measured fractional abundance, [P/H]
After about 20 years from the first discoveries, in 2011 Yamaguchi et al. (2011) reported the first detection in a low-mass star-forming region of a phosphorus molecule: PN in the
In the same year as the PN survey of Fontani et al. (2016), Rivilla et al. (2016) reported the first detection of phosphorus oxide (PO) in two star-forming regions, W51 and W3(OH) (both luminous high-mass objects), followed shortly after by Lefloch et al. (2016) towards L1157 B1. Such new detections were particularly important for many reasons. First, PO was predicted to be the most abundant P-bearing species in laboratory experiments (Thorne et al., 1984). Second, it is the basic bond of phosphates, and hence understanding its formation and survival in the ISM is crucial for astrobiology. Figure 2 shows the lines of PO detected in W51 and W3(OH) by Rivilla et al. (2016). The abundance ratio PO/PN measured by Rivilla et al. (2016) and Lefloch et al. (2016) is
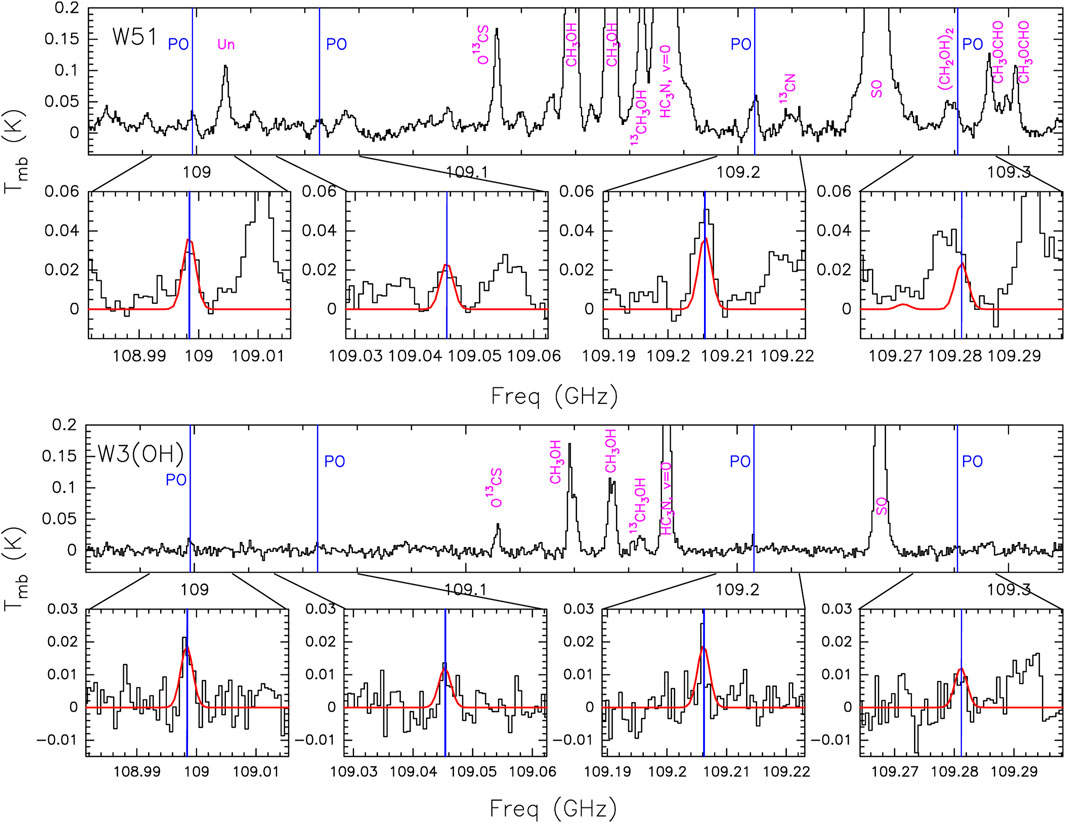
Figure 2. Top panels: Spectrum observed at 3 mm toward W51. The PO transitions are indicated with blue vertical lines. Under the spectrum, zoom-in views of the PO transitions are highlighted. The red line is the fit in local thermodynamic equilibrium with an excitation temperature of 35 K and an assumed source size of
Rivilla et al. (2018) shed more light on the role of shocks in the formation and destruction of PN and PO observing seven star-forming regions in the Galactic Centre, characterised by different types of chemistry. They detected five out of seven regions in PN and only one in PO, and suggested an efficient formation of these molecules in shock-dominated regions upon grain sputtering, and an efficient destruction in radiation-dominated (UV/X-rays/cosmic ray) regions. Such photo-destruction would change the PO/PN ratio, since PO is predicted to be destroyed more efficiently than PN by UV photons (Jiménez-Serra et al., 2018). We will come back to this point when discussing the extragalactic detection of PN (Section 5.3.1). Mininni et al. (2018) and Fontani et al. (2019) followed-up the PN
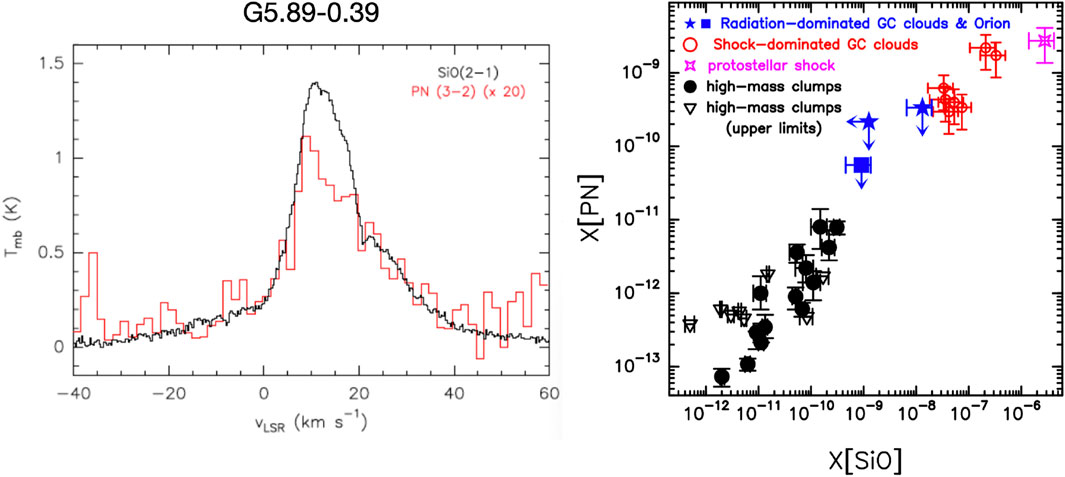
Figure 3. Left panel: Spectrum of PN (3–2) (red histogram; multiplied by a factor of 20) and SiO (2–1) (black histogram) lines measured towards the G5.89–0.39 UCHII region. Taken from Mininni et al. (2018). Right panel: Fractional abundance of PN,
From the side of low-mass star formation regions, Bergner et al. (2019) detected PN and PO in multiple lines for the first time in the envelope of a low-mass protostar: B1-a. The study was then followed-up in seven similar protostars, all of them known to be associated with an outflow (Wurmser and Bergner, 2022). The multi-line analysis performed in these studies indicates that also in the low-mass regime: (1) both the PN and the PO emission are likely sub-thermally excited; (2) the line profiles of PN and PO resemble those of the shock tracers SiO,
The tight association between PN, PO, and shock emission was robustly confirmed by high-angular resolution studies. Rivilla et al. (2020), Bergner et al. (2022) and Fontani et al. (2024) mapped at high-angular resolution the PN and PO emission towards three protostars with different properties: the high-mass protostellar object AFGL 5142 (Rivilla et al., 2020), the low-mass class I protostar B1-a (Bergner et al., 2022), and the prototypical chemically rich hot core G31.41 + 0.31 (Fontani et al., 2024). All sources are driving outflows associated with typical shock tracers: SiO, SO, and
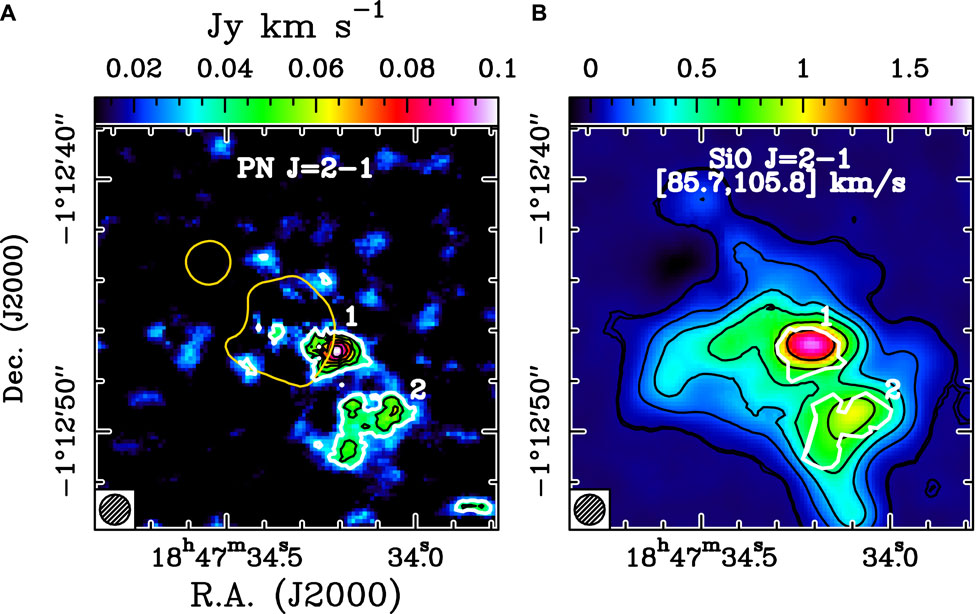
Figure 4. Intensity maps of PN and SiO integrated in velocity towards G31.41 + 0.31 (Fontani et al., 2024), obtained with ALMA. (A) PN
The third (and last so far) P-bearing molecule detected in a star-forming regions,
A key ingredient in phosphorus chemistry is certainly phosphine,
Finally, although phosphorus is thought to be mainly produced in massive stars and injected in the environment through SN explosions (see Section 2), P-bearing molecules were detected also in WB89-621, a star-forming region located in the outskirt of the Galactic disk (Koelemay et al., 2023) where the presence of supernovae is extremely rare. WB89-621 is at a Galactocentric distance of 22.6 kpc (Blair et al., 2008), even though a more recent estimate place it at
5.2 Evolved stars
Even though this review is mostly focussed on star-forming regions, a significant contribution on the interstellar chemistry of phosphorus was given by evolved stars. As said in Section 1, all phosphorus molecules found in the ISM except PN and
