- 1Department of Physics, Università della Calabria, Rende, Italy
- 2INAF-Institute for Space Astrophysics and Planetology, Roma, Italy
- 3National Institute for Astrophysics, Scientific Directorate, Roma, Italy
Observations of energetic particles at interplanetary shocks are important to study acceleration mechanisms and their connection with magnetohydrodynamic turbulence. Energetic storm particle (ESP) events are increases in proton fluxes that occur locally at the passage time of interplanetary shocks. These events are more dangerous when they are superimposed on the solar energetic particles (SEPs) produced by the eruption of flares and/or CME-driven shocks propagating from the corona to the interplanetary space. We considered ESP events occurring in association with SEPs on 3 November 2021. We used proton fluxes provided by Solar Orbiter (located at 0.85 AU) in the energy range of 30 keV–82 MeV, by Wind at energies from 70 keV to 72 MeV, and ACE in the range from 40 keV to 5 MeV (both located at the Lagrangian point L1, close to 1 AU along the Sun-Earth direction). In order to broaden the range of analyzed energies (40 keV - 72 MeV), we combine these data with the proton fluxes from the SOHO spacecraft, also located at L1. We analyzed the ESP event and fitted the proton energy spectra at both locations with several distributions to shed light on the mechanisms leading to the acceleration of energetic particles. We also investigated the turbulent magnetic field fluctuations around the shock. The obtained ESP spectra, best reproduced by the so-called double power law function, the spectral differences at the two locations, and the shock features (quasi-parallel geometry, enhanced downstream turbulence) suggest that diffusive shock acceleration is responsible for acceleration of low energy particles, whereas stochastic acceleration contributes to the (re) acceleration of high energies ones.
1 Introduction
Coronal mass ejections (CMEs) emitted in the solar corona can generate shock waves propagating in interplanetary (IP) space. Local enhancements of energetic charged particle intensities observed at IP shocks are known as energetic storm particles (ESPs; Gosling et al., 1981; Pesses et al., 1982; Tsurutani and Lin, 1985). There is a wide variety of different types of ESP events: classical, spike, step-like or irregular according to their time profile (Lario et al., 2003). These energetic particles are accelerated from a few tens of keV to many MeV (Kallenrode, 1996), although the acceleration efficiency decreases with energy, so enhancements are observed frequently in ion fluxes at low energy (Tsurutani and Lin, 1985; Lario et al., 2003; Dresing et al., 2016). ESPs can occur at the shock passage after the initially accelerated solar energetic particles (SEPs) have been released in the IP space.
The diffusive shock acceleration (DSA) theory (see, e.g., Krymskii, 1977; Bell, 1978; Blandford and Ostriker, 1978) can describe particle acceleration at shocks occurring in interplanetary space. DSA predicts a power-law energy spectrum, and several observed energy spectra, in the range from a few tens up to a few hundreds of keV, show values consistent with those predicted by this mechanism (Giacalone, 2012; Parker and Zank, 2012). Nevertheless, it has been shown that many ESP events do not follow the relation predicted by the DSA theory (see, e.g., Ho et al., 2003; Desai et al., 2004; Fisk and Gloeckler, 2012). Ellison and Ramaty (1985) proposed an exponential decay to describe the rollover in the power-law spectra at high energies. Mewaldt et al. (2005) have shown that in the case of SEP events the energy spectra can be reproduced by a power law form only at low energies, whereas the so-called double power law proposed by Band et al. (1993) provides better fits to these energy spectra (see also, Tylka et al., 2005). Stochastic acceleration (SA) mechanism could play a role in particle energization at shocks (Schlickeiser et al., 1993). Afanasiev et al. (2014) argued that the spectral break in the double power law of SEP events can be attributed to the stochastic re-acceleration of energetic protons by enhanced Alfvénic turbulence in the downstream region of a coronal shock wave. Chiappetta et al. (2021) invoked the SA mechanism to explain the ESP events, for which spectra at quasi-parallel shocks were reproduced with the Band function. On the other hand, it was found that the Weibull function (Weibull, 1951) provides the best fit for proton energy spectra in several SEP, ESP and Corotating Interaction Region (CIR) events (see, e.g., Laurenza et al., 2013; Laurenza et al., 2015; Laurenza et al., 2016; Chiappetta et al., 2021) in the case of quasi-perpendicular shocks. The Weibull distribution can be theoretically obtained as a steady-state solution of the diffusion-loss equation in a model wherein acceleration is represented as an anomalous diffusion in momentum space (Pallocchia et al., 2017). In the framework of particle acceleration, two processes can be related to the Weibull spectra, shock surfing acceleration (SSA; Sagdeev, 1966; Lee et al., 1996) and SA. In the first model, at quasi-perpendicular shocks, the particle energy augments in time as the power law E(t) ∼ t2, the acceleration efficiency decreasing with the shock angle θBn and consequently, a spectrum with a spectral index equal to γ = 1/2 is expected (as defined in Equation 1 in Section 3). An alternative interpretation is provided by the SA of protons in the vicinity of shock waves due to the interactions with magnetic irregularities or turbulent fluctuations.
In this work we investigate the kinetic energy spectra of proton flux enhancements associated with ESP events on 3 November 2021, in order to get more insight into the underlying particle acceleration processes. The same shock, thanks to multi-point in-situ observations, was detected by Solar Orbiter, Wind, Advanced Composition Explorer (ACE), and Solar and Heliospheric Observatory (SOHO) spacecraft. We analyze these ESP events, occurring in association with SEPs, using different spectral distributions. The paper is organized as follows. In Section 2, we describe the utilized data sets and the solar wind plasma observations. In Section 3 we present the results obtained from the analysis of ESP proton energy spectra by using different functional forms, such as the Ellison-Ramaty, double power law and Weibull function. The results about turbulent magnetic fluctuations around the shock are shown in Section 4. Finally, discussion and conclusions are given in Section 5.
2 Observations and data sets
The data used to study the ESP events on 3 November 2021 were taken from Solar Orbiter, Wind, ACE, and SOHO spacecraft. Solar Orbiter was located at about 0.85 AU, while Wind, ACE and SOHO were at the Lagrangian point L1, very close to 1 AU along the Sun-Earth direction. The locations of different satellites in the HEEQ coordinate system are shown in Figure 1.
