- 1Department of Climate and Space Sciences and Engineering, The University of Michigan, Ann Arbor, MI, United States
- 2Department of Astronomy, Boston University, Boston, MA, United States
The heliosphere is a protective shield around the solar system created by the Sun’s interaction with the local interstellar medium (LISM) through the solar wind, transients, and interplanetary magnetic field. The shape of the heliosphere is directly linked with interactions with the surrounding LISM, in turn affecting the space environment within the heliosphere. Understanding the shape of the heliosphere, the LISM properties, and their interactions is critical for understanding the impacts within the solar system and for understanding other astrospheres. Understanding the shape of the heliosphere requires an understanding of the heliotail, as the shape is highly dependent upon the heliotail and its LISM interactions. The heliotail additionally presents an opportunity for more direct in situ measurement of interstellar particles from within the heliosphere, given the likelihood of magnetic reconnection and turbulent mixing between the LISM and the heliotail. Measurements in the heliotail should be made of pickup ions, energetic neutral atoms, low energy neutrals, and cosmic rays, as well as interstellar ions that may be injected into the heliosphere through processes such as magnetic reconnection, which can create a direct magnetic link from the LISM into the heliosphere. The Interstellar Probe mission is an ideal opportunity for measurement either along a trajectory passing through the heliotail, via the flank, or by use of a pair of spacecraft that explore the heliosphere both tailward and noseward to yield a more complete picture of the shape of the heliosphere and to help us better understand its interactions with the LISM.
1 Introduction
The solar system comprises planets, asteroids, comets, and dust orbiting the Sun due to the force of gravity. The Sun also fills the interplanetary space with the solar wind, a steady stream of particles leaving the Sun at supersonic speeds, as predicted by Eugene Parker in 1958, as well as solar transients and the interplanetary magnetic field, which has a spiral shape that was also predicted by Parker (1958a) and Parker (1958b). The Sun, therefore, creates a plasma bubble within the local interstellar medium (LISM) comprising the solar wind, solar transients, and interplanetary magnetic field, the composition and magnetic field of which shape the local space environment. This region of the Sun’s direct influence is known as the heliosphere (Ripken and Fahr, 1983; Knie et al., 1999; Opher and Loeb, 2022). Parker’s discoveries led to a better understanding of the heliosphere, and early gas dynamic 2-D models of the heliosphere often depicted the Solar magnetic field as similar to a dipole bar magnet embedded in the surrounding interstellar magnetic field (Davis, 1955; Parker, 1961; Parker, 1963; Axford et al., 1963; Baranov et al., 1971). Another advancement in our understanding of the interactions of the heliosphere and the LISM came in 1971, when it was shown by Thomas and Krassa (1971) and Bertaux and Blamont (1971) using OGO 5 measurements that interstellar neutrals penetrate the heliosphere, as predicted by Fahr (1968). These neutrals are an important seed population for pickup ions (PUIs) and energetic neutral atoms (ENAs). However, it was noted by Axford (1972) that there will be a large gap in our understanding of the heliosphere as long as we continue to have a lack of in situ measurements. Since then, only the Voyager missions have approached the LISM, and even those missions were unable to provide in situ plasma measurements from the LISM. A more detailed history of early heliophysical discoveries can be found in Zank et al. (2022).
The properties of the LISM affect the shape and composition of the heliosphere, which are driven by interstellar interactions, guided by the particle flows and interstellar magnetic field (ISMF) (Müller et al., 2009; Zirnstein et al., 2016b; Linsky et al., 2022). Other important effects of heliosphere–interstellar interactions include evidence suggesting that the origins of life on Earth were influenced by the effects of supernovae within 50 pc of the Earth (Fields et al., 2008; Meyer et al., 2012; Wallner et al., 2016; Fields et al., 2020; Miller and Fields, 2022). In addition, the Sun moves large distances (∼19 pc/Myr) through the quite variable LISM. There is geological evidence from 60Fe and 244Pu isotopes that the Earth received interstellar material approximately 2–3 Myr ago. These isotopes were interpreted as evidence for a nearby supernova, though that has been cast into doubt (Opher et al., 2022). Opher et al. (2022) suggest the encounter of Earth with a massive cold cloud in the local ribbon of cold clouds 3 Myr ago. Such encounters shrunk the Sun’s protective bubble, the heliosphere, to within the Earth’s orbit, exposing Earth to a cold, dense LISM. Such scenarios should be discussed in context with others proposed to explain the cooling seen by oxygen isotopes measured in deep-sea foraminifera. The exposure to a cold, dense LISM has implications on climate and increased radiation. Increased radiation alone could have effects on climate, organismal mutation rates, aging, and extinction rates and thus broad patterns of diversification. Understanding the shape of the heliosphere, including its ability to protect or expose the Earth to the LISM as it grows or shrinks, guides our broader understanding of the habitability and evolution of life on Earth. An understanding of the shape of our heliosphere can additionally guide our understanding of other astrospheres, strengthening ties between heliophysics and the fields of astronomy, astrophysics, and planetary science (Wood et al., 2004; Sahai and Chronopoulos, 2010; Cox et al., 2012; Menten et al., 2012; Peri et al., 2015; Kobulnicky et al., 2016; Katushkina et al., 2018; Baalmann et al., 2020; Baalmann et al., 2022; Czechowski and Grygorczuk, 2022). Less definitive knowledge exists of this shape (Davis, 1955; Parker, 1963; Pauls and Zank, 1996; McComas et al., 2012b; McComas et al., 2013; Heerikhuisen et al., 2014; Opher et al., 2015; Opher et al., 2020; Pogorelov et al., 2015; Dialynas et al., 2017; Izmodenov and Alexashov, 2020; Reisenfeld et al., 2021; Dayeh et al., 2022; Frisch et al., 2022; Kornbleuth et al., 2023a); however, only the Voyager spacecraft may have reached the edge of the heliosphere and directly sampled the interstellar medium beyond (Decker et al., 2005; Decker et al., 2008; Krimigis et al., 2011; Krimigis et al., 2013; Krimigis et al., 2019; Stone et al., 2013; Krimigis et al., 2019; Dialynas et al., 2022).
Our knowledge of the composition, flow, and properties of the LISM is informed almost exclusively from remote sensing or indirect measurements such as of UV backscatter (Weller and Meier, 1974; Ajello, 1978; Dalaudier et al., 1984; Bertaux et al., 1985; Lallement and Bertin, 1992; Lallement et al., 2004; Vallerga et al., 2004; Lallement et al., 2005; Redfield and Linsky, 2008; Slavin and Frisch, 2008; Frisch, 2009; Lallement et al., 2010; Vincent et al., 2012; Frisch et al., 2013; Frisch et al., 2015; Zank et al., 2013; Linsky et al., 2019; Linsky and Redfield, 2021), low-energy neutral atoms (Witte et al., 1992; Witte et al., 1993; Fuselier et al., 2009b; Moebius et al., 2009), ENAs (Fuselier et al., 2009a; Frisch et al., 2009; McComas et al., 2009; McComas et al., 2012b; McComas et al., 2013; Heerikhuisen et al., 2014; Pogorelov et al., 2015; Dialynas et al., 2017; Dialynas et al., 2019; Reisenfeld et al., 2021; Brandt et al., 2022), PUIs (Möbius et al., 1985a; Gloeckler et al., 1992; Gloeckler et al., 1998; Mall et al., 1998; Gloeckler and Geiss, 2004; McComas et al., 2004; Gloeckler et al., 2004; Galvin et al., 2008; McComas et al., 2008; Drews et al., 2012; Gershman et al., 2013; Frisch et al., 2013; Frisch et al., 2015; Möbius et al., 2015a; Möbius et al., 2016a; Möbius et al., 2016b; Taut et al., 2018; Bower et al., 2019; Opher et al., 2020; Swaczyna et al., 2020; Spitzer, 2022; Spitzer et al., 2024), cosmic rays (Blandford and Ostriker, 1978; Ferrière, 2001; Schwadron et al., 2014; Orlando and Strong, 2021), and dust and interstellar polarization (Savage and Mathis, 1979; Frisch et al., 2022). While the UV backscatter measurements are made remotely, other measurements such as of low-energy neutrals, ENAs, and PUIs can be considered “remote in situ” measurements. These measurements are typically made by instruments in near-Earth space, but the data collected can be used to infer properties of regions far away. These measurements are, therefore, taken in situ within the space environment but used for remote sensing techniques. Any additional inferences that we can make about the shape of the heliosphere and its interactions with the LISM are guided by modeling efforts constrained by limited measurements.
The bulk of the interstellar ions, except highly energized galactic cosmic rays (GCRs), are deflected around the heliosphere by the magnetic boundary with the Sun’s interplanetary magnetic field (IMF). The neutral flow, caused by the relative motion of the heliosphere and the LISM, is able to penetrate the heliopause (HP), or the boundary between the heliosphere and the LISM, resulting in the neutral interstellar wind through the heliosphere. These neutrals can then become ionized through processes such as photoionization, electron impact ionization, and charge exchange with the solar wind, creating PUIs, ions that are “picked up” by the solar wind and the IMF, reaching speeds up to two times the solar wind speed (Fahr, 1968; Vasyliunas and Siscoe, 1976; Möbius et al., 1985a; Möbius et al., 1985b). The PUIs that are in turn re-neutralized yield ENAs.
It has been shown (Opher et al., 2020) that consideration of the PUIs embedded in the solar wind can contribute significantly to the shape and dynamics of the heliosphere when accounted for in modeling. McComas et al. (2017b) use in situ data from SWAP with a Vasyliunas and Siscoe model to show that PUIs play a significant role, especially in the outer heliosphere, where they majorly contribute to the solar wind internal pressure by approximately 20 au. The authors show that there is likely additional PUI heating that occurs, as an increase in H+ thermal pressure and temperature can be seen between 22 and 38 au. The PUI pressure is found to be high in the outer heliosphere and is expected to compose a significant portion of the dynamic pressure in the solar wind by the termination shock (TS) at approximately 100 au. PUI measurements are, therefore, a crucial component for studying the TS, the dynamics of the heliosphere, and the outer heliosphere interactions with the LISM.
