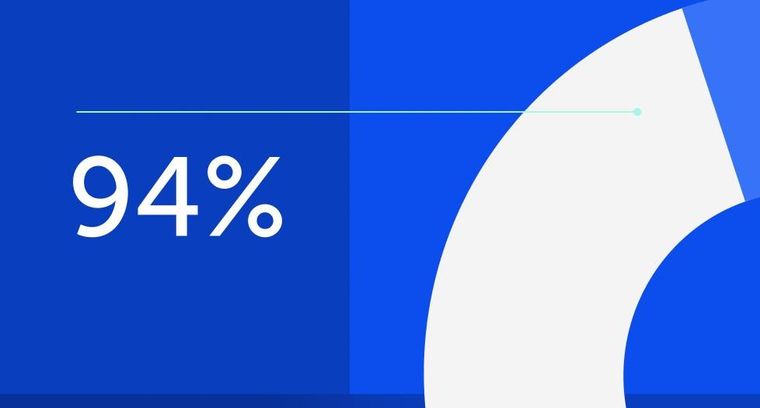
94% of researchers rate our articles as excellent or good
Learn more about the work of our research integrity team to safeguard the quality of each article we publish.
Find out more
OPINION article
Front. Astron. Space Sci., 08 April 2024
Sec. Space Physics
Volume 11 - 2024 | https://doi.org/10.3389/fspas.2024.1391990
This article is part of the Research TopicPast, Present and Future Of Multispacecraft Measurements For Space PhysicsView all 10 articles
It is well known that the electric fields and currents in the polar ionosphere have a significant role in the dissipation of energy through Joule heating. The resulting expansion of the thermosphere can have costly impacts on satellites in low-Earth orbit (Billett et al., 2024). In a paper by Codrescu et al. (1995) it was proposed that the variability in the high-latitude electric field could significantly increase the amount of energy that is dissipated through Joule heating, and therefore electric field models that do not include such variability will underestimate the total amount of heating. Since then, many papers have appeared about the topic of the additional heating that could be produced by the presence of the small-scale (
The electric fields (and heating) are generally the strongest when the Interplanetary Magnetic Field (IMF) is directed in a Southward direction, in parallel with the Earth’s magnetic field in the polar regions. Under such conditions the electric field is generally points from dawn to dusk in the polar region, and towards the equator at auroral latitudes, corresponding to anti-sunward plasma flow in the polar cap and sunward plasma flow at the lower latitudes. An example of such electric fields measured on the DE-2 satellite with the Vector Electric Field Instrument (VEFI) (Maynard et al., 1981) is shown in Figure 1A. The electric field component that is shown is orientated in the direction of the satellite’s motion. Often there may be short-duration jumps in the electric field of a “spiky” nature, as demonstrated in this figure.
Figure 1. Electric field measured on the DE-2 satellite on consecutive polar passes 19 June 1982. The field component EX is parallel to the direction of the satellite’s velocity, with positive values being in the direction of the forward motion. (A) Southern hemisphere (winter) pass. (B) Northern hemisphere (summer) pass.
The existence of these electric field spikes has been known since 1972 when Heppner (1972) had reported that the fluctuations are seen in the polar cap more often in the winter hemisphere than in the summer hemisphere. Maynard et al. (1982) reported that the large-magnitude electric field spikes are commonly seen in the polar cusp region, and also in the nightside auroral oval. This seasonal difference was also found by Heppner et al. (1993), as well as in a recent reanalysis of the DE-2 electric field data (Laakso and Pfaff, 2023). Figure 1 demonstrates the seasonal difference, as the example shown in Figure 1A was a winter pass and Figure 1B shows the electric fields measured in the summer, Northern hemisphere on the same orbit. The summer hemisphere pass exhibits fewer of the large-magnitude fluctuations in comparison to the winter pass. Regarding the duration and magnitudes of the electric field spikes, Laakso and Pfaff (2023) had noted that “the observed events last between 0.1 and 60 s, corresponding to (north-south) widths of 1–500 km along the satellite trajectory.” Magnitudes of electric field spikes in the range of 200–400 mV/m were found to be common in the data from the electric field instrument on the DE-2 satellite. These field strengths correspond to plasma drift velocities in the range of 5–8 km/s. Occasionally peaks of about 1 V/m were seen, correlating to a drift speed of 20 km/s. As noted by Pfaff et al. (2022), these velocities exceed the maximum range of the typical ion drift meter type of instrument that are common on ionospheric spacecraft, that usually have a limit of around 4 km/s. Ion drift meters often have sample rates that are lower than with double-probes such as VEFI, which sampled the electric field at 16 Hz.