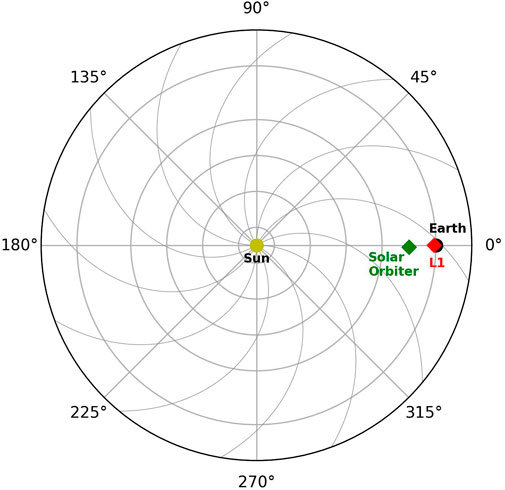
FIGURE 1. Locations of Solar Orbiter and spacecraft at L1 (Wind, ACE and SOHO) in HEEQ coordinate system on 3 November 2021 at 14:00 UT.
From Solar Orbiter, 1 min and 0.125 s magnetic field measurements from the magnetometer (MAG; Horbury et al., 2020), 4 s plasma data from the Solar Wind Analyser (SWA; Owen et al., 2020) and particle fluxes from the Energetic Particle Detector (EPD; Rodríguez-Pacheco et al., 2020) with a resolution of 30 s in the energy range from 30 keV to 82 MeV have been used. For Wind, we used 1 min and 0.092 s magnetic field data from the Magnetic Fields Investigation (MFI; Lepping et al., 1995) magnetometer, 92 s plasma data from Solar Wind Experiment (SWE; Ogilvie et al., 1995), 24 s suprathermal ion observations from the Three-Dimensional Plasma Analyzer (3DP; Lin et al., 1995) in the energy range (70 keV - 6.8 MeV) and 92 s from the Energetic Particles: Acceleration, Composition and Transport (EPACT; von Rosenvinge et al., 1995) in the range (19–72) MeV. ACE data come from the Electron, Proton and Alpha Monitor (EPAM; Gold et al., 1998) instrument in the energy range (47 keV - 4.8 MeV), with 12 s resolution. The High Energy Detector (HED) from the Energetic and Relativistic Nuclei and Electron (ERNE; Torsti et al., 1995) instrument from SOHO has been used for the flux of energetic particles with a resolution of 1 min in the energy channels from 13.0 MeV to 130.0 MeV.
The magnetic field signatures, bulk solar wind velocity, proton density, and temperature recorded by Solar Orbiter and Wind for the ESP events of 3 November 2021 are shown in Figure 2. The dashed lines refer to the shock crossing over the two spacecraft on 2021 November 3 at 14:04 UT, and at 19:35 UT, respectively.
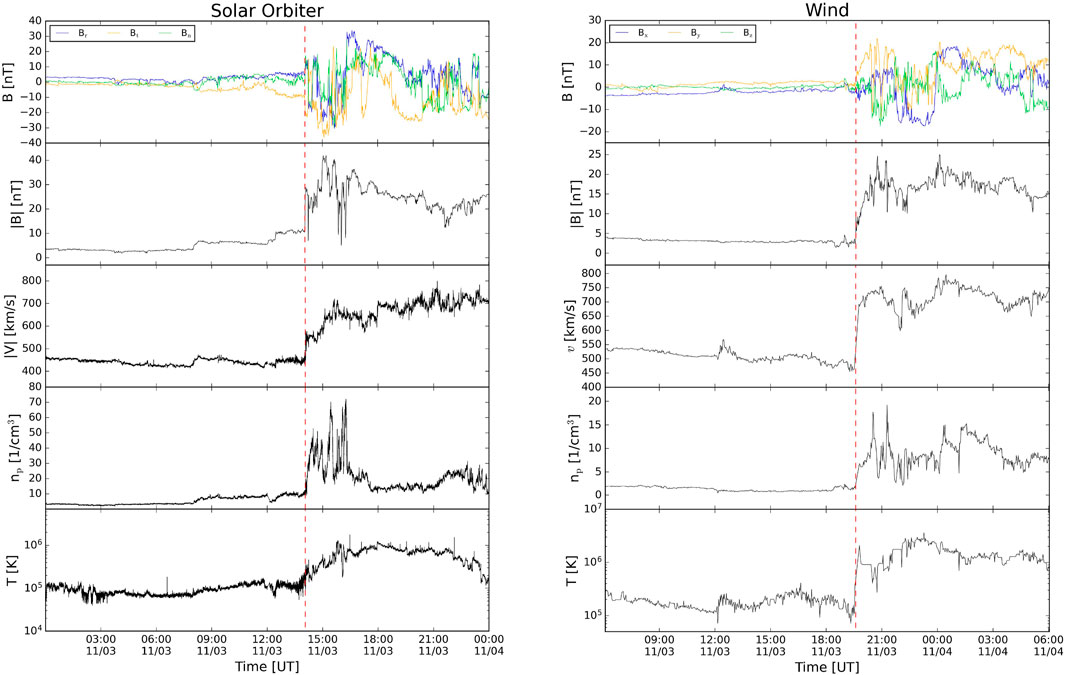
FIGURE 2. Magnetic field and plasma data obtained by Solar Orbiter and Wind spacecraft on the 3rd November 2021. From top to bottom, the panels show the magnetic field components, the magnetic field magnitude, solar wind bulk speed, proton density and temperature. The vertical dashed lines indicate the time of the shock passage over Solar Orbiter at 14:04 UT and Wind on at 19:35 UT.
The key parameters of the fast forward (FF) shock observed by Solar Orbiter and Wind, associated with the ESP events, are taken from Trotta et al. (2023), where the normal vector of the shock
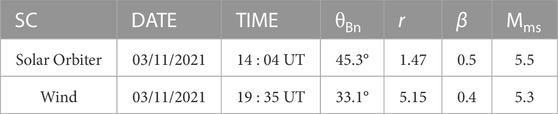
TABLE 1. Parameters of the selected shock observed from Solar Orbiter and Wind. Columns: satellite, date, time of the shock, shock-normal angle (θBn), compression ratio (r), beta of the plasma (β) and magnetosonic Mach number (Mms).
Figure 3 shows proton fluxes around the shock crossing on 2021 November 3 at Solar Orbiter at 14:04 UT, Wind at 19:35 UT, ACE at 19:25 UT and SOHO. Both ESP events are associated with an SEP event. Solar Orbiter data are taken from Electron Proton Telescope (EPT) and High Energy Telescope (HET) sensors. EPT and HET consist of two double-ended telescopes, pointing in different directions: one is pointing sunward and anti-sunward along the nominal Parker spiral and the other one is pointing northward and southward. Figure 3A displays Solar Orbiter fluxes in the sunward direction. 3DP and EPACT data from Wind spacecraft are shown in Figure 3B. In order to broaden the range of energies analysed with Wind, we combined the proton flux data from Wind and ACE (see Figure 3C) with those from SOHO (see Figure 3D), which show fluxes similar to those of the first two spacecraft.