For 10 years, CP remained the only phosphorus species found in a circumstellar shell, until Cernicharo et al. (2000) detected PN in the same object. Then, a boost of new detections of phosphorus molecules happened in 2007 and 2008. Again towards IRC+10216, the third P-bearing species, HCP, was detected in multiple lines (Agúndez et al., 2007). They are shown in the left panel of Figure 5. The line profiles indicated again that this species is confined to the inner envelope, probably near the stellar photosphere.As discussed in Agúndez et al. (2007), the gas chemical composition of the atmosphere of cool stars like IRC+10216 is in thermal equilibrium (TE). The prediction of TE models is that up to 2-3 stellar radii the dominant gaseous P-bearing molecule depends on the C/O relative ratio. These predictions are shown in the right panel of Figure 5. In C-rich stars like IRC+10216, all gaseous P is in the form of HCP, which should have an abundance more than three orders of magnitude higher than any other phosphorus molecule. However, the observed HCP line intensities suggest already a significant depletion of HCP (due to either freeze-out or dissociation) already at a few stellar radii. That the emission of phosphorus molecules is associated with inner shells of circumstellar envelopes was further confirmed by the first PO detection, the fourth P-bearing molecule seen in the ISM, reported by Tenenbaum et al. (2007) toward the oxygen-rich supergiant star VY Canis Majoris (VY CMa). The line profiles of the detected PO and PN transitions suggest an origin again in the inner part of a spherical wind known to be present in VY CMa (Ziurys et al., 2007). The same conclusion was drawn by observations of Milam et al. (2008) towards the C-rich protoplanetary nebula CRL 2688, who found that HCP and PN should be produced in the inner shells, and CP is likely a product of HCP photodissociation at larger radii. However, Tenenbaum et al. (2007) measured comparable abundances of PN and PO in VY CMa, which is at odds with the prediction of circumstellar chemistry models for O-rich stars: considering only processes in TE, these models predict a PO abundance much higher (
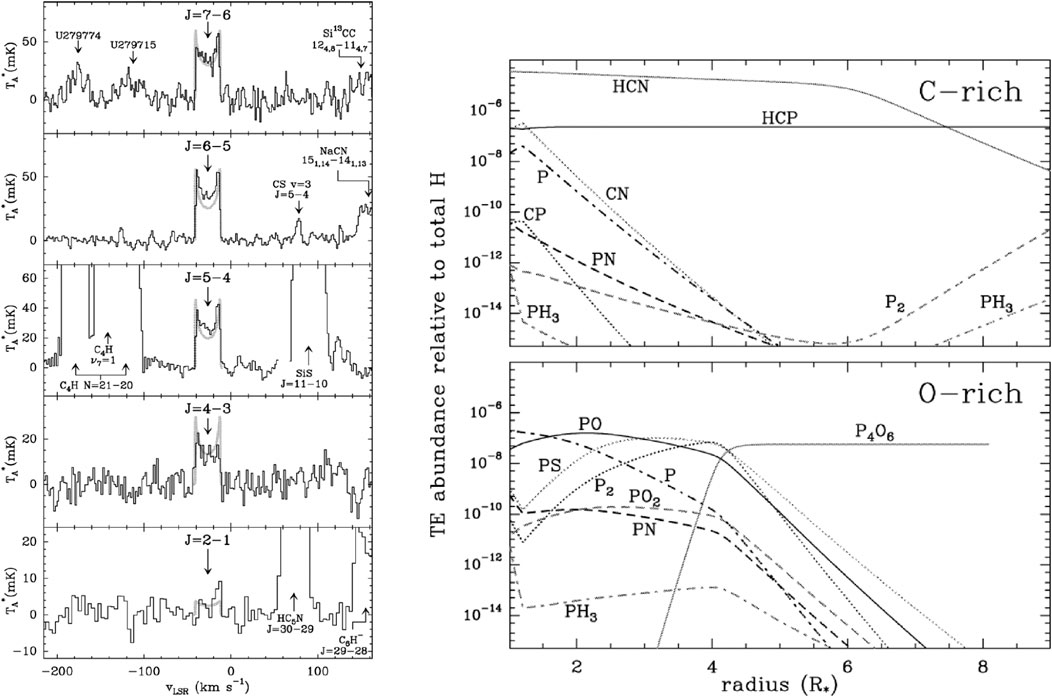
Figure 5. Left panel: rotational transitions
More recently, new identification of PO and PN towards the circumstellar shells of several O-rich stars were reported: the Asymptotic Giant Branch (AGB) stars TX Cam and R Cas (Ziurys et al., 2018), the supergiant NML Gyg (Ziurys et al., 2018), and the Mira-type variable star IK Tau (De Beck et al., 2013). Both the observed compact emission of PN and PO (De Beck et al., 2013), and the predictions of Non-TE models applied to a spherical radial distribution of the gas, suggest that the two molecules are always formed near the stellar photosphere, perhaps with their abundances enhanced by shocks (Ziurys et al., 2018). Moreover, the observed molecular abundances indicate gas phase carriers of P even more abundant than previously thought. Another important piece of the puzzle was added by the detection of the fifth and sixth phosphorus molecules in the ISM: CCP and
5.3 Other environments
5.3.1 External galaxies
The first, and so far unique, phosphorus molecule detected in an external galaxy is PN towards the nearby starburst Galaxy NGC 253 (Haasler et al., 2022), in the framework of the ALMA Comprehensive High-resolution Extragalactic Molecular Inventory (ALCHEMI) project (Martín et al., 2021). The PN emission arises from two giant molecular clouds in the galaxy, and it is enhanced towards the emission peak of the dust thermal continuum emission (Haasler et al., 2022). Simultaneous SiO observations confirm that also in these extragalactic clouds the abundances of PN and SiO are correlated, poiting to a shocked origin of PN as in Milky Way clouds (e.g., Lefloch et al., 2016; Rivilla et al., 2018; 2020; Fontani et al., 2019; Bergner et al., 2022). However, while in Galactic clouds PO is found to be always more abundant than PN, upper limits on PO abundances measured towards NGC 253 suggest that the PN abundance is comparable to or larger than that of PO. Because
5.3.2 Solar system objects and implications for astrobiology
In the Solar system, phosphorus in the form of
6 Input from laboratory experiments and computational chemistry
The synergy between observations, chemical models, laboratory experiments, and computational chemistry made it possible the identification of the aforementioned phosphorus molecules in the ISM. Laboratory experiments, in particular, were essential to identify the new species in the observed spectra through calculation of their spectroscopic parameters. As stated in Section 4, almost the totality of the gas-phase species in the ISM was identified through their rotational transitions at centimeter and (sub-)millimeter wavelengths, owing to the low temperatures of the ISM (see Section 4). Therefore, rotational spectroscopy was, and still is, of paramount importance also for the identification of phosphorus molecules. Even though this review article focusses on observations, we briefly summarise some spectroscopy works that allowed to identify the most relevant P-bearing species in centimeter and (sub-)millimeter spectra.
Several rotational transitions in the ground and first four vibrationally excited state of PN, the molecule detected so far in the highest number of sources, were first measured by Wyse et al. (1972) through a high temperature, microwave spectrometer. In the same year, Hoeft et al. (1972) resolved the hyperfine structure of the
Finally, computational simulations were relevant to understand, from a theoretical point of view, some key aspects of chemical reactions involving P (like binding energies, reaction energies, or activation barriers) particularly challenging to study in the laboratory due to the high reactivity of phosphorus radicals. For example, Molpeceres and Kästner (2021) confirmed that P can be easily hydrogenated via subsequent additions of H on cold dust grain analogues, such as ice mantles. However, using a novel methodology based on a neural network interatomic potential, Molpeceres et al. (2023) studied the surface reaction P + H