The Interstellar Probe is a proposed mission concept that has involved the work of over 1,000 scientists over a number of years proposing various potential trajectories to reach the LISM, including via the nose or the heliotail directions (McNutt Jr. et al., 2021; Schlei et al., 2021; Brandt et al., 2022). The general mission goals are to investigate the heliosphere and the Sun’s interaction with the LISM (Schlei et al., 2021). These objectives are to be achieved specifically through the PUI and in situ plasma measurements both inside and outside the heliosphere as well as direct measurements of the VLISM, including properties such as densities, composition, flows, and temperatures of ions, neutral particles, and dust (Brandt et al., 2022). In addition, though the U.S. and China do not directly collaborate on space missions, and while neither mission currently has a set launch date, NASA’s proposed Interstellar Probe and China’s proposed Interstellar Express (Xu et al., 2022) missions could provide complementary measurements to better understand the heliosphere and its interaction with the LISM, just as NASA and ESA’s Parker Solar Probe and Solar Orbiter provide complementary measurements of the Sun. As suggested by Linghua Wang of the ENA imager team on the concept of the Interstellar Express, each mission could have different instrument specifications, and having more of these spacecraft, such as the implementation of both of these proposed missions, would greatly benefit the study of interstellar space (O’Callaghan, 2022). In this paper, we propose both intentional measurements made on future outer heliosphere missions and a heliotailward component of the Interstellar Probe mission to provide the much needed measurements for answering open questions regarding the shape of the heliosphere and its interaction with the LISM. We specifically suggest that such missions should include more comprehensive PUI measurements and the utilization of low-energy neutrals, ENAs, and cosmic ray measurements.
2 Discussion
2.1 Measurements in the heliosphere
In addition to UV backscatter remote sensing techniques (Weller and Meier, 1974; Ajello, 1978; Dalaudier et al., 1984; Bertaux et al., 1985; Lallement and Bertin, 1992; Lallement et al., 2004; Vallerga et al., 2004; Lallement et al., 2005; Lallement et al., 2010; Vincent et al., 2012; Frisch et al., 2013; Frisch et al., 2015), a useful measure for determining the properties of interstellar parameters in situ is through the direct measurement of neutral atoms. Such measurements have been made within the heliosphere from spacecraft such as Ulysses (Witte et al., 1992; Witte et al., 1993) and IBEX (Fuselier et al., 2009b; Moebius et al., 2009). Such neutral measurements have been used to characterize the inflow of neutral particles known as the interstellar wind through the heliosphere. Parameters that have been analyzed include the longitudinal flow direction, velocity, and temperature (Witte et al., 1993; Witte, 2004; McComas et al., 2012a; Möbius et al., 2012; Frisch et al., 2013; Frisch et al., 2015; Schwadron et al., 2013; Bzowski et al., 2014; McComas et al., 2015a; McComas et al., 2015b; Möbius et al., 2015b; Bzowski et al., 2015; Leonard et al., 2015; Schwadron et al., 2015; Wood et al., 2015; Swaczyna et al., 2018; Swaczyna et al., 2022). These measurements, however, have been limited to those that can be made from within the heliosphere and therefore leave our understanding from the outside very limited.
Another important method for studying interstellar parameters and heliosphere–interstellar interactions is through the use of ENAs and PUIs. Most measurements of ENAs and PUIs to date have been made using instruments on spacecraft such as AMPTE-IRM (Möbius et al., 1985b), Ulysses (Gloeckler et al., 1992), ACE (Gloeckler et al., 1998), Cassini (Mall et al., 1998; McComas et al., 2004; Dialynas et al., 2017), New Horizons (McComas et al., 2008), STEREO (Galvin et al., 2008), and IBEX (Fuselier et al., 2009a; Frisch et al., 2009; McComas et al., 2009). He+ PUI measurements, for example, can be used to infer the interstellar neutral flow properties, such as the longitudinal inflow direction of the interstellar wind through the heliosphere (Gloeckler et al., 2004; Drews et al., 2012; Frisch et al., 2013; Frisch et al., 2015; Gershman et al., 2013; Möbius et al., 2015a; Möbius et al., 2016a; Möbius et al., 2016a; Möbius et al., 2016b; Möbius et al., 2016b; Taut et al., 2018; Bower et al., 2019; Spitzer, 2022). These measurements can achieve values consistent with those of other techniques from the literature, such as ENA measurements, and can be made with comparable precision and accuracy (Möbius et al., 2015b; Möbius et al., 2016a; Taut et al., 2018; Bower et al., 2019; Spitzer, 2022). However, due to the low flux/density of interstellar helium, many of these measurements are made at the limits of the instrument capabilities and therefore present challenges which need to be overcome using methods such as limiting measurements to those taken only of newly injected PUIs in order to achieve precise and accurate values (Möbius et al., 2015a). Therefore, improvements upon these measurements could yield significant advances in these techniques. Many of the instruments on the spacecraft listed above do not have characteristics such as high post-acceleration voltages and look directions away from the Sun, ideal for PUI detections, and interstellar measurements were often not included in the mission design or requirements (Gilbert et al., 2016). This limitation could be alleviated through the development of ion mass spectrometers optimized for the detection of PUIs and deployed on outer heliosphere missions with a field of view (FOV) that includes look directions away from the Sun. One such example of a future instrument is SPICES, a technology development project funded by NASA and based on the PICSpec design (Gilbert et al., 2016), which is targeted specifically for the detection of low-charge-state PUIs, including protons, alphas, and heavier ions, using a −50-kV internal post-acceleration region for full energy detection. Intentional PUI detection in the outer heliosphere has been shown to be a critical need, especially of pickup protons and alphas, as the PUI pressure, fueled by particles of interstellar origin, builds up to a significant portion of the dynamic pressure in the outer heliosphere (McComas et al., 2017b).
Thus far, five spacecraft have had solar system escape velocities, namely, Pioneers 10 and 11 (NASA, 1971; Miller, 1973), Voyagers 1 and 2 (Kohlhase and Penzo, 1977; Stone, 1977), and New Horizons (Kusnierkiewicz et al., 2005; Stern, 2008), but as of yet, the only spacecraft which may have directly sampled the LISM, though still within reach of the Sun’s effects, are Voyagers 1 and 2 (Decker et al., 2005; Decker et al., 2008; Stone et al., 2008; Stone et al., 2013; Stone et al., 2019; Krimigis et al., 2011; Krimigis et al., 2013; Krimigis et al., 2019; Dialynas et al., 2022). There remains some debate whether the Voyager spacecraft have left the heliosphere and directly sampled the LISM (Fisk and Gloeckler, 2022). Currently, both Voyagers have exceeded the scope of their missions, and only Voyager 2 is capable of taking plasma measurements since the Plasma Science (PLS) instrument on Voyager 1 failed in 1980 (Richardson and Decker, 2014). Additionally, there are various concerns with the Voyager 2 measurements, including the inability of PLS to fully determine the plasma density in the LISM (Richardson and Decker, 2014), radiation damage caused to the PLS Faraday cups near Jupiter (Gurnett and Kurth, 2019), and degradation of the Voyager 2 Plasma Wave Science (PWS) instrument (Kurth and Gurnett, 2020). Measurements that have been successfully interpreted from the Voyager spacecraft indicate a likely asymmetric TS in space and/or time and an alternate source of anomalous cosmic rays from beyond the TS (Stone et al., 2005; Stone et al., 2008), providing compelling evidence that more measurements are needed between the heliosphere and the LISM. The Voyager measurements imply that our understanding of the heliosphere–interstellar interactions is more limited than previously expected, further supporting the need for future interstellar measurements.
Another source of interstellar measurements is galactic cosmic rays (GCRs). GCRs ranging from MeV to TeV penetrate the heliosphere from the LISM due to their high energies. These GCRs serve both as a sample of particles that originate from outside the heliosphere and as a means of probing the shape of the heliosphere. Since charged particles such as cosmic rays can be conducted along current sheets, they can enter the heliosphere more easily through these regions, such as the heliospheric current sheet (HCS), which extends through the heliosphere in a wavy shape caused by the non-alignment between the Sun’s rotational and magnetic axes (Mewaldt et al., 2010). Since these cosmic rays can penetrate even into the inner heliosphere (Cooper et al., 1995; Kudela and Bucik, 2013; Mrigakshi et al., 2013; Kress et al., 2015), we can infer properties of the “thickness” of the heliosphere from the measured densities and energies of GCRs throughout the heliosphere. These properties can give us a global idea of the shape of the heliosphere but are also solar cycle-dependent. As the Sun becomes more active as the magnetic poles flip during the 11-year solar cycle, the heliosphere is inflated, there is an increase in transient events, and the HCS becomes more inclined, resulting in reduced penetration of GCRs into the heliosphere (Mewaldt et al., 2010; Kress et al., 2015).
Anisotropies in maps created from cosmic ray measurements can provide another means of enhancing our understanding of the interaction of the heliosphere with the LISM. Measurements from ground-based observatories such as IceCube, Milagro, and ASγ of cosmic rays up to TeV can be used in conjunction with measurements from space-based missions such as IBEX and future outer heliosphere missions to better understand these interactions (Schwadron et al., 2014). It is also possible to indirectly measure the features of the Sun’s influence by measuring gamma rays produced from the interaction of GCRs with the Sun’s surface and photon field (Orlando and Strong, 2021). These gamma ray emissions from the Sun can be studied from keV up to TeV and are supported by measurements from x-ray telescopes and current high-energy and gamma ray telescopes. Therefore, high-energy telescopes, including current ground-based observatories, such as VERITAS (Weekes et al., 2002), MAGIC (Baixeras et al., 2004), HESS (Aharonian et al., 2006), CTA-N and CTA-S (Acharya et al., 2013), HAWC (Abeysekara et al., 2017), and LHAASO (Aharonian et al., 2021), as well as SWGO (Barres de Almeida, 2021) which is used in research and development, and future instruments such as AMEGO (Rando, 2017; Caputo et al., 2022) and GECCO (Orlando et al., 2022), should be used as companions for future missions to study heliosphere–interstellar interactions.
2.2 Theory and modeling
The current state of our understanding of the shape of the heliosphere is largely based on theory and modeling (Heerikhuisen et al., 2014; Drake et al., 2015; Opher et al., 2015; Pogorelov et al., 2015; Dialynas et al., 2017; Izmodenov and Alexashov, 2020; Opher et al., 2020; Reisenfeld et al., 2021). Small variations in model parameters and properties measured in the nose of the heliosphere, the leading edge in the direction of the Sun’s motion through the LISM, lead to significant differences in the projected shape. The global response of the heliosphere is additionally expected to fluctuate with solar activity and therefore solar cycle (McComas et al., 2017a; McComas et al., 2019; Dialynas et al., 2017; Kim et al., 2017; Dayeh et al., 2022), which is yet another element in the interplay of heliosphere–interstellar interactions and another factor in our uncertainty of the overall shape. The main features of the shape of the heliosphere include the nose; the tail, which can be defined relative to the Sun as the region of the heliosphere found in the direction opposite the Sun’s motion through the LISM; the size of the heliosphere; and the behavior of the magnetic field lines at the heliospheric boundaries. All of these properties, including the size of the heliosphere, vary vastly in different proposed shapes and models of the heliosphere, which range in size anywhere from hundreds to thousands of au and in shape from comet- or magnetosphere-like or otherwise with extended tails (Kivelson and Russell, 1995; Cravens, 1997; Heerikhuisen et al., 2014; Pogorelov et al., 2015; Chen, 2016; Izmodenov and Alexashov, 2020; Reisenfeld et al., 2021) to spherical (Dialynas et al., 2017) to croissant-shaped with multiple tail lobes (Opher et al., 2020), as shown in Figure 1.