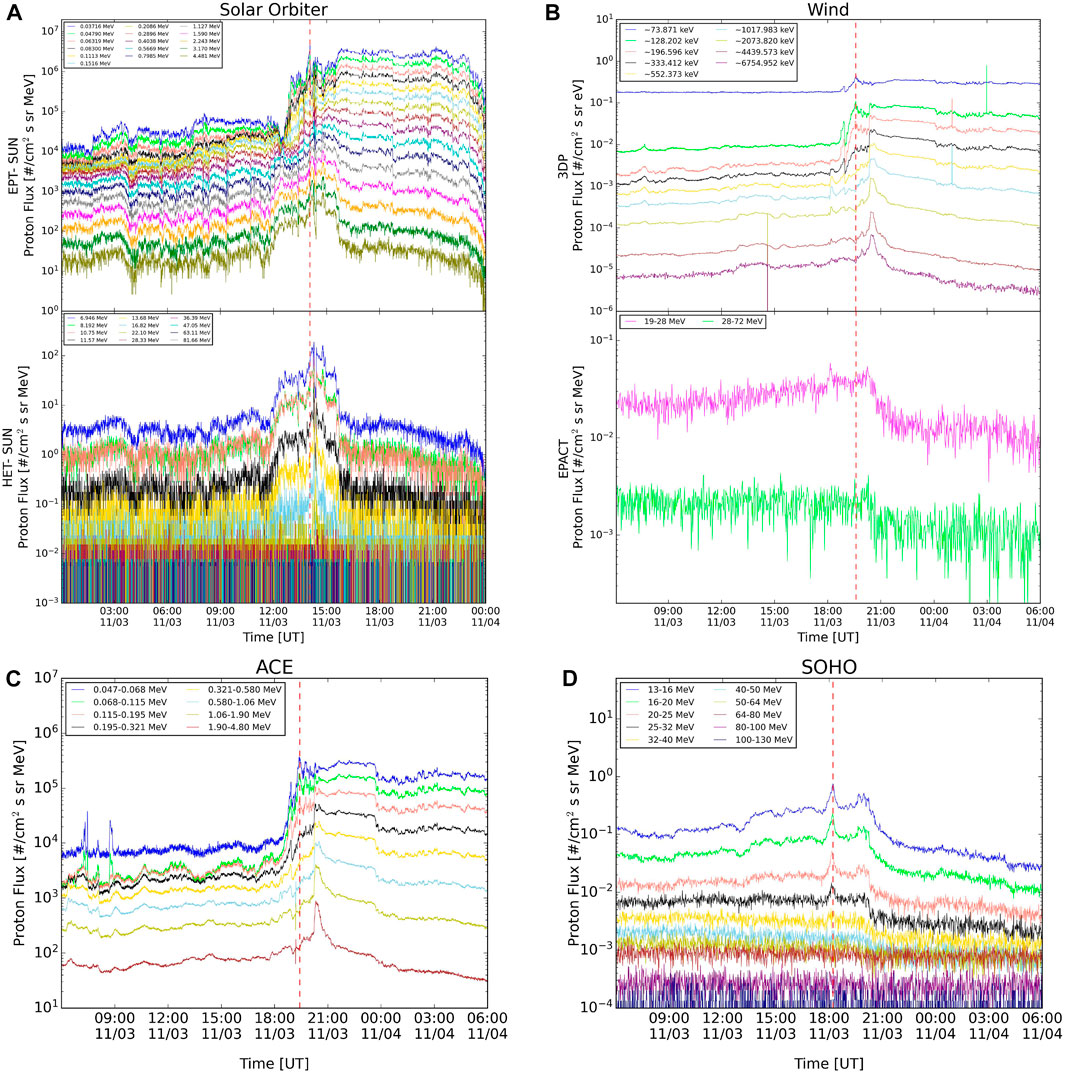
FIGURE 3. Proton fluxes around the shock crossing on 2021 November 3 at (A) Solar Orbiter at 14:04 UT in the sunward direction, (B) Wind at 19:35 UT, (C) ACE at 19:25 UT and (D) SOHO. (A) The upper and lower panels show data recorded by the EPT and HET instruments in the energy range of (0.04–4.5) MeV and (7.0–82) MeV, respectively. (B) Proton intensities taken from 3DP sensor (upper panel) in the energy range (70 keV - 6.8 MeV) and from EPACT instrument in the range (19–72) MeV. (C) Proton fluxes taken from EPAM instrument in the energy range (47 keV - 4.8 MeV). (D) Proton data recorded by the HED detector in the energy range (13–130) MeV. The vertical dashed lines indicate the time of the shock passage over the spacecraft.
3 ESP energy spectra
We analysed the proton energy spectra to have information on the acceleration processes of particles at the shocks when an SEP event is in progress. We calculated the average differential flux (dJ/dE) over an interval of 3 h around the shock arrival. To derive these spectra, we took data in the Sunward direction from EPT and HET sensors on board Solar Orbiter spacecraft.
In addition, the energy spectrum (dJ/dE) over 3 h was also calculated for the shock observed at the Lagrangian point L1 by Wind and ACE on the 3rd November at 19:35 and 19:25 UT, respectively, combining these data with proton intensities from SOHO spacecraft.
Then, we used three different distributions (see, e.g., Chiappetta et al., 2021), Weibull (Frisch and Sornette, 1997), Band (Band et al., 1993) and Ellison-Ramaty (Ellison and Ramaty, 1985) functions to fit differential fluxes dJ/dE. The first functional form has the shape
where C is a scaling factor, Eτ is a characteristic energy and γ is a spectral index (Laurenza et al., 2013; Laurenza et al., 2015; Pallocchia et al., 2017). The Weibull function is also known as the two-parameter stretched exponential associated with particle acceleration by “killed” processes, namely, stochastic processes exhibiting a power-law growth and truncated at random times. In particular, this type of spectrum was found as the steady-state solution of a diffusion-loss equation if we consider a population of particles accelerated by a second-order Fermi mechanism that gives rise to anomalous diffusion in the particle velocity space. The Band double power law function is represented by the equation
where C is a scaling factor, γa is the low energy power law slope, γb is the high energy power law slope and E0 is the break energy. This spectral shape is commonly used to fit particle spectra in SEP events (Band et al., 1993; Tylka et al., 2005; Mewaldt et al., 2012; Desai and Giacalone, 2016). For comparison, we also used the Ellison-Ramaty function (Ellison and Ramaty, 1985),
equal to the Band shape (Eq. 2) below the transition energy, to fit the spectra of the selected ESP events.