7 Implications for astrochemical models: what are the main phosphorus reservoirs?
Although the observational constraints obtained so far are important “food” for chemical models, the reactions leading to the formation of the detected phosphorus species are far from clear. Moreover, a major question still remains unanswered: what are the main phosphorus reservoirs in the molecular ISM? We will briefly summarise in this chapter the most relevant steps performed by chemical modelling to answer this question in star-forming regions (7.1) and evolved stars (7.2), triggered by the observational results presented in Section 5.
7.1 Star-forming regions
The fact that in all star-forming regions studied so far both PN and PO emission arise from shocked material and is well correlated with the emission of typical shock tracers (5), indicates very clearly dust grains as the main source of P. However, which is the main carrier in dust grains is still debated. Jiménez-Serra et al. (2018) proposed
The analysis of the spatial distribution of the PN and PO emission in B1-a carried out by Bergner et al. (2022) suggested that there is a phosphorus reservoir in dust grains more volatile than silicate grains but less volatile than simple ice mantles (such as, for example,
Jiménez-Serra et al. (2018) investigated how different energetic conditions can influence the P chemistry. They concluded that models with strong UV illumination predict a strong molecular dissociation, in line with the non-detection of PO in giant molecular clouds in NGC 253 (Haasler et al., 2022) and in the Galactic Centre (Rivilla et al., 2018). Models with enhanced
Because the three reactions 16 have similar rate coefficients, in cold gas a higher PO/PN abundance would just be due to the higher O over N elemental abundance. At higher temperatures the network becomes more complicated and more reactions, including destruction of PH and
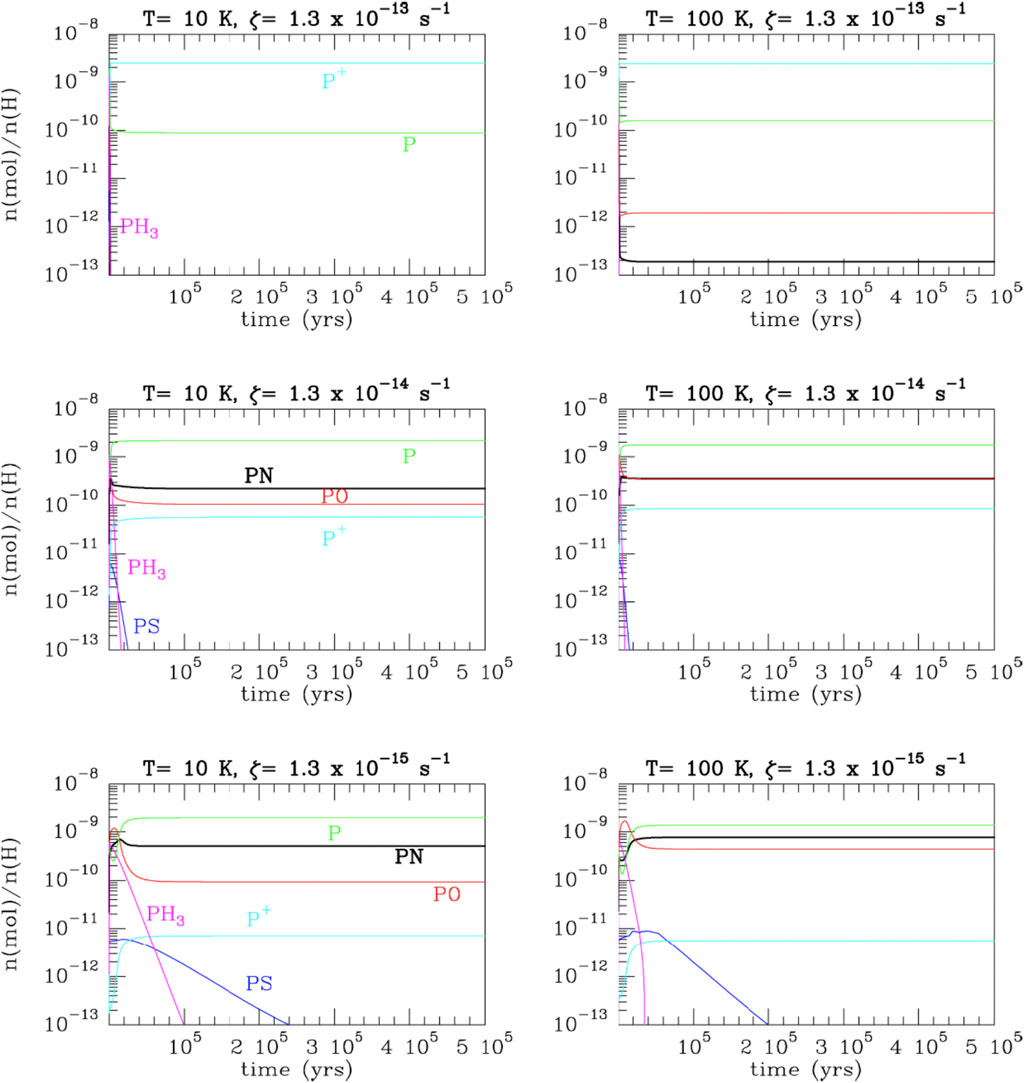
Figure 6. Evolution of the abundances of P-bearing species as a function of time in a collapsing cloud simulated for a hydrogen density n(H) =
7.2 Evolved stars
As seen in Section 5.2, phosphorus molecules are common in both oxygen- and carbon-rich evolved stars. Agúndez et al. (2007) modelled the envelope of the carbon-rich star IRC+20126 with thermodynamic calculations in TE conditions, and found that all phosphorus should be in the form of HCP (see Figure 5). A similar prediction was obtained by Turner et al. (1990), before the first detection of HCP. HCP should arise from a inner shell (up to 2-3 radii) around the stellar photosphere, as confirmed by observations (Agúndez et al., 2007). On the other hand, in an O-rich envelope the main phosphorus carriers would be PO, PS, and
Most of the models developed so far assume a steady, spherically-symmetric, outflow with constant expansion velocity and mass loss rate. MacKay and Charnley (2001) modelled both C- and O-rich circumstellar envelopes in this way, assuming that the dominant forms of P at the fiducial inner radius are HCP and PS for C-rich and O-rich, respectively. They predicted that the only species with abundance greater than
8 Summary, conclusions and future prospects
Understanding the formation of phosphorus molecules is of great importance in astrochemistry, because P is a crucial element for the development of life as we know it. Phosphorus is mostly created by neutron capture occurring within high-mass stars, and ejected during supernova explosions, even though recent works have invoked other sources of phosphorus to explain the detection of P-bearing molecules in the outskirt of the Galactic disk. In diffuse clouds, all works performed so far agree that phosphorus is essentially non depleted. Its depletion increases with increasing gas density, although model predictions indicate depletion levels lower than previously thought to reproduce the observed molecular abundances (including upper limits). The high uncertainty in the depletion level, and hence in the initial P elemental gaseous abundance, is certainly still one of the most critical input parameters for chemical models of dense clouds.
The first detections of PN in star-forming regions, obtained many years ago, already suggested that this species is linked to shocks. A significant step forward in the relation between phosphorus chemistry and shocks in star-forming clouds was made in the last decade, thanks to:
• a significant statistical increase of PN detections obtained with single-dish telescopes towards high-mass star-forming regions, which confirmed that PN is correlated with SiO both in the abundances and line shapes in a variety of Galactic environments;
• the first PO detections, which indicated that also this radical is likely a product of shocked gas, and is typically more abundant than PN;
• the first surveys of PN and PO in low-mass star-forming regions, which indicated that even in the low-mass regime PO and PN are linked to shocks. Moreover, their relative ratios are in good agreement with those found in the high-mass regime, indicating similar formation/destruction pathways in low- and high-mass star-formation environments;
• the first high-angular resolution maps of phosphorus molecules, which confirmed that the PN and PO emissions spatially overlap with those of shock tracers;