As already mentioned, the electric field variability is more pronounced on the night side and winter hemisphere. This behavior leads to the obvious conclusion that the electric field fluctuations have lager magnitudes when the ionospheric conductivity is low, and therefore results in less heating. Evans et al. (1983) had found that “the ionospheric level electric field intensity is highly correlated, often on a one-to-one basis, with the reciprocal of the height-integrated Pedersen conductivity, which in turn is controlled by the auroral electron precipitation.” This anti-correlation between the electric field and conductivity was investigated in more detail by Mallinckrodt and Carlson (1985), Baker et al. (2004), and Zhu et al. (2018).
Cosgrove et al. (2009) looked into this situation and more detail, finding that “because small spatial-scale electric fields are likely polarization electric fields, and therefore negatively correlated with conductance (over space), they may not lead to underestimation of Joule heating.” Furthermore, “the result emphasizes that it cannot be known whether small-spatial-scale variability leads to underestimation or overestimation of the Joule heating rate, until a careful measurement of the spatial correlation between conductance and electric field has been made” (Cosgrove et al., 2009).
Similar to the paper by Codrescu et al. (1995), efforts to model the effects of variability in the electric field often introduce such variations superimposed on a simulated, large-scale electric field model. The problem is that the conductivity in their calculations is often fixed, with the result that larger amounts of heating are found. Obviously, if the anti-correlated variations in the conductivity are not taken into consideration, then the results of such efforts are likely over-exaggerated, in agreement with (Cosgrove et al., 2009).
A similar problem is that the lifetimes of each electric field spike have not considered. Do these large-magnitude field spikes persist for a long time, or just a few seconds or less? Another way of putting it is in terms of their duty-cycle, or what percentage of time they exist at one location compared to being absent? How far do they extend in the transverse direction? The relevant papers generally do not consider that question, although the initial one by Codrescu et al. (1995) did admit that “E-field fluctuations are known to exist on a variety of temporal and spatial scales.” Ignoring these properties can also lend to over-exaggeration of the heating effects, especially if the modeling calculations assume that the fluctuations are fixed in place and are long lasting.
Direct measurements of the Poynting flux from simultaneous vector electric and magnetic field measurements helps to diminish the problem of not knowing conductance values. These Poynting flux data are also subject to misinterpretation if momentary energy spikes that are detected are assumed to persist for the entire duration of the 20–30 min polar pass.
Weimer (2005a) presented an empirical model for calculating the Poynting flux entering the polar ionosphere, derived from combining models of the electric fields and field-aligned currents (Note that a later Weimer (2005b) paper updated the model calculations to use a spherical cap harmonic analysis, but the methods used to obtain the Poynting flux remained the same.). Through use of results from Burke (2008) and the JB2008 thermosphere model (Bowman et al., 2008), Weimer et al. (2011) had found that the total energy flowing into the ionosphere and thermosphere calculated with the 2005 model could account for the observed changes in the density of the global thermosphere in geomagnetic storm intervals. Despite the lack of small-scale variations, there does not seem to be any missing energy in the 2005 model predictions.
The lifetimes and dimensions of the small-scale electric field fluctuations cannot be determined with measurements from only one satellite, so multispacecraft measurements are required. NASA’s future Geospace Dynamics Constellation (GDC) will be useful for this task, as measurements of electric fields and Poynting flux will be obtained at varied intervals of time and location. The Science and Technology Definition Team (STDT) Final Report for the GDC mission (https://lws.larc.nasa.gov/pdf_files/04%20GDC%20STDT%20Report%20FINAL.pdf) refers to a particularly useful orbital configuration known as “pearls on a string,” with multiple satellites on the same orbital plane separated by short distances. In this orbital configuration, if an electric field spike is detected by one or two satellites but not on the following one, then its lifetime could be narrowed down. The expected motion of the electric field spikes, along with their associated aurora, could lead to some ambiguity in the lifetime measurements. But some spikes could disappear, to be replaced by others that have moved into the satellites’ orbit. A careful analysis will be required to resolve some ambiguity. Ideally the initial separation in the GDC mission would be smaller than the 5-min that is illustrated in Figure 3.2 in the report by the STDT (2019). Multispacecraft measurements that are obtained on orbits separated in local time will be worthwhile for estimating the longitudinal spatial dimensions and motions of the small-scale variations.