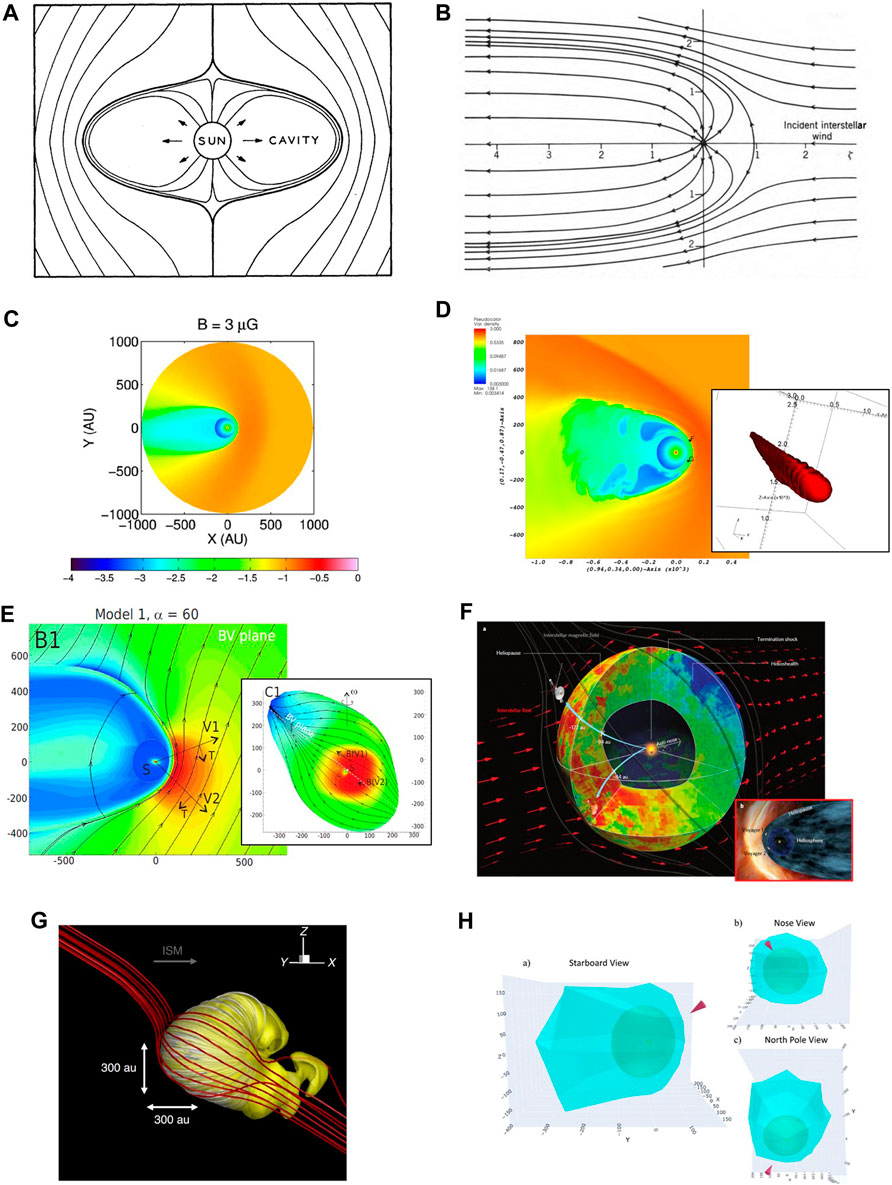
FIGURE 1. Various modeled and proposed shapes for the heliosphere including original 2-D gas dynamic models (Figures adapted from Davis, 1955; Parker, 1963) (A, B), non-spherical asymmetric models (Figures adapted from Heerikhuisen et al., 2014; Pogorelov et al., 2015; Izmodenov and Alexashov, 2020; Reisenfeld et al., 2021) (C–E, H), a spherical shape as compared with a more traditional magnetosphere-like concept (Figure adapted from Dialynas et al., 2017, references therein) (F), and a croissant-shape or small spherical shape with tail lobes (Figure adapted from Opher et al., 2020) (G). The models in (B–D) propose long tails extending in the downwind direction. The model in (E) depicts an asymmetric shape that is oblong and stretched in the tailward direction. The model in (F) proposes a very spherical shape, in which the heliotail is completely blunt and symmetric to the nose direction. This panel compares this model, depicted in the center of Panel (F), with an artistic depiction outlined in red and overlapping the bottom right corner of the model in Panel (F) that shows a heliotail that is very stretched in the tailward direction, extending beyond the bounds of the figure. The model in (G) depicts a shape in which the heliotail comprises two lobes that curve inward in the tailward direction. The model in (H) depicts an asymmetric, non-spherical shape that cannot be fully constrained in the tailward direction, other than to determine that the IBEX ENA measurements indicate a minimum distance of 350–400 au downstream along the tail. Such distance is consistent with both the croissant heliosphere (Opher et al., 2015; Kornbleuth et al., 2021) that predicts a tail extending to 350–400 au and with a heliosphere having a comet-like shape that extends to further distances. While there is currently no definitive proof or consensus as to the actual shape of the heliotail, these models represent a sampling of theorized shapes for the heliotail from the literature.
2.2.1 Elongated shape
Various stretched heliospheric models have been proposed since Parker predicted the supersonic solar wind flow in 1958, including the 2-D model presented in Parker (1963) shown in Figure 1B, compared with the earlier more simplified dipole-like structure presented in Davis (1955) shown in Figure 1A. A few representations of modern models of elongated heliospheric shapes are presented here. As shown in Figure 1C, Heerikhuisen et al. (2014) present a 3-D kinetic–magnetohydrodynamic (MHD) model of the heliosphere based on a simulation of the solar wind interaction with the LISM. The model parameters are adjusted according to IBEX measurements. These measurements are used to set the LISM temperature and velocity and to constrain the LISM H and H+ densities, especially to keep the distance to the TS below 90 au in the directions of the Voyager spacecraft. The authors orient the magnetic field toward the center of the IBEX ribbon. The reported simulation results yield a tail that stretches downwind, while its structure, as well as properties for the plasma and neutrals in the outer heliosheath, is dependent on ISMF strength. The authors simulate field strengths of 1, 2, 3, and 4 μG and find that a value in the range 2.5–3 μG yields results most consistent with the IBEX data. Additionally, Pogorelov et al. (2015) present simulation results as shown in Figure 1D using LISM property constraints from Voyager, IBEX, SOHO, and air shower observations that yield a long heliotail extending more than 5000 au downwind of the Sun.
As shown in Figure 1E, Izmodenov and Alexashov (2020) present a kinetic–MHD model of the heliosphere compared with magnetic field measurements at the heliopause taken by Voyagers 1 and 2. This model shows that disturbances in the magnetic field induced by solar activity can be measured as far away as 400–500 au. The model produces various results given the different constraints, resulting in potentially blunt or oblong heliopauses with overall asymmetric shapes and a stretched tail. The authors state that while the shape of the heliopause is influenced by the ISMF, it is not entirely determined by the ISMF, but rather is influenced by the Sun, namely, the solar wind dynamic pressure and IMF as well as transient variations, to a lesser extent. These effects are incorporated into the model. The authors note that the model and spacecraft measurements show discrepancies in the radial component of the ISMF, which may be related to temporal compressions in the heliosphere as well as averaging in the model that may reduce the effects of instabilities at the heliopause. The authors conclude that their Model 1, the model which aligns the ISMF with the hydrogen deflection plane, is most consistent with Voyager measurements. Out of approximately 50 parameterizations of their model with various constraints, the authors find this one to be the most consistent with the data. However, there are discrepancies that are yet to be resolved.
2.2.2 Bubble-like shape
As shown compared with a magnetosphere-like concept of the heliosphere in Figure 1F, Dialynas et al. (2017) model the heliosphere using ENA maps taken from Cassini data and compare with ion measurements taken by Voyagers 1 and 2 in the heliosheath over an 11-year solar cycle. The results of this model suggest a bubble-like shape to the heliosphere, with no significant features extending the shape in the heliotail. The authors suggest that a mechanism for outflow of particles from the system could be polar jet-like structures as suggested by other modeling efforts. This model also suggests that the heliosphere does not have a bow shock. The results of this model may be supported by the fact that similar shapes were observed when simulating the Ganymede magnetosphere embedded in the sub-Alfvenic Jovian magnetospheric plasma. The authors suggest that the shape can be further constrained in the future by additional dedicated ENA detections and in situ measurements.
2.2.3 Small, round shape with tail jets
As shown in Figure 1G, Opher et al. (2020) model the heliosphere using a two-fluid MHD approach that treats the thermal solar wind and PUIs as separate fluids. This model produces a smaller, rounder heliosphere with two jet-like structures in the heliotail region. Turbulence and magnetic reconnection between the IMF and ISMF are seen mainly in the flanks in the heliotail, as highlighted in Figure 2 (Figures from Opher et al., 2017; Opher et al., 2020). Since the interstellar parameters, namely, the magnetic field strength and direction, are not well-constrained by in situ measurements, Opher et al. (2020) present two cases using potential interstellar magnetic field vectors. The two-fluid approach allows the authors to reproduce some of the cooling and thinning of the heliosheath that occurs when the PUIs undergo charge exchange with neutrals and leave the system as energetic neutral atoms (ENAs). The PUI depletion shifts the magnetic field enhancement downstream near the heliopause, influencing the overall shape. The large gradient in PUI pressure in the heliosheath additionally causes faster flows toward the north and south, additionally compressing the heliosphere. The authors, therefore, present a smaller and rounder model, suggesting this shape is in line with Cassini ENA observations. The authors find that their second case, which places the interstellar magnetic field along the center of the IBEX ribbon, is consistent with the Voyager 1 and 2 measurements that indicate that the magnetic field strength increases at the heliopause but also that the direction does not change. Their first case, with the magnetic field in the hydrogen deflection plane, does not exhibit this behavior. They also find that their model allows for magnetic reconnection in the heliotail, though it is suppressed in the nose. While the authors are able to show many consistencies with existing spacecraft measurements from Cassini, New Horizons, and Voyagers 1 and 2, there are still discrepancies, such as a significant discrepancy between the models and the thinner heliosheath measured by the Voyagers 1 and 2 spacecraft. Future remote and in situ measurements are needed to confirm the results of these models. Furthermore, Opher et al. (2020) show the PUIs to be located in a spherical region, which might indicate consistency with the results obtained with ENA emissions seen by INCA (Dialynas et al., 2017).