All these findings point to a solid main carrier of phosphorus in star-forming regions. However, it is still unclear if this is mainly in a volatile or refractory form. Theory indicates that the main volatile form would be
From the side of evolved stars, CP was the first molecule detected in the shell of a C-rich AGB star, followed by PN, HCP, CCP, PO, and
The detection of phosphorus compounds in new sources and new molecules, especially those predicted to be relevant from models such as PS in the envelope of O-rich stars, HCP and CP in diffuse clouds, and PS, HPO, and H
Author contributions
FF: Writing–original draft.
Funding
The author(s) declare that no financial support was received for the research, authorship, and/or publication of this article.
Acknowledgments
FF is grateful to the reviewers for their constructive comments, which helped to improve the original version of the manuscript. FF is also grateful to V. Lattanzi, V. M. Rivilla, and L. Magrini, for useful discussion.
Conflict of interest
The author declares that the research was conducted in the absence of any commercial or financial relationships that could be construed as a potential conflict of interest.
Publisher’s note
All claims expressed in this article are solely those of the authors and do not necessarily represent those of their affiliated organizations, or those of the publisher, the editors and the reviewers. Any product that may be evaluated in this article, or claim that may be made by its manufacturer, is not guaranteed or endorsed by the publisher.
References
Adams, N. G., McIntosh, B. J., and Smith, D. (1990). Production of phosphorus-containing molecules in interstellar clouds. A&A 232, 443.
Agúndez, M., Cernicharo, J., Decin, L., Encrenaz, P., and Teyssier, D. (2014). Confirmation of circumstellar phosphine. ApJL 790, L27. doi:10.1088/2041-8205/790/2/L27
Agúndez, M., Cernicharo, J., and Guélin, M. (2007). Discovery of phosphaethyne (HCP) in space: phosphorus chemistry in circumstellar envelopes. ApJL 662, L91–L94. doi:10.1086/519561
Agúndez, M., Cernicharo, J., Pardo, J. R., Guélin, M., and Phillips, T. G. (2008). Tentative detection of phosphine in IRC +10216. A&A 485, L33–L36. doi:10.1051/0004-6361:200810193
Ahmad, I. K., and Hamilton, P. A. (1995). The fourier transform infrared spectrum of PN. J. Mol. Spectrosc. 169, 286–291. doi:10.1006/jmsp.1995.1022
Altwegg, K., Balsiger, H., Bar-Nun, A., Berthelier, J. J., Bieler, A., Bochsler, P., et al. (2016). Prebiotic chemicals–amino acid and phosphorus–in the coma of comet 67P/Churyumov-Gerasimenko. Sci. Adv. 2, e1600285. e1600285–e1600285. doi:10.1126/sciadv.1600285
Anders, E., and Grevesse, N. (1989). Abundances of the elements: meteoritic and solar. GeCoA 53, 197–214. doi:10.1016/0016-7037(89)90286-X
Anicich, V. G. (1993). A survey of bimolecular ion-molecule reactions for use in modeling the chemistry of planetary atmospheres, cometary comae, and interstellar clouds: 1993 supplement. ApJS 84, 215. doi:10.1086/191752
Asplund, M., Grevesse, N., Sauval, A. J., and Scott, P. (2009). The chemical composition of the Sun. ARA&A 47, 481–522. doi:10.1146/annurev.astro.46.060407.145222
Bailleux, S., Bogey, M., Demuynck, C., Liu, Y., and Walters, A. (2002). Millimeter-wave spectroscopy of PO in excited vibrational states up to v=7. J. Mol. Spectrosc. 216, 465–471. doi:10.1006/jmsp.2002.8665
Bekki, K., and Tsujimoto, T. (2024). Phosphorus enrichment by ONe novae in the galaxy. ApJL 967, L1. doi:10.3847/2041-8213/ad3fb6
Bergner, J. B., Burkhardt, A. M., Öberg, K. I., Rice, T. S., and Bergin, E. A. (2022). First images of phosphorus molecules toward a protosolar analog. ApJ 927, 7. doi:10.3847/1538-4357/ac47a2
Bergner, J. B., Öberg, K. I., Walker, S., Guzmán, V. V., Rice, T. S., and Bergin, E. A. (2019). Detection of phosphorus-bearing molecules toward a solar-type protostar. ApJL 884 (36), L36. doi:10.3847/2041-8213/ab48f9
Bizzocchi, L., Thorwirth, S., Müller, H. S. P., Lewen, F., and Winnewisser, G. (2001). Submillimeter-wave spectroscopy of phosphaalkynes: HCCCP, NCCP, HCP, and DCP. J. Mol. Spectrosc. 205, 110–116. doi:10.1006/jmsp.2000.8234
Blair, S. K., Magnani, L., Brand, J., and Wouterloot, J. G. A. (2008). Formaldehyde in the far outer galaxy: constraining the outer boundary of the galactic habitable zone. Astrobiology 8, 59–73. doi:10.1089/ast.2007.0171
Boogert, A. C. A., Gerakines, P. A., and Whittet, D. C. B. (2015). Observations of the icy universe. ARA&A 53, 541–581. doi:10.1146/annurev-astro-082214-122348
Caffau, E., Bonifacio, P., Faraggiana, R., and Steffen, M. (2011). The Galactic evolution of phosphorus. A&A 532, A98. doi:10.1051/0004-6361/201117313
Calmonte, U., Altwegg, K., Balsiger, H., Berthelier, J. J., Bieler, A., Cessateur, G., et al. (2016). Sulphur-bearing species in the coma of comet 67P/Churyumov-Gerasimenko. MNRAS 462, S253–S273. doi:10.1093/mnras/stw2601
Caselli, P., and Ceccarelli, C. (2012). Our astrochemical heritage. A&A Rev. 20, 56. doi:10.1007/s00159-012-0056-x
Cazaux, S., and Tielens, A. G. G. M. (2004). H2 formation on grain surfaces. ApJ 604, 222–237. doi:10.1086/381775
Cazzoli, G., Cludi, L., and Puzzarini, C. (2006). Microwave spectrum of P 14N and P 15N: spectroscopic constants and molecular structure. J. Mol. Struct. 780, 260–267. doi:10.1016/j.molstruc.2005.07.010
Cazzoli, G., and Puzzarini, C. (2006). The Lamb-dip spectrum of phosphine: the nuclear hyperfine structure due to hydrogen and phosphorus. J. Mol. Spectrosc. 239, 64–70. doi:10.1016/j.jms.2006.05.019