Unfortunately, the currently planned configuration of the GDC satellites will not have onboard any double-probe type of electric field instrument, like the VEFI on DE-2. With plans for only a plasma drift instrument to measure the electric fields, the GDC capabilities will not be optimal for the detection of electric field spikes having the largest magnitudes (plasma drifts over 5 km/s) and smallest spatial sizes (Pfaff et al., 2022; Laakso and Pfaff, 2023).
To resolve the matter of the amount of heating generated by the small-scale electric field variations, the global, physics based ionosphere-theremosphere-magnetosphere models need further improvement. In order for to fully reconstruct the small-scale structure in the electric field, they would need to have spatial grid resolutions on the order of 10–50 km in both dimensions. The temporal variability of the electric fields in such models will need to be realistic, following from the results obtained from the future GDC mission. Modeling the conductivity variations on similar scales will be difficult, as the conductivity cannot be easily obtained. Conductivity variations that are derived from electron precipitation require that the precipitation inputs have the same spatial resolutions as the electric field, with appropriate dimensions and temporal scales.
During the last 2 decades there has been a common viewpoint that postulates that the small-scale and mesoscale fluctuations in the polar electric fields provide a significant contribution to the amount of Joule heating that is dissipated in the ionosphere. The opinion expressed here is that this significance may be overestimated. Oftentimes the calculations that are presented to support this hypothesis ignore the relevant conductivity variations as well as assuming that the fluctuations persist for tens of minutes. Multispacecraft measurements, such as with the future GDC mission, will provide valuable evidence about the temporal and spatial characteristics of the small-scale fields and Poynting flux, with some limitations due to missing double-probes. These data will help to answer the questions about their significance.
DW: Visualization, Writing–original draft, Writing–review and editing.
The author(s) declare that no financial support was received for the research, authorship, and/or publication of this article.
The author declares that the research was conducted in the absence of any commercial or financial relationships that could be construed as a potential conflict of interest.
All claims expressed in this article are solely those of the authors and do not necessarily represent those of their affiliated organizations, or those of the publisher, the editors and the reviewers. Any product that may be evaluated in this article, or claim that may be made by its manufacturer, is not guaranteed or endorsed by the publisher.
Baker, J. B. H., Zhang, Y., Greenwald, R. A., Paxton, L. J., and Morrison, D. (2004). Height-integrated Joule and auroral particle heating in the night side high latitude thermosphere. Geophys. Res. Lett. 31. doi:10.1029/2004GL019535
Billett, D. D., Sartipzadeh, K., Ivarsen, M. F., Iorfida, E., Doornbos, E., Kalafatoglu Eyiguler, E. C., et al. (2024). The 2022 Starlink geomagnetic storms: global thermospheric response to a high-latitude ionospheric driver. Space weather. 22, e2023SW003748. doi:10.1029/2023SW003748
Bowman, B. R., Tobiska, W. K., Marcos, F. A., Huang, C. Y., Lin, C. S., and Burke, W. J. (2008). “A new empirical thermospheric density model JB2008 using new solar and geomagnetic indices,” in AIAA/AAS Astrodynamics Specialist Conference Proceedings, Honolulu, HI, USA, August 2008, 19.
Burke, W. J. (2008). “Stormtime energy budgets of the global thermosphere,” in Mid-latitude ionospheric Dynamics and disturbances. Editor P. M. Kintner (Washington, DC: NASA), 181, 235–246.