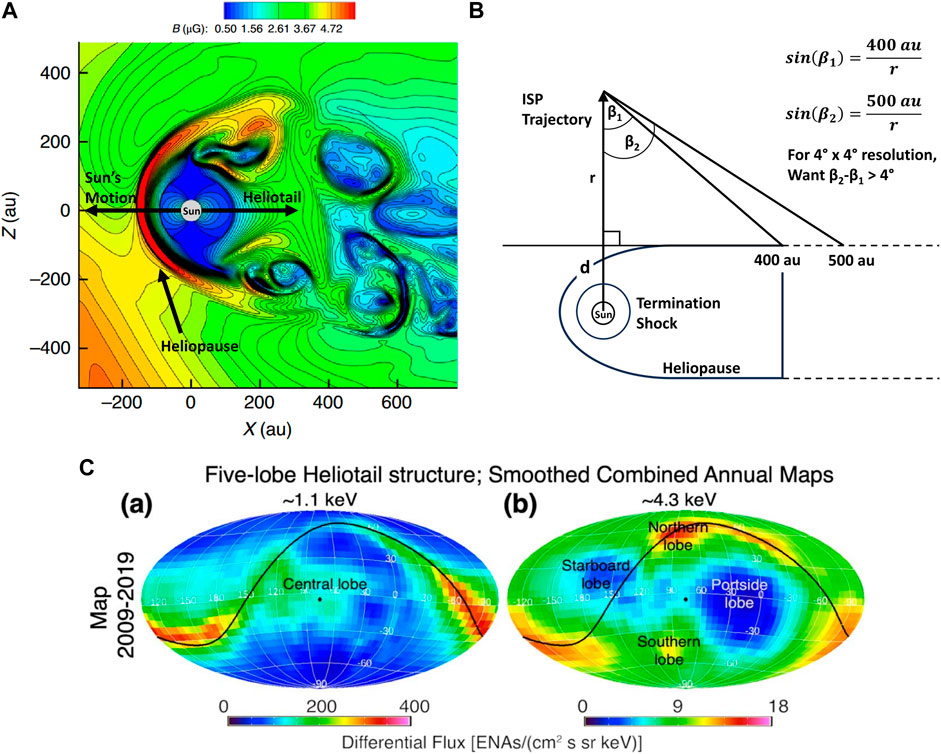
FIGURE 2. (A) Magnetic reconnection in the heliotail is seen in some current models of the heliosphere, such as the global heliosphere model treating PUIs as a separate fluid shown in this figure modified from Opher et al. (2020). If magnetic reconnection is occurring in the heliotail between the IMF and ISMF, it is reasonable to assume there will be injection into the heliosphere of interstellar ions. (B) Sample geometry for calculating the distance an Interstellar Probe would need to travel to resolve 100 au of the heliotail within two pixels. (C) Figure adapted from Dayeh et al. (2022), showing the heliospheric lobe structure observed by IBEX in ENAs, which can be used as a reference point for prospective probe trajectories.
2.2.4 Asymmetric, potentially bullet-like shape
As shown in Figure 1H, Reisenfeld et al. (2021) use a “sounding” method to build a 3D map of the heliosphere by measuring the average distance to the ENA source region throughout the heliosphere. This is done by correlating variations in the outbound pressure of the solar wind from 1 au and measuring the time variation, or “trace-back” time, to fluctuations in the ENA measurements made by IBEX over the course of one 11-year solar cycle. The authors filter out the enhancements from the IBEX ribbon and use the sounding method to determine a lower limit on the distance to the heliopause in all directions. These results are compared with Voyager 1 and 2 measurements. The authors present three models, the first of which uses a uniform TS distance of 100 au as a baseline to demonstrate that the shape of the heliopause is not significantly impacted by the shape of the TS to show the stability of the sounding method. The second model uses a Voyager-derived TS model, which only gives hard constraints along the trajectories of Voyagers 1 and 2. The third model uses a Z-H TS shape determined by solar wind dynamic pressure, which is roughly latitude-dependent. This shape is not significantly impacted by the solar cycle and is constrained by the Voyager measurements, so it is considered the most accurate by the authors, though it exhibits an unexplained asymmetry of ∼10 au. The authors state that the shape of the heliosphere is primarily determined by the interaction of the heliosphere with the LISM flow and secondarily affected by the ISMF. The results of the sounding models yield a minimum distance of 350–400 au downstream. The authors note that the overall shape obtained is more consistent with a “bullet-like” (Heerikhuisen et al., 2014; Pogorelov et al., 2015; Izmodenov and Alexashov, 2020) shape than a “croissant” (Opher et al., 2015; Opher et al., 2020) shape. However, while this method uses spacecraft measurements to remotely sample the shape of the heliosphere, this sounding method is limited in scale and cannot determine an upper bound on the length of the heliotail to determine if the overall shape of the heliosphere is more compressed or stretched. The limitations of this uniquely data-driven model of the heliosphere greatly emphasize the need for more in situ measurements required to more fully understand the shape of the heliosphere. Additionally, heliotail model comparisons in Kornbleuth et al. (2021) show that differences in the heliosheath plasma for different heliotail configurations do not begin to manifest until approximately 350–400 au, which coincides with the observational limit of the IBEX-Hi observations noted by Reisenfeld et al. (2021).
2.3 Interstellar measurements and heliotail observations
To address these unknowns about the shape and properties of the heliosphere, direct interstellar measurements must be taken outside of the heliosphere, away from the Sun’s direct influence. Remotely sampling the LISM from within the heliosphere has its limitations and cannot give the complete picture of the full heliosphere and its interactions with the surrounding LISM. Only direct measurements from the LISM can definitively address the open questions of how the heliosphere is affected by interstellar interactions and better constrain the boundary conditions for heliospheric models. This can be achieved as part of a future Interstellar Probe mission, as discussed by McNutt Jr. et al. (2021), Brandt et al. (2022), and Brandt et al. (2023). However, a single-spacecraft Interstellar Probe mission comes with its own limitations. The most significant difference among the proposed shapes of the heliosphere can be found in the tail region, but a likely trajectory of a future Interstellar Probe may be noseward (Schlei et al., 2021). While it is important to sample the LISM noseward of the heliosphere to understand the interstellar space environment ahead of the heliosphere to understand where the solar system is headed and what the future implications may be on future composition and dynamics within the solar system, it is also critical to properly understand the shape of the entire heliosphere. Only a study of the tailward region of the heliosphere will give definitive evidence for the complete shape, which impacts how the heliosphere interacts with the LISM and therefore how the LISM impacts the composition of the heliosphere. Therefore, complementary in situ and indirect interstellar measurements must be made tailward within the heliosphere. These measurements can be made through the use of intentional instrumentation requirements for outer heliosphere missions. Additionally, it would be beneficial for the Interstellar Probe mission to either consider a trajectory through the heliotail, via the flank, or to comprise a unified mission of two spacecraft, in which a second Interstellar Probe would be launched with a tailward trajectory, perhaps intersecting one of the proposed (Opher et al., 2020) tail lobes. A two-part Interstellar Probe mission could be seen as an analog to the two Voyager spacecraft which were deployed as part of a unified mission to visit each of the planets within the solar system. Such an Interstellar Probe mission would additionally have the potential for interdisciplinary science, such as planetary science, as proposed by Holler et al. (2023).
Intentional tailward measurements included in future mission requirements using PUIs, ENAs, low-energy neutrals, and cosmic rays will better inform our understanding of the global shape of the heliosphere and its interaction with the LISM. Furthermore, in addition to in situ remote sensing techniques, it may be possible to take direct measurements of interstellar ions from within the heliosphere, especially from the heliotail. While most of the interstellar ions at the nose of the heliosphere are deflected around the heliosphere by the boundary with the IMF, interstellar ions may penetrate the heliosphere through other mechanisms. There may be reconnection sites between the IMF and ISMF and further mixing that lead to the entry of interstellar particles into the heliosphere. Given heliospheric features such as tail lobes or jets or a magnetosphere-like heliotail, there is likely injection of interstellar plasma into the heliosphere through magnetic reconnection and turbulent mixing in the heliotail, even if this effect is more of a “leaking in” of interstellar plasma rather than the more eruptive events which can be caused in a magnetosphere due to solar activity. The likelihood of magnetic reconnection between the IMF and ISMF in the heliotail is supported by current modeling efforts such as the separate fluid PUI model shown in Figure 2 (Opher et al., 2020). Such modeling efforts support reconnection in the tail, allowing for the injection of interstellar material through the heliotail (Opher et al., 2021). Furthermore, given any shape of the heliosphere, some amount of tailward mixture of the heliospheric and interstellar plasmas should result, which should likely have detectable signatures from within the heliosphere, especially as there is evidence for turbulence and vortices in the heliotail and other astrotails (Pauls and Zank, 1996; Sahai and Chronopoulos, 2010; Desiati and Lazarian, 2012; Opher et al., 2017).
Flow behavior of the LISM around the heliosphere resulting in turbulence and reconnection is generally noticeable in models of the heliosphere from the literature (Opher et al., 2015; Pogorelov et al., 2015). Therefore, as some amount of mixing of the heliospheric and interstellar plasmas should occur in the heliotail region, it is reasonable to assume that these interstellar particles may be measured away from the Sun, even within the heliosphere. Sampling these interstellar particles will both allow us to measure additional interstellar properties and to further constrain the global shape of the heliosphere. In fact, such plasma flows may be found to reconcile some of the critical differences between currently proposed heliospheric shapes, such as a stretched vs. croissant-like shape. Therefore, direct measurement of interstellar particles from within the heliosphere should be explicitly included in the mission goals for future outer heliosphere missions.
2.4 Possible trajectories for an Interstellar Probe mission
A number of viable trajectories may be considered for a future Interstellar Probe mission, namely, six possible trajectories, each with notable advantages and disadvantages. Specifically, these possibilities include trajectories through the nose, through the IBEX Ribbon center, through the port lobe, through the starboard lobe, through the northern heliotail lobe, and through the low-latitude tail.
2.4.1 Through the nose
Passing through the nose direction of the heliosphere, which is (7°N, 252°E) in Earth ecliptic coordinates, offers an opportunity to connect the Voyager observations, being close to the midpoint of their trajectories. It also offers the opportunity to effectively revisit the Voyager observations by evaluating how the physical processes observed by Voyagers 1 and 2 changed with time to help determine which observed phenomena were temporal or spatial. Additionally, the lifetimes of the Voyager 1 and 2 spacecraft will not be able to extend beyond 200 au, and both spacecraft are currently limited in their instrumentation. An Interstellar Probe in this direction would be able to expand upon previous observations to provide a more complete view of the LISM environment in front of the heliosphere. The nose direction also provides an interesting opportunity, as measurements through this direction would provide important observations of the bow wave in front of the heliosphere. This trajectory also offers the shortest path to the heliopause to begin this sampling of the bow wave. This would additionally help to indicate, through global imaging, the spatial regions over which the IBEX Ribbon is created, as the spacecraft would pass through the radial distances of the ribbon source locations. Despite these advantages, this direction poses several limitations, specifically regarding breakthrough science. Primarily, the plasma environment along this direction would likely be similar to the plasma environment investigated by both Voyager spacecraft, as well as New Horizons. Additionally, there would be no opportunity to investigate the physics or structure of the heliotail given this trajectory, limiting our observational perspective strictly to the upwind hemisphere.