Ceccarelli, C., Codella, C., Balucani, N., Bockelee-Morvan, D., Herbst, E., Vastel, C., et al. (2023). “Organic chemistry in the first phases of solar-type protostars,”. Protostars and planets VII. Editors S. Inutsuka, Y. Aikawa, T. Muto, K. Tomida, and M. Tamura (San Francisco: Astronomical Society of the Pacific), 534, 379.
Cernicharo, J., Guélin, M., and Kahane, C. (2000). A lambda 2 mm molecular line survey of the C-star envelope IRC+10216. A&AS 142, 181–215. doi:10.1051/aas:2000147
Chantzos, J., Rivilla, V. M., Vasyunin, A., Redaelli, E., Bizzocchi, L., Fontani, F., et al. (2020). The first steps of interstellar phosphorus chemistry. A&A 633, A54. doi:10.1051/0004-6361/201936531
Charnley, S. B., and Millar, T. J. (1994). The chemistry of phosphorus in hot molecular cores. MNRAS 270, 570–574. doi:10.1093/mnras/270.3.570
Cherchneff, I. (2006). A chemical study of the inner winds of asymptotic giant branch stars. A&A 456, 1001–1012. doi:10.1051/0004-6361:20064827
Davies, P. B., Neumann, R. M., Wofsy, S. C., and Klemperer, W. (1971). Radio-frequency spectrum of phosphine (PH3). JChPh 55, 3564–3568. doi:10.1063/1.1676614
De Beck, E., Kamiński, T., Patel, N. A., Young, K. H., Gottlieb, C. A., Menten, K. M., et al. (2013). PO and PN in the wind of the oxygen-rich AGB star Ik Tauri. A&A 558, A132. doi:10.1051/0004-6361/201321349
Draine, B. T., and Li, A. (2001). Infrared emission from interstellar dust. I. Stochastic heating of small grains. ApJ 551, 807–824. doi:10.1086/320227
Dréan, P., Demaison, J., Poteau, L., and Denis, J. M. (1996). Rotational spectrum and structure of HCP. J. Mol. Spectrosc. 176, 139–145. doi:10.1006/jmsp.1996.0070
Dufton, P. L., Keenan, F. P., and Hibbert, A. (1986). The abundance of phosphorus in the interstellar medium. A&A 164, 179–183.
Endres, C. P., Schlemmer, S., Schilke, P., Stutzki, J., and Müller, H. S. P. (2016). The Cologne database for molecular spectroscopy, CDMS, in the virtual atomic and molecular data Centre, VAMDC. J. Mol. Spectrosc. 327, 95–104. doi:10.1016/j.jms.2016.03.005
Fernández-Ruz, M., Jiménez-Serra, I., and Aguirre, J. (2023). A theoretical approach to the complex chemical evolution of phosphorus in the interstellar medium. ApJ 956, 47. doi:10.3847/1538-4357/acf290
Fletcher, L. N., Irwin, P. G. J., Teanby, N. A., Orton, G. S., Parrish, P. D., Calcutt, S. B., et al. (2007). The meridional phosphine distribution in Saturn’s upper troposphere from Cassini/CIRS observations. Icarus 188, 72–88. doi:10.1016/j.icarus.2006.10.029
Fletcher, L. N., Orton, G. S., Teanby, N. A., and Irwin, P. G. J. (2009). Phosphine on jupiter and Saturn from Cassini/CIRS. Icarus 202, 543–564. doi:10.1016/j.icarus.2009.03.023
Fontani, F., Colzi, L., Bizzocchi, L., Rivilla, V. M., Elia, D., Beltrán, M. T., et al. (2022). CHEMOUT: CHEMical complexity in star-forming regions of the OUTer Galaxy. I. Organic molecules and tracers of star-formation activity. A&A 660, A76. doi:10.1051/0004-6361/202142923
Fontani, F., Mininni, C., Beltrán, M. T., Rivilla, V. M., Colzi, L., Jiménez-Serra, I., et al. (2024). The GUAPOS project: G31.41+0.31 Unbiased ALMA sPectral Observational Survey. IV. Phosphorus-bearing molecules and their relation to shock tracers. A&A 682, A74. doi:10.1051/0004-6361/202348219
Fontani, F., Rivilla, V. M., Caselli, P., Vasyunin, A., and Palau, A. (2016). Phosphorus-bearing molecules in massive dense cores. ApJL 822, L30. doi:10.3847/2041-8205/822/2/L30
Fontani, F., Rivilla, V. M., van der Tak, F. F. S., Mininni, C., Beltrán, M. T., and Caselli, P. (2019). Origin of the PN molecule in star-forming regions: the enlarged sample. MNRAS 489, 4530–4542. doi:10.1093/mnras/stz2446
Fontani, F., Roueff, E., Colzi, L., and Caselli, P. (2023). The evolution of sulphur-bearing molecules in high-mass star-forming cores. A&A 680, A58. doi:10.1051/0004-6361/202347565
Friedman, S. D., Howk, J. C., Andersson, B. G., Sembach, K. R., Ake, T. B., Roth, K., et al. (2000). [ITAL]Far ultraviolet spectroscopic explorer[/ITAL] observations of interstellar gas toward the large magellanic cloud star S[CLC]k[/CLC] −67°05. ApJL 538, L39–L42. doi:10.1086/312800
Fuente, A., Rivière-Marichalar, P., Beitia-Antero, L., Caselli, P., Wakelam, V., Esplugues, G., et al. (2023). Gas phase Elemental abundances in Molecular cloudS (GEMS). VII. Sulfur elemental abundance. A&A 670, A114. doi:10.1051/0004-6361/202244843
Furuya, K., Oba, Y., and Shimonishi, T. (2022). Quantifying the chemical desorption of H2S and PH3 from amorphous water-ice surfaces. ApJ 926, 171. doi:10.3847/1538-4357/ac4260
Furuya, K., and Shimonishi, T. (2024). Deep search for phosphine in a prestellar core. arXiv e-prints, 05978doi. arXiv:2406. doi:10.48550/arXiv.2406.05978
García de la Concepción, J., Cavallotti, C., Barone, V., Puzzarini, C., and Jiménez-Serra, I. (2024). Relevance of the P+O2 reaction for PO formation in astrochemical environments: electronic structure calculations and kinetic simulations. ApJ 963, 142. doi:10.3847/1538-4357/ad1ffa
García de la Concepción, J., Puzzarini, C., Barone, V., Jiménez-Serra, I., and Roncero, O. (2021). Formation of phosphorus monoxide (PO) in the interstellar medium: insights from quantum-chemical and kinetic calculations. ApJ 922, 169. doi:10.3847/1538-4357/ac1e94
Glassgold, A. E., Mamon, G. A., Omont, A., and Lucas, R. (1987). Photochemistry and molecular ions in carbon-rich circumstellar envelopes. A&A 180, 183–190.