Figure 4A shows the spectra of energetic protons related to the 3rd November 2021 ESP event observed from Solar Orbiter and the combined spectra at L1. In the case of the spectrum computed 3 h around the shock arrival at Solar Orbiter, the three distributions have been fitted in the energy range (40.0 keV - 22.10 MeV) and the Band function provides the best fit for the ESP event (blue curve). As can be seen from Figure 4A, the double power law seems to better reproduce also the combined spectrum at L1 in the range of energies from 0.3 to 22.4 MeV (red curve), confirming results from Chiappetta et al. (2021). In fact, in a statistical study on shocks observed from Solar Terrestrial Relations Observatory (STEREO) A spacecraft performed by Chiappetta et al. (2021), they found that the Band distribution better reproduces the proton energy spectra at quasi-parallel shocks associated with an SEP event. On the contrary, for quasi-perpendicular shocks, the best fit is provided by the Weibull function. In our analysis, the shock observed by Solar Orbiter and Wind can be considered in both cases quasi-parallel, since θBn = 45° and θBn = 33°, respectively, and the fit results are in agreement with the previous work. In order to have information directly related to the acceleration region around the shock front, we also calculated the spectra obtained by subtracting the background spectrum calculated over a 1 h interval preceding the ESP increase, at least 1.5 h before the passage of the shock. The subtracted spectrum, displayed in Figure 4B, presents similar results. For the subtracted spectrum calculated at L1 (lime markers) both Band and Ellison-Ramaty function are in good agreement with data. Fit parameters obtained for the ESP events over 3 h around the shock passage and for the background subtracted spectra are listed in Table 2, where γa is the low energy power-law slope, γb is the high energy power-law slope and E0 is the energy break. For both locations we found that the slopes of the Band function at low energies are the same within the uncertainties, and this result is consistent with the small evolution of the shock. The other two parameters obtained from the fit, the rollover energy and the spectral slope above this quantity are also very close to each other at the spacecraft locations.
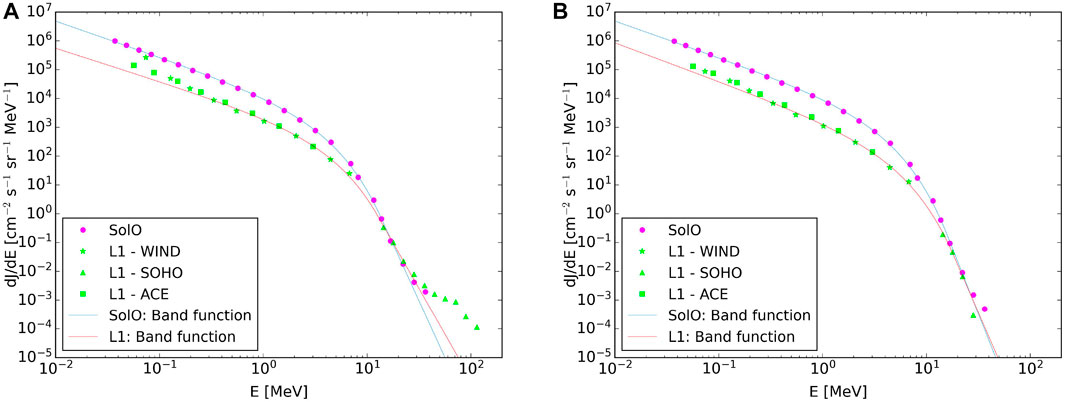
FIGURE 4. (A) Time averaged differential fluxes of energetic particles calculated 3 h around the shock arrival at Solar Orbiter and at L1 and (B) after subtracting the background. Magenta circles indicate the data from EPT and HET telescopes pointing sunward on board Solar Orbiter. The data from Wind, ACE and SOHO are indicated with lime stars, squares and triangles, respectively. The blue and red solid curves are the Band distribution used to fit the spectra at Solar Orbiter and L1, respectively. Data errors are within the marker size.

TABLE 2. Fit parameters, by using Band function, for the ESP events observed from Solar Orbiter in the Sunward direction and from spacecrafts at L1 (Wind, ACE, SOHO).
4 Turbulence around the shock
The characteristics of turbulent fluctuations around interplanetary shocks can have a significant impact on the particle acceleration processes, in particular if SA is involved. Claßen et al. (1999) analysed the correlation between magnetic fluctuations and energetic particles (1 MeV/nucl.) at CIR-related shocks, and found a significant correlation between the high energy proton and helium flux and the turbulence level (quantified by the total wave power in a given frequency range) in the downstream region, while the correlation was found to be not significant for the upstream region. These results were interpreted by the authors as an indication of the existence of strong wave-particle interactions in the region downstream of the shocks. In Chiappetta et al. (2021), the correlation between the proton flux enhancements in the range 4–6 MeV and magnetic field turbulence downstream of interplanetary shocks was analysed for ESP events observed by the STEREO A spacecraft and associated with SEP events. The used turbulence measure was the same as in Claßen et al. (1999). More specifically, a significant correlation was found between the peak flux value in the 4–6 MeV energy range and the magnetic field magnitude fluctuations downstream of the selected shocks, which included both quasi–parallel and quasi–perpendicular shocks. These results appear to confirm the idea of downstream turbulence being a relevant factor in shock acceleration or re-acceleration processes.
In the above mentioned works, the turbulence level was simply quantified through the total wave power integrated over a given frequency range. However, especially when studying a single event, the turbulence properties around an interplanetary shock can be investigated in more detail by calculating the power spectral density (PSD) and structure functions of the magnetic field (see e.g., Carbone et al., 2004; Bruno and Carbone, 2016). For the present analysis, we used magnetic field measurements taken around the shock passages by the Solar Orbiter/MAG instrument (in RTN coordinates) with a 0.125 s resolution and by Wind/MFI (in GSE coordinates) with a 0.092 s resolution. Two time intervals were considered, upstream and downstream of the shock, respectively. The length of the time intervals is 68 min avoiding a 5 min interval around the shock.
The upstream and downstream power spectral densities (PSDs), obtained from the traces of the spectral matrices of magnetic field fluctuations, are shown in Figure 5. Due to the presence of data gaps within both the upstream and downstream Wind/MFI data samples, the PSD in this case was calculated through the Lomb-Scargle method (see e. g.,; Lomb, 1976; Scargle, 1982). The downstream PSD is significantly larger than the upstream one, as expected as a consequence of the enhancement of the fluctuations arising from the shock. The PSDs show a clear inertial range (slightly shorter for the upstream case) with a power law behaviour close to the Kolmogorov-like behaviour f−5/3. It is also possible to observe a breakdown at 0.5–1 Hz, followed by steepening of the spectrum, which can be attributed to the occurrence of kinetic effects (see e.g., Leamon et al., 1998; Bruno and Carbone, 2016).