2.4.2 Through the IBEX ribbon center
This trajectory is a highlighted trajectory in the Interstellar Probe Mission Concept Report (McNutt Jr. et al., 2021). The main appeal of this trajectory, which is approximately 45° from the heliopause nose direction, is that the heliopause and the LISM can be reached within a reasonable timeframe (
2.4.3 Through the port lobe
While a port lobe trajectory (Figure 2) would require a further distance to the heliopause than through the IBEX ribbon center as described above, this trajectory offers significant advantages. As first noted in McComas et al. (2013), the port lobe is a low-/mid-latitude region of the heliosphere on the port side, or flank, relative to the motion of the heliosphere through the LISM. In IBEX-Hi observations, the port lobe has the signature of a high spectral slope (McComas et al., 2013; Zirnstein et al., 2016a; Dayeh et al., 2022). In general, by passing through the flank of the heliosphere where there is a thicker heliosheath, there are not only more opportunities to learn about the physics of the heliosheath, but also to observe diverted flows from the nose region of the heliosphere. Opher et al. (2023) suggest that anisotropies seen in Voyager energetic ion measurements result from pressure gradients between the flanks and nose of the heliosphere. A trajectory through the flanks, therefore, when taken with previous Voyager measurements, offers not only the opportunity to investigate a potential source of previously observed PUI anisotropies but also to construct a better global picture of plasma flows in different regions of the heliosheath. This is an important implication due to the observed ENA depletion regions in IBEX observations at energies
2.4.4 Through the starboard lobe
Passing through the starboard lobe (Figure 2), which is the low-/mid-latitude region of the high spectral slope (McComas et al., 2013; Zirnstein et al., 2016a; Dayeh et al., 2022) on the starboard side of the moving heliosphere, offers many of the same advantages as passing through the port lobe. Some differences, however, can be noted from the port lobe trajectory. In contrast to the port lobe, the presently accepted ISMF draping configuration along the heliopause suggests a longer heliopause distance in the starboard flank than in the port flank (Section 2.4.3). This would have implications not only for the heliopause but also for the termination shock. This trajectory has the potential to increase the amount of time an Interstellar Probe would spend reaching the LISM, the reduction of which is considered a primary mission goal. However, it also offers the opportunity to help better characterize the global shape of the heliosphere. In addition, the starboard lobe trajectory offers the potential opportunity to directly pass through the IBEX ribbon in the ecliptic plane, which could help reveal the IBEX ribbon source physics and location for the segment of the ribbon through which it passes. This trajectory offers the advantage of traversing a similar distance within the heliosphere as compared with the port lobe trajectory to obtain a side-view global ENA image from outside that can capture heliotail distances. Passing through the starboard lobe, therefore, offers the best opportunity to both directly study the IBEX ribbon and to characterize the shape of the heliotail.
2.4.5 Through the northern heliotail lobe
Passing through the heliotail offers the best opportunity to study the dynamics of the downwind hemisphere. However, given a single Interstellar Probe spacecraft, this direction would offer the least opportunity to investigate both the heliosphere and the LISM. Should an Interstellar Probe mission include two spacecraft, however, the heliotail offers an ideal trajectory for better characterizing the global picture of the heliotail. Specifically, passing through the northern heliotail lobe (Figure 2) explores a region of great interest in the heliophysics community. The northern heliotail lobe can readily be identified in ENA observations as being located near ∼45° in latitude from the ecliptic plane in the heliotail and having higher intensity in IBEX-Hi ENA maps than the surrounding area at energies
2.4.6 Through the low-latitude tail
The primary goal of this trajectory would be to determine the extent of the heliotail. In the low-latitude tail, Kornbleuth et al. (2021) show that differences between a long and short heliotail manifest around 400 au. Reconnection between the ISMF and IMF is shown to be present beyond 400 au in the short-tail scenario, along with mixed interstellar and solar wind plasma (Opher et al., 2015). A trajectory through this region should allow an Interstellar Probe to address whether or not this mixing region exists to help evaluate the structure and physics of the heliotail. Despite this potential, notable limitations of this trajectory include a null result should the spacecraft not encounter a mixing region, as well as a potential lack of ability to study the LISM and the IBEX ribbon. Additionally, should the spacecraft enter the mixed region of solar wind and interstellar plasma, it would be unable to make determinations on the pristine LISM conditions. This trajectory would, therefore, be another option that should likely only be considered given a two-spacecraft Interstellar Probe mission, though more science objectives would still likely be obtained from other trajectories.
3 Conclusion
The intent of this paper is to address the science question: what is the global shape of the heliosphere, especially in the heliotail, and how does the LISM interact with, penetrate, and affect the composition of the heliosphere? To address this question, we propose intentional in situ and indirect measurements of interstellar properties as a mission requirement for future missions through: 1. More comprehensive PUI measurements taken with improved sensitivity, higher cadence, and larger fields of view, especially aimed at interstellar measurements and the heliotail. 2. Use of cosmic ray measurements, ENAs, and low-energy neutrals in the heliotail. 3. Measurements of interstellar ions that penetrate the heliosphere through magnetic reconnection, turbulence, and other mechanisms, along with their signatures, especially in the heliotail. 4. Either an Interstellar Probe trajectory through the heliotail, via the flank, or a two-spacecraft Interstellar Probe mission with noseward and heliotailward trajectories. We additionally provide the background on the current state of knowledge and modeling in the field and present the benefits and shortcomings of six possible Interstellar Probe trajectories ranging from the nose to the tail directions.
Data availability statement
The original contributions presented in the study are included in the article/Supplementary Material; further inquiries can be directed to the corresponding author.
Author contributions
The manuscript was compiled by SS and MK with conceptual and editorial contributions from all co-authors. All authors contributed to the article and approved the submitted version.
Funding
This work is supported by NASA’s Solar Orbiter, Advanced Composition Explorer, and WIND missions as well as NASA grants AGS 1358268 for JG and SL; NNX16AP03H, 80NSSC20K0192, and 80NSSC19K0457 for SL; and NASA grant 18-DRIVE18_2-0029, Our Heliospheric Shield, 80NSSC22M0164, for MO and MK.
Acknowledgments
A modified version of this paper was submitted to the 2024 Solar and Space Physics Decadal Survey and is published in the associated issue of the Bulletin of the American Astronomical Society. MK would like to acknowledge helpful discussions with Dr. Jesse Miller.
Conflict of interest
The authors declare that the research was conducted in the absence of any commercial or financial relationships that could be construed as a potential conflict of interest.
Publisher’s note
All claims expressed in this article are solely those of the authors and do not necessarily represent those of their affiliated organizations, or those of the publisher, the editors, and the reviewers. Any product that may be evaluated in this article, or claim that may be made by its manufacturer, is not guaranteed or endorsed by the publisher.
References
Abeysekara, A. U., Albert, A., Alfaro, R., Alvarez, C., Álvarez, J. D., Arceo, R., et al. (2017). The 2HWC HAWC observatory gamma-ray catalog. Astrophysical J. 843, 40. doi:10.3847/1538-4357/aa7556
Acharya, B. S., Actis, M., Aghajani, T., Agnetta, G., Aguilar, J., Aharonian, F., et al. (2013). Introducing the CTA concept. Astropart. Phys. 43, 3–18. doi:10.1016/j.astropartphys.2013.01.007
Aharonian, F., Akhperjanian, A. G., Bazer-Bachi, A. R., Beilicke, M., Benbow, W., Berge, D., et al. (2006). Observations of the crab nebula with HESS. Astronomy Astrophysics 457, 899–915. doi:10.1051/0004-6361:20065351
Aharonian, F., An, Q., Axikegu, , Bai, L. X., Bai, Y. X., Bao, Y. W., et al. (2021). Observation of the crab nebula with LHAASO-km2a - a performance study. Chin. Phys. C 45, 025002. doi:10.1088/1674-1137/abd01b
Ajello, J. M. (1978). An interpretation of Mariner 10 helium (584 Å) and hydrogen (1216 Å) interplanetary emission observations. Astrophysical J. 222, 1068–1079. doi:10.1086/156224
Axford, W. I. (1972). “The interaction of the solar wind with the interstellar medium,”. NASA special publication. Editors C. P. Sonett, P. J. Coleman, and J. M. Wilcox, 308, 609.
Axford, W. I., Dessler, A. J., and Gottlieb, B. (1963). Termination of solar wind and solar magnetic field. Astrophysical J. 137, 1268. doi:10.1086/147602
Baalmann, L. R., Scherer, K., Fichtner, H., Kleimann, J., Bomans, D. J., and Weis, K. (2020). Skymaps of observables of three-dimensional magnetohydrodynamic astrosphere models. Astronomy Astrophysics 634, A67. doi:10.1051/0004-6361/201937017
Baalmann, L. R., Scherer, K., Kleimann, J., Fichtner, H., Bomans, D. J., and Weis, K. (2022). Modelling O-star astrospheres with different relative speeds between the ISM and the star: 2D and 3D MHD model comparison. Astronomy Astrophysics 663, A10. doi:10.1051/0004-6361/202243525
Baixeras, C., Bastieri, D., Bigongiari, C., Blanch, O., Blanchot, G., Bock, R., et al. (2004). Commissioning and first tests of the MAGIC telescope. Nucl. Instrum. Methods Phys. Res. A 518, 188–192. doi:10.1016/j.nima.2003.10.057
Baranov, V. B., Krasnobaev, K. V., and Kulikovskii, A. G. (1971). A model of the interaction of the solar wind with the interstellar medium. Sov. Phys. Dokl. 15, 791.
Barres de Almeida, U. (2021). The southern wide field gamma ray observatory. Astron. Nachrichten 342, 431–437. doi:10.1002/asna.202113946
Bertaux, J. L., and Blamont, J. E. (1971). Evidence for a source of an extraterrestrial hydrogen lyman-alpha emission. Astronomy Astrophysics 11, 200.
Bertaux, J. L., Lallement, R., Kurt, V. G., and Mironova, E. N. (1985). Characteristics of the local interstellar hydrogen determined from PROGNOZ 5 and 6 interplanetary Lyman-alpha line profile measurements with a hydrogen absorption cell. Astronomy Astrophysics 150, 1–20.