Gould, R. J., and Salpeter, E. E. (1963). The interstellar abundance of the hydrogen molecule. I. Basic processes. ApJ 138, 393. doi:10.1086/147654
Greaves, J. S., Richards, A. M. S., Bains, W., Rimmer, P. B., Sagawa, H., Clements, D. L., et al. (2021). Phosphine gas in the cloud decks of Venus. Nat. Astron. 5, 655–664. doi:10.1038/s41550-020-1174-4
Guelin, M., Cernicharo, J., Paubert, G., and Turner, B. E. (1990). Free CP in IRC +10216. A&A 230, L9–L11.
Haasler, D., Rivilla, V. M., Martín, S., Holdship, J., Viti, S., Harada, N., et al. (2022). First extragalactic detection of a phosphorus-bearing molecule with ALCHEMI: phosphorus nitride (PN). A&A 659, A158. doi:10.1051/0004-6361/202142032
Halfen, D. T., Clouthier, D. J., and Ziurys, L. M. (2008). Detection of the CCP radical (X2Πr) in IRC +10216: a new interstellar phosphorus-containing species. ApJL 677, L101–L104. doi:10.1086/588024
Helminger, P., and Gordy, W. (1969). Submillimeter-wave spectra of ammonia and phosphine. Phys. Rev. 188, 100–108. doi:10.1103/PhysRev.188.100
Hoeft, J., Tiemann, E., and Törring, T. (1972). Rotationsspektrum des PN. Z. Naturforsch. Teil A 27, 703–704. doi:10.1515/zna-1972-0424
Jenkins, E. B. (2009). A unified representation of gas-phase element depletions in the interstellar medium. ApJ 700, 1299–1348. doi:10.1088/0004-637X/700/2/1299
Jenkins, E. B., Savage, B. D., and Spitzer, J. L. (1986). Abundances of interstellar atoms from ultaviolet absorption lines. ApJ 301, 355. doi:10.1086/163906
Jiménez-Serra, I., Viti, S., Quénard, D., and Holdship, J. (2018). The chemistry of phosphorus-bearing molecules under energetic phenomena. ApJ 862, 128. doi:10.3847/1538-4357/aacdf2
Johns, J. W. C., Stone, J. M. R., and Winnewisser, G. (1971). Millimeter wave spectra of HCP and DCP. J. Mol. Spectrosc. 38, 437–440. doi:10.1016/0022-2852(71)90127-5
Jørgensen, J. K., Belloche, A., and Garrod, R. T. (2020). Astrochemistry during the formation of stars. ARA&A 58, 727–778. doi:10.1146/annurev-astro-032620-021927
Jura, M., and York, D. G. (1978). Observations of interstellar chlorine and phosphorus. ApJ 219, 861–869. doi:10.1086/155847
Kawaguchi, K., Saito, S., and Hirota, E. (1983). Far-infrared laser magnetic resonance detection and microwave spectroscopy of the PO radical. JChPh 79, 629–634. doi:10.1063/1.445810
Kaye, J. A., and Strobel, D. F. (1983). Phosphine photochemistry in Saturn’s atmosphere. GeoRL 10, 957–960. doi:10.1029/GL010i010p00957
Koelemay, L. A., Burton, M. A., Singh, A. P., Sheridan, P. M., Bernal, J. J., and Ziurys, L. M. (2022). Laboratory and astronomical detection of the SiP radical (X2Πr): more circumstellar phosphorus. ApJL 940, L11. doi:10.3847/2041-8213/ac9d9b
Koelemay, L. A., Gold, K. R., and Ziurys, L. M. (2023). Phosphorus-bearing molecules PO and PN at the edge of the Galaxy. Nature 623, 292–295. doi:10.1038/s41586-023-06616-1
Koo, B.-C., Lee, Y.-H., Moon, D.-S., Yoon, S.-C., and Raymond, J. C. (2013). Phosphorus in the young supernova remnant cassiopeia A. Science 342, 1346–1348. doi:10.1126/science.1243823
Larson, H. P., Treffers, R. R., and Fink, U. (1977). Phosphine in Jupiter’s atmosphere: the evidence from high-altitude observations at 5 micrometers. ApJ 211, 972–979. doi:10.1086/155009
Lebouteiller, V., Heap, S., Hubeny, I., and Kunth, D. (2013). Chemical enrichment and physical conditions in I Zw 18. A&A 553, A16. doi:10.1051/0004-6361/201220948
Lebouteiller, V., Kuassivi, , and Ferlet, R. (2006a). “Phosphorus in the diffuse ISM,” In Astrophysics in the far ultraviolet: five years of discovery with FUSE. Editors G. Sonneborn, H. W. Moos, and B. G. Andersson (Astronomical Society of the Pacific Conference Series), 348, 480.