Codrescu, M. V., Fuller-Rowell, T. J., and Foster, J. C. (1995). On the importance of E-field variability for Joule heating in the high-latitude thermosphere. Geophys. Res. Lett. 22, 2393–2396. doi:10.1029/95GL01909
Cosgrove, R. B., Lu, G., Bahcivan, H., Matsuo, T., Heinselman, C. J., and McCready, M. A. (2009). Comparison of AMIE-modeled and Sondrestrom-measured Joule heating: a study in model resolution and electric field–conductivity correlation. J. Geophys. Res. 114. doi:10.1029/2008JA013508
Evans, D. S., Maynard, N. C., Troim, J., Jacobsen, T., and Egeland, A. (1983). Auroral vector electric field and particle comparisons 2. Electrodynamics of an arc. J. Geophys. Res. 82, 2235–2249. doi:10.1029/JA082i016p02235
Heppner, J. (1972). Electric field variations during substorms: OGO-6 measurements. Planet. Space Sci. 20, 1475–1498. doi:10.1016/0032-0633(72)90052-9
Heppner, J. P., Liebrecht, M. C., Maynard, N. C., and Pfaff, R. F. (1993). High-latitude distributions of plasma waves and spatial irregularities from de 2 alternating current electric field observations. J. Geophys. Res. Space Phys. 98, 1629–1652. doi:10.1029/92JA01836
Laakso, H., and Pfaff, R. (2023). Fast plasma drifts in the high latitude ionosphere. Geophys. Res. Lett. 50, e2023GL103566. doi:10.1029/2023GL103566
Mallinckrodt, A. J., and Carlson, C. W. (1985). On the anticorrelation of the electric field and peak electron energy within an auroral arc. J. Geophy. Res. 90, 399–408. doi:10.1029/JA090iA01p00399
Maynard, N., Bielecki, E., and Burdick, H. (1981). Instrumentation for vector electric field measurements from DE-b. Space Sci. Instrum. 5, 523–534.
Maynard, N. C., Heppner, J. P., and Egeland, A. (1982). Intense, variable electric fields at ionospheric altitudes in the high latitude regions as observed by DE-2. Geophys. Res. Lett. 9, 981–984. doi:10.1029/GL009i009p00981
Pfaff, R., Laakso, H., Bonnell, J. W., Cattell, C., Lu, G., Thayer, J., et al. (2022). Measuring the vector electric field in the ionosphere – important new scientific and space weather advances and a synopsis of double probes as an excellent instrument to measure electric fields on non-spinning satellites. A White Pap. 2024-2033 Decadal Surv. Sol. Space Phys. (Heliophysics). Available at: https://surveygizmoresponseuploads.s3.amazonaws.com/fileuploads%2F623127%2F6920789%2F79-39d6663d3238e36b6b61e 223c229605e_PfaffRobertF2.pdf.
Sheng, C., Deng, Y., Bristow, W. A., Nishimura, Y., Heelis, R. A., and Gabrielse, C. (2022). Multi-scale geomagnetic forcing derived from high-resolution observations and their impacts on the upper atmosphere. Space weather. 20, e2022SW003273. doi:10.1029/2022SW003273
STDT (2019). NASA science and Technology definition Team for the Geospace Dynamics constellation: final report. Available at: https://lws.larc.nasa.gov/pdf_files/04%20GDC%20STDT%20Report%20FINAL.pdf. (Accessed February 12 2019).
Weimer, D. R. (2005a). Improved ionospheric electrodynamic models and application to calculating Joule heating rates. J. Geophys. Res. 110, A05306. doi:10.1029/2004JA010884
Weimer, D. R. (2005b). Predicting surface geomagnetic variations using ionospheric electrodynamic models. J. Geophys. Res. 110, A12307. doi:10.1029/2005JA011270
Weimer, D. R., Bowman, B. R., Sutton, E. K., and Tobiska, W. K. (2011). Predicting global average thermospheric temperature changes resulting from auroral heating. J. Geophys. Res. 116, A01312. doi:10.1029/2010JA015685
Keywords: electric fields, Joule heating, small-scale, mesoscale, structure, variability, conductivity, GDC
Citation: Weimer D (2024) The significance of small-scale electric fields may be overestimated. Front. Astron. Space Sci. 11:1391990. doi: 10.3389/fspas.2024.1391990
Received: 26 February 2024; Accepted: 25 March 2024;
Published: 08 April 2024.
Edited by:
Katariina Nykyri, National Aeronautics and Space Administration, United StatesReviewed by:
Mario J. Pinheiro, University of Lisbon, PortugalCopyright © 2024 Weimer. This is an open-access article distributed under the terms of the Creative Commons Attribution License (CC BY). The use, distribution or reproduction in other forums is permitted, provided the original author(s) and the copyright owner(s) are credited and that the original publication in this journal is cited, in accordance with accepted academic practice. No use, distribution or reproduction is permitted which does not comply with these terms.
*Correspondence: Daniel Weimer, ZHdlaW1lckB2dC5lZHU=
Disclaimer: All claims expressed in this article are solely those of the authors and do not necessarily represent those of their affiliated organizations, or those of the publisher, the editors and the reviewers. Any product that may be evaluated in this article or claim that may be made by its manufacturer is not guaranteed or endorsed by the publisher.
Research integrity at Frontiers
Learn more about the work of our research integrity team to safeguard the quality of each article we publish.