Blandford, R. D., and Ostriker, J. P. (1978). Particle acceleration by astrophysical shocks. Astrophysical J. Lett. 221, L29–L32. doi:10.1086/182658
Bower, J. S., Möbius, E., Berger, L., Farrugia, C., Keilbach, D., Lee, M. A., et al. (2019). Effect of rapid changes of solar wind conditions on the pickup ion velocity distribution. J. Geophys. Res. (Space Phys. 124, 6418–6437. doi:10.1029/2019JA026781
Brandt, P., Alterman, B., Alvarez, E., Baker, D., Bale, S., Baliukin, I., et al. (2023). Pushing the frontier of solar & space physics: exploration of the heliosphere and the very local interstellar medium by an interstellar probe. Bull. Am. Astronomical Soc. 55, 038. doi:10.3847/25c2cfeb.e0bf48d5
Brandt, P. C., Provornikova, E., Bale, S., Cocoros, A., DeMajistre, R., Dialynas, K., et al. (2022). Future exploration of the outer heliosphere and very local interstellar medium by interstellar probe. Space Sci. Rev. 219, 18. doi:10.1007/s11214-022-00943-x
Bzowski, M., Kubiak, M. A., Hłond, M., Sokół, J. M., Banaszkiewicz, M., and Witte, M. (2014). Neutral interstellar He parameters in front of the heliosphere 1994-2007. Astronomy Astrophysics 569, A8. doi:10.1051/0004-6361/201424127
Bzowski, M., Swaczyna, P., Kubiak, M. A., Sokół, J. M., Fuselier, S. A., Galli, A., et al. (2015). Interstellar neutral helium in the heliosphere from IBEX observations. III. Mach number of the flow, velocity vector, and temperature from the first six years of measurements. Astrophysical J. Suppl. 220, 28. doi:10.1088/0067-0049/220/2/28
Caputo, R., Ajello, M., Kierans, C. A., Perkins, J. S., Racusin, J. L., Baldini, L., et al. (2022). All-sky medium energy gamma-ray observatory eXplorer mission concept. J. Astronomical Telesc. Instrum. Syst. 8, 044003. doi:10.1117/1.JATIS.8.4.044003
Chen, F. F. (2016). Introduction to plasma physics and controlled fusion. Springer. doi:10.1007/978-3-319-22309-4
Cooper, J. F., Chen, J., Guzik, T. G., Sang, Y., and Wefel, J. P. (1995). Cosmic ray heavy ion penetration deep into the magnetosphere: results from S81-1 and CRRES. Adv. Space Res. 15, 61–64. doi:10.1016/S0273-1177(99)80127-6
Cox, N. L. J., Kerschbaum, F., van Marle, A. J., Decin, L., Ladjal, D., Mayer, A., et al. (2012). A far-infrared survey of bow shocks and detached shells around AGB stars and red supergiants. Astronomy Astrophysics 537, A35. doi:10.1051/0004-6361/201117910
Cravens, T. E. (1997). “Physics of solar system plasmas,” in Cambridge atmospheric and space science series (Cambridge University Press). doi:10.1017/CBO9780511529467
Czechowski, A., and Grygorczuk, J. (2022). Closing of the astrotail. Astronomy Astrophysics 666, A197. doi:10.1051/0004-6361/202243297
Dalaudier, F., Bertaux, J. L., Kurt, V. G., and Mironova, E. N. (1984). Characteristics of interstellar helium observed with Prognoz 6 58.4-nm photometers. Astronomy Astrophysics 134, 171–184.
Davis, L. (1955). Interplanetary magnetic fields and cosmic rays. Phys. Rev. 100, 1440–1444. doi:10.1103/PhysRev.100.1440
Dayeh, M. A., Zirnstein, E. J., Fuselier, S. A., Funsten, H. O., and McComas, D. J. (2022). Evolution of the heliotail lobes over a solar cycle as measured by IBEX. Astrophysical J. 261, 27. Supplement 261. doi:10.3847/1538-4365/ac714e
Decker, R. B., Krimigis, S. M., Roelof, E. C., Hill, M. E., Armstrong, T. P., Gloeckler, G., et al. (2005). Voyager 1 in the foreshock, termination shock, and heliosheath. Science 309, 2020–2024. doi:10.1126/science.1117569
Decker, R. B., Krimigis, S. M., Roelof, E. C., Hill, M. E., Armstrong, T. P., Gloeckler, G., et al. (2008). Mediation of the solar wind termination shock by non-thermal ions. Nature 454, 67–70. doi:10.1038/nature07030
Desiati, P., and Lazarian, A. (2012). Cosmic rays and stochastic magnetic reconnection in the heliotail. Nonlinear Process. Geophys. 19, 351–364. doi:10.5194/npg-19-351-2012
Dialynas, K., Krimigis, S. M., Decker, R. B., Hill, M., Mitchell, D. G., Hsieh, K. C., et al. (2022). The structure of the global heliosphere as seen by in-situ ions from the Voyagers and remotely sensed ENAs from Cassini. Space Sci. Rev. 218, 21. doi:10.1007/s11214-022-00889-0
Dialynas, K., Krimigis, S. M., Decker, R. B., and Mitchell, D. G. (2019). Plasma pressures in the heliosheath from Cassini ENA and voyager 2 measurements: validation by the voyager 2 heliopause crossing. Geophys. Res. Lett. 46, 7911–7919. doi:10.1029/2019GL083924
Dialynas, K., Krimigis, S. M., Mitchell, D. G., Decker, R. B., and Roelof, E. C. (2017). The bubble-like shape of the heliosphere observed by Voyager and Cassini. Nat. Astron. 1, 0115. doi:10.1038/s41550-017-0115
Dialynas, K., Krimigis, S. M., Mitchell, D. G., Roelof, E. C., and Decker, R. B. (2013). A three-coordinate system (ecliptic, galactic, ISMF) spectral analysis of heliospheric ENA emissions using Cassini/INCA measurements. Astrophysical J. 778, 40. doi:10.1088/0004-637X/778/1/40
Drake, J. F., Swisdak, M., and Opher, M. (2015). A model of the heliosphere with jets. Astrophysical J. Lett. 808, L44. doi:10.1088/2041-8205/808/2/L44
Drews, C., Berger, L., Wimmer-Schweingruber, R. F., Bochsler, P., Galvin, A. B., Klecker, B., et al. (2012). Inflow direction of interstellar neutrals deduced from pickup ion measurements at 1 AU. J. Geophys. Res. (Space Phys. 117, A09106. doi:10.1029/2012JA017746
Fahr, H. J. (1968). Neutral corpuscular energy flux by charge-transfer collisions in the vicinity of the Sun. Astrophysics Space Sci. 2, 496–503. doi:10.1007/BF02175924
Ferrière, K. M. (2001). The interstellar environment of our galaxy. Rev. Mod. Phys. 73, 1031–1066. doi:10.1103/RevModPhys.73.1031
Fields, B. D., Athanassiadou, T., and Johnson, S. R. (2008). Supernova collisions with the heliosphere. Astrophysical J. 678, 549–562. doi:10.1086/523622
Fields, B. D., Melott, A. L., Ellis, J., Ertel, A. F., Fry, B. J., Lieberman, B. S., et al. (2020). Supernova triggers for end-Devonian extinctions. Proc. Natl. Acad. Sci. 117, 21008–21010. doi:10.1073/pnas.2013774117
Fisk, L. A., and Gloeckler, G. (2022). Global structure and dominant particle acceleration mechanism of the heliosheath: definitive conclusions. Astrophysical J. 927, 73. doi:10.3847/1538-4357/ac4d2f
Fraternale, F., Pogorelov, N. V., and Bera, R. K. (2023). The role of electrons and helium atoms in global modeling of the heliosphere. Astrophysical J. 946, 97. doi:10.3847/1538-4357/acba10
Frisch, P. C. (2009). Is the Sun embedded in a typical interstellar cloud? Connecting interstellar gas in and out of the heliosphere. Space Sci. Rev. 143, 191–204. doi:10.1007/s11214-008-9394-4
Frisch, P. C., Bzowski, M., Drews, C., Leonard, T., Livadiotis, G., McComas, D. J., et al. (2015). Correcting the record on the analysis of IBEX and STEREO data regarding variations in the neutral interstellar wind. Astrophysical J. 801, 61. doi:10.1088/0004-637X/801/1/61
Frisch, P. C., Bzowski, M., Grün, E., Izmodenov, V., Krüger, H., Linsky, J. L., et al. (2009). The galactic environment of the Sun: interstellar material inside and outside of the heliosphere. Space Sci. Rev. 146, 235–273. doi:10.1007/s11214-009-9502-0
Frisch, P. C., Bzowski, M., Livadiotis, G., McComas, D. J., Moebius, E., Mueller, H. R., et al. (2013). Decades-long changes of the interstellar wind through our solar system. Science 341, 1080–1082. doi:10.1126/science.1239925
Frisch, P. C., Piirola, V., Berdyugin, A. B., Heiles, C., Cole, A., Hill, K., et al. (2022). Whence the interstellar magnetic field shaping the heliosphere? Astrophysical J. Suppl. 259, 48. doi:10.3847/1538-4365/ac5750
Fuselier, S. A., Allegrini, F., Funsten, H. O., Ghielmetti, A. G., Heirtzler, D., Kucharek, H., et al. (2009a). Width and variation of the ENA flux ribbon observed by the interstellar boundary explorer. Science 326, 962–964. doi:10.1126/science.1180981
Fuselier, S. A., Bochsler, P., Chornay, D., Clark, G., Crew, G. B., Dunn, G., et al. (2009b). The IBEX-lo sensor. Space Sci. Rev. 146, 117–147. doi:10.1007/s11214-009-9495-8
Galvin, A. B., Kistler, L. M., Popecki, M. A., Farrugia, C. J., Simunac, K. D. C., Ellis, L., et al. (2008). The plasma and suprathermal ion composition (PLASTIC) investigation on the STEREO observatories. Space Sci. Rev. 136, 437–486. doi:10.1007/s11214-007-9296-x
Gershman, D. J., Gloeckler, G., Gilbert, J. A., Raines, J. M., Fisk, L. A., Solomon, S. C., et al. (2013). Observations of interstellar helium pickup ions in the inner heliosphere. J. Geophys. Res. (Space Phys. 118, 1389–1402. doi:10.1002/jgra.50227
Giacalone, J., Nakanotani, M., Zank, G. P., Kòta, J., Opher, M., and Richardson, J. D. (2021). Hybrid simulations of interstellar pickup protons accelerated at the solar-wind termination shock at multiple locations. Astrophysical J. 911, 27. doi:10.3847/1538-4357/abe93a
Gilbert, J. A., Zurbuchen, T. H., and Battel, S. (2016). The pickup ion composition spectrometer. J. Geophys. Res. (Space Phys. 121, 5097–5104. doi:10.1002/2016JA022381
Gkioulidou, M., Opher, M., Kornbleuth, M., Dialynas, K., Giacalone, J., Richardson, J. D., et al. (2022). On the energization of pickup ions downstream of the heliospheric termination shock by comparing 0.52-55 keV observed energetic neutral atom spectra to ones inferred from proton hybrid simulations. Astrophysical J. Lett. 931, L21. doi:10.3847/2041-8213/ac6beb
Gloeckler, G., Cain, J., Ipavich, F. M., Tums, E. O., Bedini, P., Fisk, L. A., et al. (1998). Investigation of the composition of solar and interstellar matter using solar wind and pickup ion measurements with SWICS and SWIMS on the ACE spacecraft. Space Sci. Rev. 86, 497–539. doi:10.1023/A:1005036131689
Gloeckler, G., and Geiss, J. (2004). Composition of the local interstellar medium as diagnosed with pickup ions. Adv. Space Res. 34, 53–60. doi:10.1016/j.asr.2003.02.054
Gloeckler, G., Geiss, J., Balsiger, H., Bedini, P., Cain, J. C., Fischer, J., et al. (1992). The solar wind ion composition spectrometer. Astronomy Astrophysics 92, 267–289.