Lebouteiller, V., Kunth, D., Lequeux, J., Aloisi, A., Désert, J. M., Hébrard, G., et al. (2006b). Interstellar abundances in the neutral and ionized gas of NGC 604. A&A 459, 161–174. doi:10.1051/0004-6361:20053161
Lefloch, B., Vastel, C., Viti, S., Jimenez-Serra, I., Codella, C., Podio, L., et al. (2016). Phosphorus-bearing molecules in solar-type star-forming regions: first PO detection. MNRAS 462, 3937–3944. doi:10.1093/mnras/stw1918
Lodders, K. (2003). Solar system abundances and condensation temperatures of the elements. ApJ 591, 1220–1247. doi:10.1086/375492
Maas, Z. G., Cescutti, G., and Pilachowski, C. A. (2019). Phosphorus abundances in the hyades and galactic disk. AJ 158, 219. doi:10.3847/1538-3881/ab4a1a
Macia’, E. (2005). The role of phosphorus in chemical evolution. Chem. Soc. Rev. 34, 691–701. doi:10.1039/b416855k
MacKay, D. D. S., and Charnley, S. B. (2001). Phosphorus in circumstellar envelopes. MNRAS 325, 545–549. doi:10.1046/j.1365-8711.2001.04429.x
Maki, A. G., and Lovas, F. J. (1981). The infrared spectrum of 31P 14N near 1300 cm -1. J. Mol. Spectrosc. 85, 368–374. doi:10.1016/0022-2852(81)90209-5
Marks, M., Kroupa, P., Dabringhausen, J., and Pawlowski, M. S. (2012). Evidence for top-heavy stellar initial mass functions with increasing density and decreasing metallicity. MNRAS 422, 2246–2254. doi:10.1111/j.1365-2966.2012.20767.x
Martín, S., Mangum, J. G., Harada, N., Costagliola, F., Sakamoto, K., Muller, S., et al. (2021). ALCHEMI, an ALMA comprehensive high-resolution extragalactic molecular inventory. Survey presentation and first results from the ACA array. A&A 656, A46. doi:10.1051/0004-6361/202141567
Matthews, H. E., Feldman, P. A., and Bernath, P. F. (1987). Upper limits to interstellar PO. ApJ 312, 358. doi:10.1086/164881
McElroy, D., Walsh, C., Markwick, A. J., Cordiner, M. A., Smith, K., and Millar, T. J. (2013). The UMIST database for astrochemistry 2012. A&A 550, A36. doi:10.1051/0004-6361/201220465
McGuire, B. A. (2022). 2021 census of interstellar, circumstellar, extragalactic, protoplanetary disk, and exoplanetary molecules. ApJS 259, 30. doi:10.3847/1538-4365/ac2a48
Milam, S. N., Halfen, D. T., Tenenbaum, E. D., Apponi, A. J., Woolf, N. J., and Ziurys, L. M. (2008). Constraining phosphorus chemistry in carbon- and oxygen-rich circumstellar envelopes: observations of PN, HCP, and CP. ApJ 684, 618–625. doi:10.1086/589135
Millar, T. J., Bennett, A., and Herbst, E. (1987). An efficient gas phase synthesis for interstellar PN. MNRAS 229, 41P–44P. doi:10.1093/mnras/229.1.41P
Mininni, C., Fontani, F., Rivilla, V. M., Beltrán, M. T., Caselli, P., and Vasyunin, A. (2018). On the origin of phosphorus nitride in star-forming regions. MNRAS 476, L39–L44. doi:10.1093/mnrasl/sly026
Molpeceres, G., and Kästner, J. (2021). Computational study of the hydrogenation sequence of the phosphorous atom on interstellar dust grains. ApJ 910, 55. doi:10.3847/1538-4357/abe38c
Molpeceres, G., Zaverkin, V., Furuya, K., Aikawa, Y., and Kästner, J. (2023). Reaction dynamics on amorphous solid water surfaces using interatomic machine-learned potentials. Microscopic energy partition revealed from the P + H → PH reaction. A&A. 673 (A51). doi:10.1051/0004-6361/202346073
Müller, H. S. P. (2013). Spectroscopic parameters of phosphine, PH3, in its ground vibrational state. JQSRT 130, 335–340. doi:10.1016/j.jqsrt.2013.05.002
Nandakumar, G., Ryde, N., Montelius, M., Thorsbro, B., Jönsson, H., and Mace, G. (2022). The Galactic chemical evolution of phosphorus observed with IGRINS. A&A 668, A88. doi:10.1051/0004-6361/202244724
Padovani, M., Galli, D., and Glassgold, A. E. (2009). Cosmic-ray ionization of molecular clouds. A&A 501, 619–631. doi:10.1051/0004-6361/200911794
Pantaleone, S., Corno, M., Rimola, A., Balucani, N., and Ugliengo, P. (2021). Ab initio computational study on Fe2NiP schreibersite: bulk and surface characterization. ACS Earth Space Chem. 5, 1741–1751. doi:10.1021/acsearthspacechem.1c00083
Pantaleone, S., Corno, M., Rimola, A., Balucani, N., and Ugliengo, P. (2023). Computational study on the water corrosion process at schreibersite (Fe2NiP) surfaces: from phosphide to phosphates. ACS Earth Space Chem. 7, 2050–2061. doi:10.1021/acsearthspacechem.3c00167
Pasek, M. A., and Lauretta, D. S. (2005). Aqueous corrosion of phosphide minerals from iron meteorites: a highly reactive source of prebiotic phosphorus on the surface of the early Earth. Astrobiology 5, 515–535. doi:10.1089/ast.2005.5.515
Pickett, H. M., Poynter, R. L., Cohen, E. A., Delitsky, M. L., Pearson, J. C., and Müller, H. S. P. (1998). Submillimeter, millimeter and microwave spectral line catalog. JQSRT 60, 883–890. doi:10.1016/S0022-4073(98)00091-0
Pirronello, V., Liu, C., Roser, J. E., and Vidali, G. (1999). Measurements of molecular hydrogen formation on carbonaceous grains. A&A 344, 681–686.
Plane, J., Feng, W., and Douglas, K. (2021). “Phosphorus chemistry in planetary upper atmospheres,” in European planetary science congress. EPSC2021–322. doi:10.5194/epsc2021-322
Qin, Z., Hu, P., Li, J., and Liu, L. (2023). Radiative association of P+ (3P) and O(3P) for the PO+ formation. MNRAS 523, 2684–2692. doi:10.1093/mnras/stad1571
Ranasinghe, S., and Leahy, D. (2022). Distances, radial distribution, and total number of galactic supernova remnants. ApJ 940, 63. doi:10.3847/1538-4357/ac940a
Ritchey, A. M., Brown, J. M., Federman, S. R., and Sonnentrucker, P. (2023). A reexamination of phosphorus and chlorine depletions in the diffuse interstellar medium. ApJ 948, 139. doi:10.3847/1538-4357/acc179
Ritchey, A. M., Federman, S. R., and Lambert, D. L. (2018). Abundances and depletions of neutron-capture elements in the interstellar medium. ApJS 236, 36. doi:10.3847/1538-4365/aab71e
Rivilla, V. M., Drozdovskaya, M. N., Altwegg, K., Caselli, P., Beltrán, M. T., Fontani, F., et al. (2020). ALMA and ROSINA detections of phosphorus-bearing molecules: the interstellar thread between star-forming regions and comets. MNRAS 492, 1180–1198. doi:10.1093/mnras/stz3336
Rivilla, V. M., Fontani, F., Beltrán, M. T., Vasyunin, A., Caselli, P., Martín-Pintado, J., et al. (2016). The first detections of the key prebiotic molecule PO in star-forming regions. ApJ 826, 161. doi:10.3847/0004-637X/826/2/161
Rivilla, V. M., García De La Concepción, J., Jiménez-Serra, I., Martín-Pintado, J., Colzi, L., Tercero, B., et al. (2022). Ionize hard: interstellar PO+ detection. Front. Astronomy Space Sci. 9, 829288. doi:10.3389/fspas.2022.829288
Rivilla, V. M., Jiménez-Serra, I., Zeng, S., Martín, S., Martín-Pintado, J., Armijos-Abendaño, J., et al. (2018). Phosphorus-bearing molecules in the galactic center. MNRAS 475, L30–L34. doi:10.1093/mnrasl/slx208