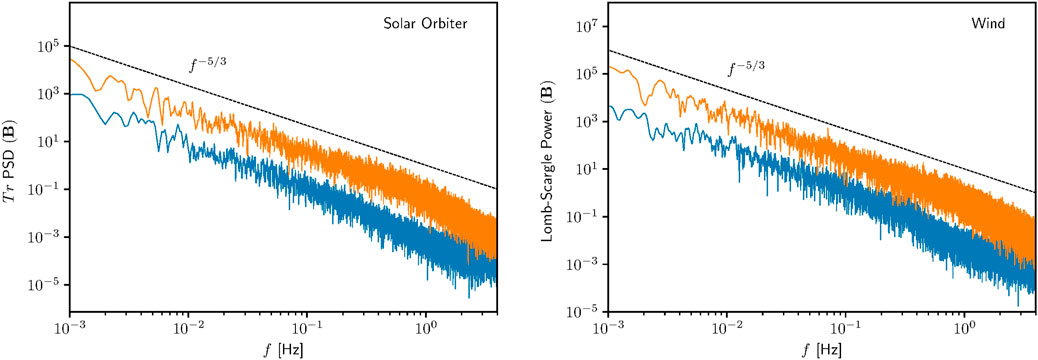
FIGURE 5. PSDs of the magnetic field upstream and downstream of the shock associated with the ESP event of 3 November 2021 from the Solar Orbiter (left) and Wind (right) spacecraft. The PSDs are obtained from the traces of the spectral matrices of magnetic field fluctuations. The black dashed lines corresponds to the power law f−5/3, shown for comparison.
In order to obtain information about the intermittency level of magnetic field fluctuations, the flatness (or kurtosis) Fi(τ) (see e.g., Carbone et al., 2004; Bruno and Carbone, 2016) was calculated as
where
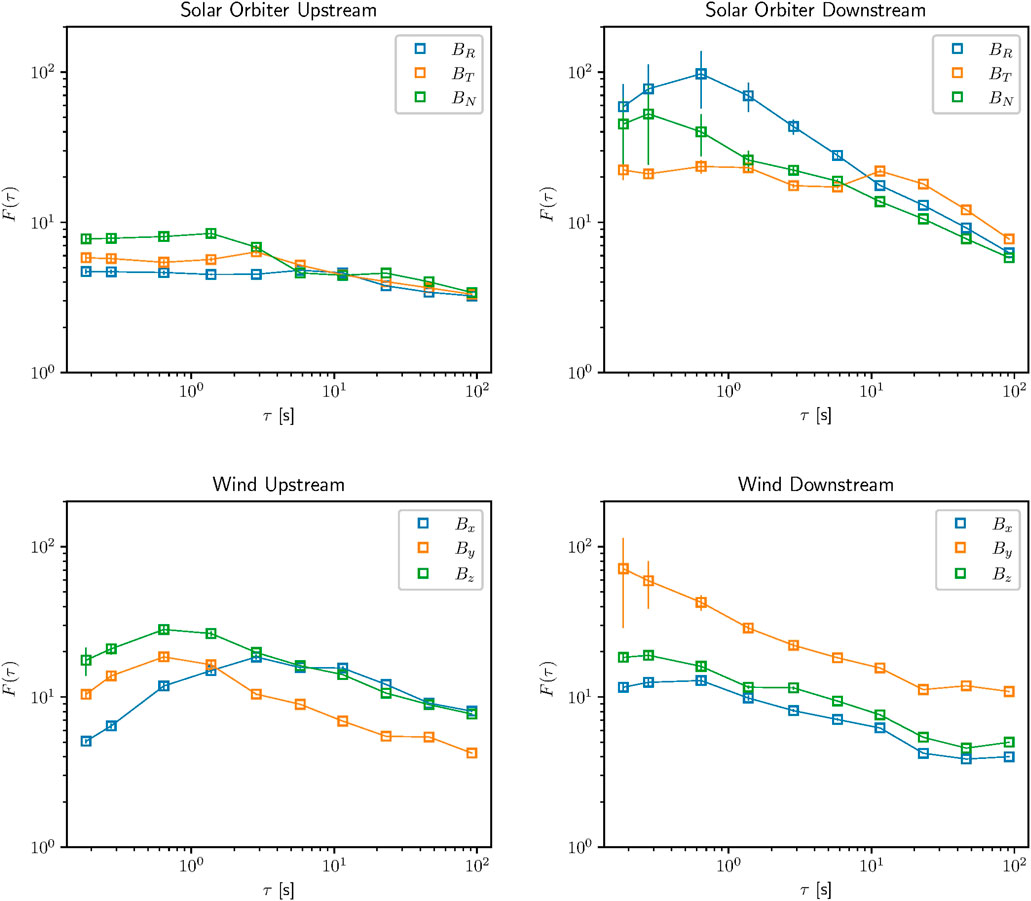
FIGURE 6. Flatness of the increments of the magnetic field components upstream (left panels) and downstream (right panels) of the shock associated with the ESP events of 3 November 2021 as observed by Solar Orbiter at 0.85 AU (top) and Wind at L1 (bottom).
5 Discussion and conclusion
In order to shed light on the mechanisms leading to the acceleration and transport of energetic particles in interplanetary space we have investigated the SEP-associated ESP events observed on the 3rd November 2021 from two locations. We have used in-situ observations of proton fluxes from Solar Orbiter, ACE, and Wind, which observed a shock passage at 14:04 UT, 19:25 UT, and 19:35 UT, respectively. The proton enhancement covered energies from about 40 keV up to about 20 MeV. We separately studied the ESP event for Solar Orbiter and for spacecraft located at L1.
We obtained the ESP spectrum and the background subtracted spectrum for both locations, where the background spectrum was computed in a period of pre-increase, before the ESP start, to the aim of having information directly related to the acceleration region around the shock front. We found that for the shock observed by Solar Orbiter and by the spacecraft at L1, the Band function fits better the differential fluxes of energetic particles. Moreover, a good agreement is observed also between the Band distribution and the background subtracted spectra, which are more representative of the acceleration region around the shock front.