Gloeckler, G., Möbius, E., Geiss, J., Bzowski, M., Chalov, S., Fahr, H., et al. (2004). Observations of the helium focusing cone with pickup ions. Astronomy Astrophysics 426, 845–854. doi:10.1051/0004-6361:20035768
Gurnett, D. A., and Kurth, W. S. (2019). Plasma densities near and beyond the heliopause from the Voyager 1 and 2 plasma wave instruments. Nat. Astron. 3, 1024–1028. doi:10.1038/s41550-019-0918-5
Heerikhuisen, J., Zirnstein, E. J., Funsten, H. O., Pogorelov, N. V., and Zank, G. P. (2014). The effect of New interstellar medium parameters on the heliosphere and energetic neutral atoms from the interstellar boundary. Astrophysical J. 784, 73. doi:10.1088/0004-637X/784/1/73
Holler, B., Runyon, K., Ahrens, C., Annex, A., Bannister, M., Beddingfield, C., et al. (2023). Interstellar probe at the intersection of heliophysics & planetary science: probing endo- & exo-heliospheric environments with trans-neptunian objects. Bull. Am. Astronomical Soc. 55, 171. doi:10.3847/25c2cfeb.20f2e216
Izmodenov, V. V., and Alexashov, D. B. (2015). Three-dimensional kinetic-MHD model of the global heliosphere with the heliopause-surface fitting. Astrophysical J. Suppl. 220, 32. doi:10.1088/0067-0049/220/2/32
Izmodenov, V. V., and Alexashov, D. B. (2020). Magnitude and direction of the local interstellar magnetic field inferred from Voyager 1 and 2 interstellar data and global heliospheric model. Astronomy Astrophysics 633, L12. doi:10.1051/0004-6361/201937058
Katushkina, O. A., Alexashov, D. B., Gvaramadze, V. V., and Izmodenov, V. V. (2018). An astrosphere around the blue supergiant κ Cas: possible explanation of its filamentary structure. Mon. Notices R. Astronomical Soc. 473, 1576–1588. doi:10.1093/mnras/stx2488
Kim, T. K., Pogorelov, N. V., and Burlaga, L. F. (2017). Modeling shocks detected by voyager 1 in the local interstellar medium. Astrophysical J. Lett. 843, L32. doi:10.3847/2041-8213/aa7b2b
Kivelson, M. G., and Russell, C. T. (1995). Introduction to space physics. Cambridge, United Kingdom: Cambridge University Press, 586.
Knie, K., Korschinek, G., Faestermann, T., Wallner, C., Scholten, J., and Hillebrandt, W. (1999). Indication for supernova produced 60Fe activity on Earth. Phys. Rev. Lett. 83, 18–21. doi:10.1103/PhysRevLett.83.18
Kobulnicky, H. A., Chick, W. T., Schurhammer, D. P., Andrews, J. E., Povich, M. S., Munari, S. A., et al. (2016). A comprehensive search for stellar bowshock nebulae in the milky way: a catalog of 709 mid-infrared selected candidates. Astrophysical J. Suppl. Ser. 227, 18. doi:10.3847/0067-0049/227/2/18
Kohlhase, C. E., and Penzo, P. A. (1977). Voyager mission description. Space Sci. Rev. 21, 77–101. doi:10.1007/BF00200846
Kornbleuth, M., Opher, M., Baliukin, I., Dayeh, M. A., Zirnstein, E., Gkioulidou, M., et al. (2021). Signature of a heliotail organized by the solar magnetic field and the role of nonideal processes in modeled IBEX ENA maps: a comparison of the BU and Moscow MHD models. Astrophysical J. 921, 164. doi:10.3847/1538-4357/ac1e2a
Kornbleuth, M., Opher, M., Dialynas, K., Zank, G. P., Wang, B. B., Baliukin, I., et al. (2023a). Probing the length of the heliospheric tail with energetic neutral atoms (ENAs) from 0.52 to 80 keV. Astrophysical J. Lett. 945, L15. doi:10.3847/2041-8213/acbc73
Kornbleuth, M., Opher, M., Zank, G. P., Wang, B. B., Giacalone, J., Gkioulidou, M., et al. (2023b). An anomalous cosmic-ray mediated termination shock: implications for energetic neutral atoms. Astrophysical J. Lett. 944, L47. doi:10.3847/2041-8213/acb9e0
Kress, B. T., Hudson, M. K., Selesnick, R. S., Mertens, C. J., and Engel, M. (2015). Modeling geomagnetic cutoffs for space weather applications. J. Geophys. Res. (Space Phys. 120, 5694–5702. doi:10.1002/2014JA020899
Krimigis, S. M., Decker, R. B., Roelof, E. C., Hill, M. E., Armstrong, T. P., Gloeckler, G., et al. (2013). Search for the exit: voyager 1 at heliosphere’s border with the galaxy. Science 341, 144–147. doi:10.1126/science.1235721
Krimigis, S. M., Decker, R. B., Roelof, E. C., Hill, M. E., Bostrom, C. O., Dialynas, K., et al. (2019). Energetic charged particle measurements from Voyager 2 at the heliopause and beyond. Nat. Astron. 3, 997–1006. doi:10.1038/s41550-019-0927-4
Krimigis, S. M., Roelof, E. C., Decker, R. B., and Hill, M. E. (2011). Zero outward flow velocity for plasma in a heliosheath transition layer. Nature 474, 359–361. doi:10.1038/nature10115
Kudela, K., and Bucik, R. (2013). Low energy cosmic rays and the disturbed magnetosphere. arXiv e-prints , arXiv:1303.4052. doi:10.48550/arXiv.1303.4052
Kurth, W. S., and Gurnett, D. A. (2020). Observations of a radial density gradient in the very local interstellar medium by voyager 2. Astrophysical Journall 900, L1. doi:10.3847/2041-8213/abae58
Kusnierkiewicz, D. Y., Hersman, C. B., Guo, Y., Kubota, S., and McDevitt, J. (2005). A description of the pluto-bound New Horizons spacecraft. Acta Astronaut. 57, 135–144. doi:10.1016/j.actaastro.2005.03.030
Lallement, R., and Bertin, P. (1992). Northern-hemisphere observations of nearby interstellar gas: possible detection of the local cloud. Astronomy Astrophysics 266, 479–485.
Lallement, R., Quémerais, E., Bertaux, J. L., Ferron, S., Koutroumpa, D., and Pellinen, R. (2005). Deflection of the interstellar neutral hydrogen flow across the heliospheric interface. Science 307, 1447–1449. doi:10.1126/science.1107953
Lallement, R., Quémerais, E., Koutroumpa, D., Bertaux, J.-L., Ferron, S., Schmidt, W., et al. (2010). “The interstellar H flow: updated analysis of SOHO/SWAN data,” in Twelfth international solar wind conference. Editors M. Maksimovic, K. Issautier, N. Meyer-Vernet, M. Moncuquet, and F. Pantellini, 555–558. of American Institute of Physics Conference Series. doi:10.1063/1.3395925
Lallement, R., Raymond, J. C., Vallerga, J., Lemoine, M., Dalaudier, F., and Bertaux, J. L. (2004). Modeling the interstellar-interplanetary helium 58.4 nm resonance glow: towards a reconciliation with particle measurements. Astronomy Astrophysics 426, 875–884. doi:10.1051/0004-6361:20035929
Leonard, T. W., Möbius, E., Bzowski, M., Fuselier, S. A., Heirtzler, D., Kubiak, M. A., et al. (2015). Revisiting the ISN flow parameters, using a variable IBEX pointing strategy. Astrophysical J. 804, 42. doi:10.1088/0004-637X/804/1/42
Linsky, J., Redfield, S., Ryder, D., and Moebius, E. (2022). Inhomogeneity in the local ISM and its relation to the heliosphere. Space Sci. Rev. 218, 16. doi:10.1007/s11214-022-00884-5
Linsky, J. L., and Redfield, S. (2021). Could the local cavity be an irregularly shaped strömgren sphere? Astrophysical J. 920, 75. doi:10.3847/1538-4357/ac1feb
Linsky, J. L., Redfield, S., and Tilipman, D. (2019). The interface between the outer heliosphere and the inner local ISM: morphology of the local interstellar cloud, its hydrogen hole, strömgren shells, and 60Fe accretion. Astrophysical J. 886, 41. doi:10.3847/1538-4357/ab498a
Mall, U., Fichtner, H., Kirsch, E., Hamilton, D. C., and Rucinski, D. (1998). Cassini as a heliospheric probe - the potential of pick-up ion measurements during its cruise phase. Planet. Space Sci. 46, 1375–1382. doi:10.1016/S0032-0633(97)00193-1
McComas, D., Allegrini, F., Bagenal, F., Casey, P., Delamere, P., Demkee, D., et al. (2008). The solar wind around pluto (SWAP) instrument aboard New Horizons. Space Sci. Rev. 140, 261–313. doi:10.1007/s11214-007-9205-3
McComas, D. J., Alexashov, D., Bzowski, M., Fahr, H., Heerikhuisen, J., Izmodenov, V., et al. (2012a). The heliosphere’s interstellar interaction: No bow shock. Science 336, 1291–1293. doi:10.1126/science.1221054
McComas, D. J., Allegrini, F., Bochsler, P., Bzowski, M., Christian, E. R., Crew, G. B., et al. (2009). Global observations of the interstellar interaction from the interstellar boundary explorer (IBEX). Science 326, 959–962. doi:10.1126/science.1180906
McComas, D. J., Bzowski, M., Frisch, P., Fuselier, S. A., Kubiak, M. A., Kucharek, H., et al. (2015a). Warmer local interstellar medium: a possible resolution of the ulysses-IBEX enigma. Astrophysical J. 801, 28. doi:10.1088/0004-637X/801/1/28
McComas, D. J., Bzowski, M., Fuselier, S. A., Frisch, P. C., Galli, A., Izmodenov, V. V., et al. (2015b). Local interstellar medium: six years of direct sampling by IBEX. Astrophysical J. Suppl. 220, 22. doi:10.1088/0067-0049/220/2/22
McComas, D. J., Dayeh, M. A., Allegrini, F., Bzowski, M., DeMajistre, R., Fujiki, K., et al. (2012b). The first three years of IBEX observations and our evolving heliosphere. Astrophysical J. 203, 1. doi:10.1088/0067-0049/203/1/1
McComas, D. J., Dayeh, M. A., Funsten, H. O., Janzen, P. H., Schwadron, N. A., Szalay, J. R., et al. (2019). Expanding global features in the outer heliosphere. Astrophysical J. 872, 127. doi:10.3847/1538-4357/aafc2c
McComas, D. J., Dayeh, M. A., Funsten, H. O., Livadiotis, G., and Schwadron, N. A. (2013). The heliotail revealed by the interstellar boundary explorer. Astrophysical J. 771, 77. doi:10.1088/0004-637X/771/2/77
McComas, D. J., Schwadron, N. A., Crary, F. J., Elliott, H. A., Young, D. T., Gosling, J. T., et al. (2004). The interstellar hydrogen shadow: observations of interstellar pickup ions beyond Jupiter. J. Geophys. Res. (Space Phys. 109, A02104. doi:10.1029/2003JA010217
McComas, D. J., Zirnstein, E. J., Bzowski, M., Dayeh, M. A., Funsten, H. O., Fuselier, S. A., et al. (2017a). Seven years of imaging the global heliosphere with IBEX. Astrophysical J. Suppl. Ser. 229, 41. doi:10.3847/1538-4365/aa66d8
McComas, D. J., Zirnstein, E. J., Bzowski, M., Elliott, H. A., Randol, B., Schwadron, N. A., et al. (2017b). Interstellar Pickup Ion Observations to 38 au. Astrophysical J. Suppl. 233, 8. doi:10.3847/1538-4365/aa91d2
McNutt, R. L., Paul, M. V., Brandt, P. C., Kinnison, J. D., and Team, I. P. (2021). Interstellar probe: humanity’s journey to interstellar space, Laurel, MD: Johns Hopkins University Applied Physics Laboratory (JHU APL), Report no. nasa task order nnn06aa01c. Available at: https://interstellarprobe.jhuapl.edu/Interstellar-Probe-MCR.pdf.