Roederer, I. U., Jacobson, H. R., Thanathibodee, T., Frebel, A., and Toller, E. (2014). Detection of neutral phosphorus in the near-ultraviolet spectra of late-type stars. ApJ 797, 69. doi:10.1088/0004-637X/797/1/69
Saito, S., Yamamoto, S., Kawaguchi, K., Ohishi, M., Suzuki, H., Ishikawa, S.-I., et al. (1989). The microwave spectrum of the CP radical and related astronomical search. ApJ 341, 1114. doi:10.1086/167570
Savage, B. D., and Sembach, K. R. (1996). Interstellar gas-phase abundances and physical conditions toward two distant high-latitude halo stars. ApJ 470, 893. doi:10.1086/177919
Schwartz, A. W. (2006). Phosphorus in prebiotic chemistry. Philosophical Trans. R. Soc. B Biol. Sci. 361, 1743–1749. doi:10.1098/rstb.2006.1901
Shimonishi, T., Izumi, N., Furuya, K., and Yasui, C. (2021). The detection of a hot molecular core in the extreme outer galaxy. ApJ 922, 206. doi:10.3847/1538-4357/ac289b
Sil, M., Srivastav, S., Bhat, B., Mondal, S. K., Gorai, P., Ghosh, R., et al. (2021). Chemical complexity of phosphorous-bearing species in various regions of the interstellar medium. AJ 162, 119. doi:10.3847/1538-3881/ac09f9
Sousa-Silva, C., Seager, S., Ranjan, S., Petkowski, J. J., Zhan, Z., Hu, R., et al. (2020). Phosphine as a biosignature gas in exoplanet atmospheres. Astrobiology 20, 235–268. doi:10.1089/ast.2018.1954
Sousa-Silva, C., Yurchenko, S. N., and Tennyson, J. (2013). A computed room temperature line list for phosphine. J. Mol. Spectrosc. 288, 28–37. doi:10.1016/j.jms.2013.04.002
Souza, A. C., Silva, M. X., and Galvão, B. R. L. (2021). Interconversion mechanisms of PN and PO in the interstellar medium through simple atom-diatom collisions. MNRAS 507, 1899–1903. doi:10.1093/mnras/stab2255
Tafalla, M., and Bachiller, R. (1995). Ammonia emission from bow shocks in the L1157 outflow. ApJL 443, L37. doi:10.1086/187830
Tenenbaum, E. D., Woolf, N. J., and Ziurys, L. M. (2007). Identification of phosphorus monoxide (X2Πr) in VY Canis Majoris: detection of the first PO bond in space. ApJL 666, L29–L32. doi:10.1086/521361
Tenenbaum, E. D., and Ziurys, L. M. (2008). A search for phosphine in circumstellar envelopes: PH3 in IRC +10216 and CRL 2688? ApJL 680, L121–L124. doi:10.1086/589973
Thorne, L. R., Anicich, V. G., Prasad, S. S., and Huntress, J. W. T. (1984). The chemistry of phosphorus in dense interstellar clouds. ApJ 280, 139–143. doi:10.1086/161977
Turner, A. M., Bergantini, A., Abplanalp, M. J., Zhu, C., Góbi, S., Sun, B.-J., et al. (2018). An interstellar synthesis of phosphorus oxoacids. Nat. Commun. 9, 3851. doi:10.1038/s41467-018-06415-7
Turner, B. E., and Bally, J. (1987). Detection of interstellar PN: the first identified phosphorus compound in the interstellar medium. ApJL 321, L75. doi:10.1086/185009
Turner, B. E., Tsuji, T., Bally, J., Guelin, M., and Cernicharo, J. (1990). Phosphorus in the dense interstellar medium. ApJ 365, 569. doi:10.1086/169511
Vasyunin, A. I., and Herbst, E. (2013). A unified Monte Carlo treatment of gas-grain chemistry for large reaction networks. II. A multiphase gas-surface-layered bulk model. ApJ 762, 86. doi:10.1088/0004-637X/762/2/86
Villanueva, G. L., Cordiner, M., Irwin, P. G. J., de Pater, I., Butler, B., Gurwell, M., et al. (2021). No evidence of phosphine in the atmosphere of Venus from independent analyses. Nat. Astron. 5, 631–635. doi:10.1038/s41550-021-01422-z
Viti, S., Jimenez-Serra, I., Yates, J. A., Codella, C., Vasta, M., Caselli, P., et al. (2011). L1157-B1: water and ammonia as diagnostics of shock temperature. ApJL 740, L3. doi:10.1088/2041-8205/740/1/L3
Wakelam, V., Bron, E., Cazaux, S., Dulieu, F., Gry, C., Guillard, P., et al. (2017). H2 formation on interstellar dust grains: the viewpoints of theory, experiments, models and observations. Mol. Astrophys. 9, 1–36. doi:10.1016/j.molap.2017.11.001
Wakelam, V., Herbst, E., Loison, J. C., Smith, I. W. M., Chandrasekaran, V., Pavone, B., et al. (2012). A KInetic database for astrochemistry (KIDA). ApJS 199, 21. doi:10.1088/0067-0049/199/1/21
Willacy, K., and Millar, T. J. (1997). Chemistry in oxygen-rich circumstellar envelopes. A&A 324, 237–248.
Wilson, T. L. (1999). Isotopes in the interstellar medium and circumstellar envelopes. Rep. Prog. Phys. 62, 143–185. doi:10.1088/0034-4885/62/2/002
Woosley, S. E., and Weaver, T. A. (1995). The evolution and explosion of massive stars. II. Explosive hydrodynamics and nucleosynthesis. ApJS 101, 181. doi:10.1086/192237
Wurmser, S., and Bergner, J. B. (2022). New detections of phosphorus molecules toward solar-type protostars. ApJ 934, 153. doi:10.3847/1538-4357/ac7c0e
Wyse, F. C., Manson, E. L., and Gordy, W. (1972). Millimeter wave rotational spectrum and molecular constants of 31P14N. JChPh 57, 1106–1108. doi:10.1063/1.1678365
Yamaguchi, T., Takano, S., Sakai, N., Sakai, T., Liu, S.-Y., Su, Y.-N., et al. (2011). Detection of phosphorus nitride in the lynds 1157 B1 shocked region. PASJ 63, L37–L41. doi:10.1093/pasj/63.5.L37
Yamamoto, S. (2017). Introduction to astrochemistry: chemical evolution from interstellar clouds to star and planet formation. doi:10.1007/978-4-431-54171-4
Ziurys, L. M. (1987). Detection of interstellar PN: the first phosphorus-bearing species observed in molecular clouds. ApJL 321, L81. doi:10.1086/185010
Ziurys, L. M., Milam, S. N., Apponi, A. J., and Woolf, N. J. (2007). Chemical complexity in the winds of the oxygen-rich supergiant star VY Canis Majoris. Nature 447, 1094–1097. doi:10.1038/nature05905
Keywords: star formation, interstellar medium, astrochemistry, evolved stars, protostars
Citation: Fontani F (2024) Observations of phosphorus-bearing molecules in the interstellar medium. Front. Astron. Space Sci. 11:1451127. doi: 10.3389/fspas.2024.1451127
Received: 18 June 2024; Accepted: 25 July 2024;
Published: 21 August 2024.
Edited by:
Piero Ugliengo, University of Turin, ItalyReviewed by:
Martin Robert Stewart McCoustra, Heriot-Watt University, United KingdomAlbert Rimola, Autonomous University of Barcelona, Spain
Jean-Claude Guillemin, UMR6226 Institut des Sciences Chimiques de Rennes (ISCR), France
Copyright © 2024 Fontani. This is an open-access article distributed under the terms of the Creative Commons Attribution License (CC BY). The use, distribution or reproduction in other forums is permitted, provided the original author(s) and the copyright owner(s) are credited and that the original publication in this journal is cited, in accordance with accepted academic practice. No use, distribution or reproduction is permitted which does not comply with these terms.
*Correspondence: Francesco Fontani, ZnJhbmNlc2NvLmZvbnRhbmlAaW5hZi5pdA==