In the scenario of particle acceleration, the Band distribution has been associated with SA mechanism (Afanasiev et al., 2014; Chiappetta et al., 2021), in which particles are stochastically re-accelerated in the downstream region of the shock, where it is found a significant level of magnetic irregularities and turbulent fluctuations (Zank et al., 2006; Giacalone and Jokipii, 2007; Lu et al., 2009; Guo et al., 2012; Pohl et al., 2015). In particular, Chiappetta et al. (2021) showed that for four SEP-associated ESPs at quasi-parallel shocks the Band function reproduces the observed spectra better than other spectral shapes (e.g., the Weibull distribution, which on the contrary, was found to be the best fit for ESPs at quasi-perpendicular shocks). As the shocks observed at both Solar Orbiter and Wind are both quasi-parallel (θBn = 45° and θBn = 33°, respectively), our results confirm previous findings.
By comparing the spectra at Solar Orbiter and L1 we observed that both the spectral indices of the Band function are basically the same within the uncertainties at the two considered locations, which is consistent with the small evolution of the shock. Nevertheless, a flux difference of about 1 order of magnitude at energies below the rollover energy at about 2 MeV can be observed mainly because of the radial density gradient (see e.g., Gardini et al., 2008; Gardini et al., 2011) due to the different location of the spacecraft (0.85 and 0.99, respectively). On the contrary, the spectra at higher energies are quite similar showing no noticeable flux difference between the two locations. Moreover, the particle profile at lower energies (less than 0.5 MeV) both at Solar Orbiter and L1 have the behaviour predicted by the DSA (see e.g., Zank et al., 2006) as the flux remains almost flat in the downstream region after the peak, while at higher energies they show a sharp decrease.
These results support the scenario in which two different mechanisms contribute to the particle acceleration in different energy ranges as proposed in the past (Kallenrode, 1996; Pallocchia et al., 2017; Chiappetta et al., 2021). In particular, we speculate that at low energy the dominant mechanism is the DSA, although the value of the first power law index γa is not related to the shock compression ratio as predicted by the DSA theory. This could be explained by considering that the shock compression ratio is a local measure of the shock at the position of the observer and at the time the shock passes the spacecraft. On the contrary, the particle event and hence the spectral index, although obtained from measurements taken very close to the shock, may combine particles produced at various locations on the shock front, whereas the locally observed plasma properties do not necessarily relate to the properties of the plasma sampled by the mobile energetic particles. As a matter of fact, it is not possible to accurately determine the average plasma density jump across the shock along its surface using only a single spacecraft and this leads to considerable uncertainty in the plasma density jump (Giacalone, 2012). Also past attempts to establish the predicted relationship between the observed power-law spectral exponent and the local plasma density jump across the shock have been unsatisfying (see e.g., van Nes et al., 1984). On the other hand, at higher energy a contribution from the SA can be relevant, as achieved through adiabatic particle reflection from randomly moving turbulent waves or eddies, especially in the downstream region of the shock (Schlickeiser et al., 1993). For instance, Ostrowski (1994) has showed that statistical acceleration by high-amplitude MHD turbulence can transfer the energy of a weak parallel shock to the particles more efficiently than a first-order process. As a matter of fact, our analysis of magnetic field fluctuations around the shock observed at Solar Orbiter and Wind shows a turbulence enhancement in the downstream region, as expected. This is also confirmed by the intermittency level, which is found to be significantly higher in the downstream region with respect to the upstream one.
In conclusion, we found that for both locations the proton spectra are fitted by the Band function at the quasi-parallel shock, confirming that this spectral shape can be related to a stochastic re-acceleration of particles to high energies by enhanced downstream turbulence. Moreover, the higher level of intermittency observed downstream with respect to the upstream region is found to be similar at the two locations as well as the spectral shape (same γb and flux values) at high energies above the rollover energy. This suggests that the more structured downstream turbulence can favor the SA mechanism in determining the particle re-acceleration at high energies and hence the second power law of the ESP spectrum, even regardless of the radial distance.
In this context a systematic study of several ESP events associated with different plasma conditions and shock types, with different geometries going from quasi-parallel to quasi-perpendicular, would help in establishing in clearer way the interplay between particle acceleration processes and turbulent magnetic fluctuations at interplanetary shocks.
Data availability statement
The raw data supporting the conclusion of this article will be made available by the authors, without undue reservation. The data used for this study can be found in the CDAWeb service of NASA at https://cdaweb.gsfc.nasa.gov/index.html for Solar Orbiter, Wind and ACE spacecrafts and in the Space Research Laboratory University of Turku at https://srl.utu.fi/ for SOHO spacecraft.
Author contributions
FC, ML, and FL contributed to conception and design of the study, performed the data analysis and wrote the first draft of the manuscript. SB performed part of the data analysis. All authors contributed to the article and approved the submitted version.
Funding
This research has been carried out in the framework of the CAESAR (Comprehensive spAce wEather Studies for the ASPIS prototype Realization) project, supported by the Italian Space Agency and the National Institute of Astrophysics through the ASI-INAF n. 2020-35-HH.0 agreement for the development of the ASPIS (ASI Space weather InfraStructure) prototype of scientific data centre for Space Weather.
Conflict of interest
The authors declare that the research was conducted in the absence of any commercial or financial relationships that could be construed as a potential conflict of interest.
Publisher’s note
All claims expressed in this article are solely those of the authors and do not necessarily represent those of their affiliated organizations, or those of the publisher, the editors and the reviewers. Any product that may be evaluated in this article, or claim that may be made by its manufacturer, is not guaranteed or endorsed by the publisher.
References
Afanasiev, A., Vainio, R., and Kocharov, L. (2014). The effect of stochastic re-acceleration on the energy spectrum of shock-accelerated protons. Astrophys. J.790, 36. doi:10.1088/0004-637X/790/1/36
Band, D., Matteson, J., Ford, L., Schaefer, B., Palmer, D., Teegarden, B., et al. (1993). BATSE observations of gamma-ray burst spectra. I. Spectral diversity. Astrophys. J.413, 281. doi:10.1086/172995
Bell, A. R. (1978). The acceleration of cosmic rays in shock fronts – I. Mon. Notices R. Astronomical Soc.182, 147–156. doi:10.1093/mnras/182.2.147
Blandford, R. D., and Ostriker, J. P. (1978). Particle acceleration by astrophysical shocks. Astrophys. J. Lett.221, L29–L32. doi:10.1086/182658
Bruno, R., and Carbone, V. (2016). Turbulence in the solar wind. Berlin, Germany: Springer. doi:10.1007/978-3-319-43440-7
Carbone, V., Lepreti, F., Sorriso-Valvo, L., Veltri, P., Antoni, V., and Bruno, R. (2004). Scaling laws in plasma turbulence. Nuovo Cimento Riv. Ser.27, 1–108. doi:10.1393/ncr/i2005-10003-1
Chiappetta, F., Laurenza, M., Lepreti, F., and Consolini, G. (2021). Proton energy spectra of energetic storm particle events and relation with shock parameters and turbulence. Astrophys. J.915, 8. doi:10.3847/1538-4357/abfe09
Claßen, H. T., Mann, G., Forsyth, R., and Keppler, E. (1999). Low frequency plasma turbulence and high energy particles at CIR-related shock waves. Astronomy Astrophysics347, 313.
Desai, M., and Giacalone, J. (2016). Large gradual solar energetic particle events. Living Rev. Sol. Phys.13, 3. doi:10.1007/s41116-016-0002-5
Desai, M. I., Mason, G. M., Wiedenbeck, M. E., Cohen, C. M. S., Mazur, J. E., Dwyer, J. R., et al. (2004). Spectral properties of heavy ions associated with the passage of interplanetary shocks at 1 au. Astrophys. J.611, 1156–1174. doi:10.1086/422211
Dresing, N., Theesen, S., Klassen, A., and Heber, B. (2016). Efficiency of particle acceleration at interplanetary shocks: Statistical study of stereo observations. Astron. Astrophys.588, A17. doi:10.1051/0004-6361/201527853
Ellison, D. C., and Ramaty, R. (1985). Shock acceleration of electrons and ions in solar flares. Astrophys. J.298, 400–408. doi:10.1086/163623
Fisk, L. A., and Gloeckler, G. (2012). Particle acceleration in the heliosphere: Implications for Astrophysics. Space Sci. Rev.173, 433–458. doi:10.1007/s11214-012-9899-8
Frisch, U., and Sornette, D. (1997). Extreme deviations and applications. J. Phys. I7, 1155–1171. doi:10.1051/jp1:1997114
Gardini, A., Laurenza, M., and Storini, M. (2008). Comparing solar energetic particle events from ∼ 0.3 AU to ∼ 1 AU. Mem. della Soc. Astron. Ital. Suppl.12, 91.
Gardini, A., Laurenza, M., and Storini, M. (2011). SEP events and multi-spacecraft observations: Constraints on theory. Adv. Space Res.47, 2127–2139. doi:10.1016/j.asr.2011.01.025
Giacalone, J. (2012). Energetic charged particles associated with strong interplanetary shocks. Astrophys. J.761, 28. doi:10.1088/0004-637X/761/1/28
Giacalone, J., and Jokipii, J. R. (2007). Magnetic field amplification by shocks in turbulent fluids. Astrophys. J.663, L41–L44. doi:10.1086/519994
Gold, R. E., Krimigis, S. M., Hawkins, I., Haggerty, D. K., Lohr, D. A., Fiore, E., et al. (1998). Electron, proton, and Alpha monitor on the advanced composition explorer spacecraft. Space Sci. Rev.86, 541–562. doi:10.1023/A:1005088115759
Gosling, J. T., Asbridge, J. R., Bame, S. J., Feldman, W. C., Zwickl, R. D., Paschmann, G., et al. (1981). Interplanetary ions during an energetic storm particle event: The distribution function from solar wind thermal energies to 1.6 me. J. Geophys. Res.86, 547–554. doi:10.1029/JA086iA02p00547
Guo, F., Li, S., Li, H., Giacalone, J., Jokipii, J. R., and Li, D. (2012). On the amplification of magnetic field by a supernova blast shock wave in a turbulent medium. Astrophys. J.747, 98. doi:10.1088/0004-637X/747/2/98
Ho, G. C., Lario, D., Decker, R. B., Roelof, E. C., Desai, M., and Smith, C. W. (2003). Energetic electrons associated with transient interplanetary shocks: Evidence for weak interaction. Int. Cosm. Ray Conf.6, 3689.
Horbury, T. S., O´Brien, H., Carrasco Blazquez, I., Bendyk, M., Brown, P., Hudson, R., et al. (2020). The solar orbiter magnetometer. Astron. Astrophys.642, A9. doi:10.1051/0004-6361/201937257
Kallenrode, M. B. (1996). A statistical survey of 5-me proton events at transient interplanetary shocks. J. Geophys. Res.101, 24393–24409. doi:10.1029/96JA01897
Krymskii, G. F. (1977). A regular mechanism for the acceleration of charged particles on the front of a shock wave. Akad. Nauk. SSSR Dokl.234, 1306–1308.
Lario, D., Ho, G. C., Decker, R. B., Roelof, E. C., Desai, M. I., and Smith, C. W. (2003). Ace observations of energetic particles associated with transient interplanetary shocks. AIP Conf. Proc.679, 640–643. doi:10.1063/1.1618676
Laurenza, M., Consolini, G., Storini, M., and Damiani, A. (2013). “On the spectral shape of SEP events: An extreme value statistics approach,” in Solar wind 13. Editors G. P. Zank, J. Borovsky, R. Bruno, J. Cirtain, S. Cranmer, H. Elliottet al. (New York: American Institute of Physics Conference Series (AIP)), 219–222. doi:10.1063/1.4811027
Laurenza, M., Consolini, G., Storini, M., and Damiani, A. (2015). The Weibull functional form for sep event spectra. J. Phys. Conf. Ser.632, 012066. doi:10.1088/1742-6596/632/1/012066
Laurenza, M., Consolini, G., Storini, M., Pallocchia, G., and Damiani, A. (2016). The Weibull functional form for the energetic particle spectrum at interplanetary shock waves. J. Phys. Conf. Ser.767, 012015. doi:10.1088/1742-6596/767/1/012015
Leamon, R. J., Smith, C. W., Ness, N. F., Matthaeus, W. H., and Wong, H. K. (1998). Observational constraints on the dynamics of the interplanetary magnetic field dissipation range. J. Geophys. Res. Space Phys.103, 4775–4787. doi:10.1029/97JA03394
Lee, M. A., Shapiro, V. D., and Sagdeev, R. Z. (1996). Pickup ion energization by shock surfing. J. Geophys. Res.101, 4777–4789. doi:10.1029/95JA03570
Lepping, R. P., Acũna, M. H., Burlaga, L. F., Farrell, W. M., Slavin, J. A., Schatten, K. H., et al. (1995). The wind magnetic field investigation. Space Sci. Rev.71, 207–229. doi:10.1007/BF00751330
Lin, R. P., Anderson, K. A., Ashford, S., Carlson, C., Curtis, D., Ergun, R., et al. (1995). A three-dimensional plasma and energetic particle investigation for the wind spacecraft. Space Sci. Rev.71, 125–153. doi:10.1007/BF00751328
Lomb, N. R. (1976). Least-squares frequency analysis of unequally spaced data. Astrophysics Space Sci.39, 447–462. doi:10.1007/BF00648343
Lu, Q., Hu, Q., and Zank, G. P. (2009). The interaction of alfvÉn waves with perpendicular shocks. Astrophys. J.706, 687–692. doi:10.1088/0004-637X/706/1/687
Mewaldt, R. A., Cohen, C. M. S., Labrador, A. W., Leske, R. A., Mason, G. M., Desai, M. I., et al. (2005). Proton, helium, and electron spectra during the large solar particle events of october–november 2003. J. Geophys. Res.110, 38. doi:10.1029/2005JA011038
Mewaldt, R. A., Looper, M. D., Cohen, C. M. S., Haggerty, D. K., Labrador, A. W., Leske, R. A., et al. (2012). Energy spectra, composition, and other properties of ground-level events during solar cycle 23. Space Sci. Rev.171, 97–120. doi:10.1007/s11214-012-9884-2
Ogilvie, K. W., Chornay, D. J., Fritzenreiter, R. J., Hunsaker, F., Keller, J., Lobell, J., et al. (1995). SWE, A comprehensive plasma instrument for the wind spacecraft. Space Sci. Rev.71, 55–77. doi:10.1007/BF00751326
Ostrowski, M. (1994). Efficiency of the second-order Fermi acceleration at parallel shock waves. Astron. Astrophys.283, 344–348.
Owen, C. J., Bruno, R., Livi, S., Louarn, P., Al Janabi, K., Allegrini, F., et al. (2020). The solar orbiter solar wind analyser (swa) suite. Astron. Astrophys.642, A16. doi:10.1051/0004-6361/201937259
Pallocchia, G., Laurenza, M., and Consolini, G. (2017). On Weibull’s spectrum of nonrelativistic energetic particles at ip shocks: Observations and theoretical interpretation. Astrophys. J.837, 158. doi:10.3847/1538-4357/aa633a
Parker, L. N., and Zank, G. P. (2012). Particle acceleration at quasi-parallel shock waves: Theory and observations at 1 au. Astrophys. J.757, 97. doi:10.1088/0004-637X/757/1/97
Paschmann, G., and Schwartz, S. J. (2000). “ISSI book on analysis methods for multi-spacecraft data,” in Cluster-II workshop multiscale/multipoint plasma measurements. Editor R. A. Harris (Netherlands: ESA Publications Division AG Noordwijk), 99.
Pesses, M. E., Decker, R. B., and Armstrong, T. P. (1982). The acceleration of charged particles in interplanetary shock waves. Space Sci. Rev.32, 185–204. doi:10.1007/BF00225184
Pohl, M., Wilhelm, A., and Telezhinsky, I. (2015). Reacceleration of electrons in supernova remnants. Astron. Astrophys.574, A43. doi:10.1051/0004-6361/201425027
Rodríguez-Pacheco, J., Wimmer-Schweingruber, R. F., Mason, G. M., Ho, G. C., Sánchez-Prieto, S., Prieto, M., et al. (2020). The energetic particle detector - energetic particle instrument suite for the solar orbiter mission. Astron. Astrophys.642, A7. doi:10.1051/0004-6361/201935287
Sagdeev, R. Z. (1966). Cooperative phenomena and shock waves in collisionless plasmas. Rev. Plasma Phys.4, 23.
Scargle, J. D. (1982). Studies in astronomical time series analysis. II. Statistical aspects of spectral analysis of unevenly spaced data. Astrophys. J.263, 835–853. doi:10.1086/160554
Schlickeiser, R., Campeanu, A., and Lerche, I. (1993). “Stochastic particle acceleration at shock waves,” in 23rd international cosmic ray conference (ICRC23). Editor D. A. Leahy, R. B. Hicks, and D. Venkatesan (Alberta, Canada: International Cosmic Ray Conference), 211.
Torsti, J., Valtonen, E., Lumme, M., Peltonen, P., Eronen, T., Louhola, M., et al. (1995). Energetic particle experiment ERNE. Sol. Phys.162, 505–531. doi:10.1007/BF00733438
Trotta, D., Hietala, H., Horbury, T., Dresing, N., Vainio, R., Wilson, L., et al. (2023). Multi-spacecraft observations of shocklets at an interplanetary shock. MNRAS520, 437–445. doi:10.1093/mnras/stad104
Tsurutani, B. T., and Lin, R. P. (1985). Acceleration of >47 keV ions and >2 keV electrons by interplanetary shocks at 1 AU. J. Geophys. Res.90, 1–11. doi:10.1029/JA090iA01p00001
Tylka, A. J., Cohen, C. M. S., Dietrich, W. F., Lee, M. A., Maclennan, C. G., Mewaldt, R. A., et al. (2005). Shock geometry, seed populations, and the origin of variable elemental composition at high energies in large gradual solar particle events. Astrophys. J.625, 474–495. doi:10.1086/429384
van Nes, P., Reinhard, R., Sanderson, T. R., Wenzel, K. P., and Zwickl, R. D. (1984). The energy spectrum of 35- to 1600-keV protons associated with interplanetary shocks. J. Geophys. Res.89, 2122–2132. doi:10.1029/JA089iA04p02122
von Rosenvinge, T. T., Barbier, L. M., Karsch, J., Liberman, R., Madden, M. P., Nolan, T., et al. (1995). The energetic particles: Acceleration, composition, and transport (EPACT) investigation on the WIND spacecraft. Space Sci. Rev.71, 155–206. doi:10.1007/BF00751329
Weibull, W. (1951). A statistical distribution function of wide applicability. J. Appl. Mech.18, 293–297. doi:10.1115/1.4010337
Keywords: particle acceleration, shock waves, solar energetic particles, solar-terrestrial relations, turbulence
Citation: Chiappetta F, Laurenza M, Lepreti F, Benella S, Consolini G and Marcucci MF (2023) The energetic storm particle events of 3 November 2021. Front. Astron. Space Sci. 10:1209479. doi: 10.3389/fspas.2023.1209479
Received: 20 April 2023; Accepted: 12 July 2023;
Published: 28 July 2023.
Edited by:
Luca Sorriso-Valvo, National Research Council (CNR), ItalyReviewed by:
Jingnan Guo, University of Science and Technology of China, ChinaVladimir Florinski, University of Alabama in Huntsville, United States
Copyright © 2023 Chiappetta, Laurenza, Lepreti, Benella, Consolini and Marcucci. This is an open-access article distributed under the terms of the Creative Commons Attribution License (CC BY). The use, distribution or reproduction in other forums is permitted, provided the original author(s) and the copyright owner(s) are credited and that the original publication in this journal is cited, in accordance with accepted academic practice. No use, distribution or reproduction is permitted which does not comply with these terms.
*Correspondence: Federica Chiappetta, ZmVkZXJpY2EuY2hpYXBwZXR0YUB1bmljYWwuaXQ=