Menten, K. M., Reid, M. J., Kamiński, T., and Claussen, M. J. (2012). The size, luminosity, and motion of the extreme carbon star IRC+10216 (CW Leonis). Astronomy Astrophysics 543, A73. doi:10.1051/0004-6361/201219422
Mewaldt, R. A., Davis, A. J., Lave, K. A., Leske, R. A., Stone, E. C., Wiedenbeck, M. E., et al. (2010). Record-setting cosmic-ray intensities in 2009 and 2010. Astrophysical J. Lett. 723, L1–L6. doi:10.1088/2041-8205/723/1/L1
Meyer, D. M., Lauroesch, J. T., Peek, J. E. G., and Heiles, C. (2012). The remarkable high pressure of the local leo cold cloud. Astrophysical J. 752, 119. doi:10.1088/0004-637X/752/2/119
Miller, J. A., and Fields, B. D. (2022). Heliospheric compression due to recent nearby supernova explosions. Astrophysical J. 934, 32. doi:10.3847/1538-4357/ac77f1
Möbius, E., Bochsler, P., Bzowski, M., Heirtzler, D., Kubiak, M. A., Kucharek, H., et al. (2012). Interstellar gas flow parameters derived from interstellar boundary explorer-lo observations in 2009 and 2010: analytical analysis. Astrophysical J. 198, 11. doi:10.1088/0067-0049/198/2/11
Möbius, E., Bzowski, M., Frisch, P. C., Fuselier, S. A., Heirtzler, D., Kubiak, M. A., et al. (2015a). Interstellar flow and temperature determination with IBEX: robustness and sensitivity to systematic effects. Astrophysical J. Suppl. 220, 24. doi:10.1088/0067-0049/220/2/24
Möbius, E., Hovestadt, D., Klecker, B., Scholer, M., Arbinger, H., Hoefner, H., et al. (1985a). The time-of-flight spectrometer SULEICA for ions of the energy range 5-270 keV/charge on AMPTE IRM. IEEE Trans. Geoscience Remote Sens. 23, 274–279. doi:10.1109/TGRS.1985.289527
Möbius, E., Hovestadt, D., Klecker, B., Scholer, M., Gloeckler, G., and Ipavich, F. M. (1985b). Direct observation of He+ pick-up ions of interstellar origin in the solar wind. Nature 318, 426–429. doi:10.1038/318426a0
Möbius, E., Lee, M. A., and Drews, C. (2015b). Interstellar flow longitude from the symmetry of the pickup ion cut-off at 1 AU. Astrophysical J. 815, 20. doi:10.1088/0004-637X/815/1/20
Möbius, E., Lee, M. A., Drews, C., and Gloeckler, G. (2016a). Interstellar flow direction from pickup ion cut-off dependence on longitude, flow and solar wind speed. In Solar Wind 14. vol. 1720 of Am. Inst. Phys. Conf. Ser., 090002. doi:10.1063/1.4943854
Möbius, E., Lee, M. A., Gloeckler, G., Drews, C., and Keilbach, D. (2016b). Interstellar flow longitude from pickup ion cut-off observations at 1 AU with STEREO and ACE. J. Phys. Conf. Ser. 767, 012017. doi:10.1088/1742-6596/767/1/012017
Moebius, E., Bochsler, P. A., Bzowski, M., Crew, G. B., Funsten, H. O., Fuselier, S. A., et al. (2009). “Direct observations of interstellar H, He, and O by the interstellar boundary explorer (invited),” in AGU fall meeting abstracts, 2009, SH31B–02.
Mrigakshi, A. I., Matthiä, D., Berger, T., Reitz, G., and Wimmer-Schweingruber, R. F. (2013). Estimation of Galactic Cosmic Ray exposure inside and outside the Earth’s magnetosphere during the recent solar minimum between solar cycles 23 and 24. Adv. Space Res. 52, 979–987. doi:10.1016/j.asr.2013.05.007
Müller, H. R., Frisch, P. C., Fields, B. D., and Zank, G. P. (2009). The heliosphere in time. Space Sci. Rev. 143, 415–425. doi:10.1007/s11214-008-9448-7
NASA (1971). The pioneer mission to jupiter. Available at: https://ntrs.nasa.gov/api/citations/19710022890/downloads/19710022890.pdf.
O’Callaghan, J. (2022). U.S. And Chinese scientists propose bold New missions beyond the solar system
Opher, M., Drake, J. F., Swisdak, M., Zieger, B., and Toth, G. (2017). The twist of the draped interstellar magnetic field ahead of the heliopause: a magnetic reconnection driven rotational discontinuity. Astrophysical J. Lett. 839, L12. doi:10.3847/2041-8213/aa692f
Opher, M., Drake, J. F., Zank, G., Powell, E., Shelley, W., Kornbleuth, M., et al. (2021). A turbulent heliosheath driven by the Rayleigh-taylor instability. Astrophysical J. 922, 181. doi:10.3847/1538-4357/ac2d2e
Opher, M., Drake, J. F., Zieger, B., and Gombosi, T. I. (2015). Magnetized jets driven by the Sun: the structure of the heliosphere revisited. Astrophysical J. Lett. 800, L28. doi:10.1088/2041-8205/800/2/L28
Opher, M., Kornbleuth, M., Decker, R., Richardson, J. D., et al. (2023). Flow anisotropies in the heliosheath. Astrophysical J. Letters submitted.
Opher, M., and Loeb, A. (2022). Terrestrial impact from the passage of the solar system through a cold cloud a few million years ago. Am. Astronomical Soc. Meet. Abstr. 54–06.
Opher, M., Loeb, A., Drake, J., and Toth, G. (2020). A small and round heliosphere suggested by magnetohydrodynamic modelling of pick-up ions. Nat. Astron. 4, 675–683. doi:10.1038/s41550-020-1036-0
Opher, M., Peek, J., and Loeb, A. (2022). Evidence that Earth was exposed to cold dense interstellar medium 2 Myrs ago as a result of the encounter with local Lynx cold clouds. “Preprint on webpage at. doi:10.21203/rs.3.rs-1855846/v1
Orlando, E., Bottacini, E., Moiseev, A. A., Bodaghee, A., Collmar, W., Ensslin, T., et al. (2022). Exploring the MeV sky with a combined coded mask and Compton telescope: the Galactic Explorer with a Coded aperture mask Compton telescope (GECCO). J. Cosmol. Astropart. Phys. 2022, 036. doi:10.1088/1475-7516/2022/07/036
Orlando, E., and Strong, A. (2021). StellarICS: inverse Compton emission from the quiet Sun and stars from keV to TeV. J. Cosmol. Astropart. Phys. 2021, 004. doi:10.1088/1475-7516/2021/04/004
Parker, E. N. (1958a). Dynamics of the interplanetary gas and magnetic fields. Astrophysical J. 128, 664. doi:10.1086/146579
Parker, E. N. (1958b). Suprathermal particle generation in the solar corona. Astrophysical J. 128, 677. doi:10.1086/146580
Pauls, H. L., and Zank, G. P. (1996). Interaction of a nonuniform solar wind with the local interstellar medium. J. Geophys. Res. 101, 17081–17092. doi:10.1029/96JA01298
Peri, C. S., Benaglia, P., and Isequilla, N. L. (2015). E-BOSS: an Extensive stellar BOw Shock Survey. II. Catalogue second release. Astronomy Astrophysics 578, A45. doi:10.1051/0004-6361/201424676
Pogorelov, N. V., Borovikov, S. N., Heerikhuisen, J., and Zhang, M. (2015). The heliotail. Astrophysical J. Lett. 812, L6. doi:10.1088/2041-8205/812/1/L6
Rando, R. (2017). The all-sky medium energy gamma-ray observatory. J. Instrum. 12, C11024. doi:10.1088/1748-0221/12/11/C11024
Redfield, S., and Linsky, J. L. (2008). The structure of the local interstellar medium. IV. Dynamics, morphology, physical properties, and implications of cloud-cloud interactions. Astrophysical J. 673, 283–314. doi:10.1086/524002
Reisenfeld, D. B., Bzowski, M., Funsten, H. O., Heerikhuisen, J., Janzen, P. H., Kubiak, M. A., et al. (2021). A three-dimensional map of the heliosphere from IBEX. Astrophysical J. Suppl. Ser. 254, 40. doi:10.3847/1538-4365/abf658
Richardson, J. D., and Decker, R. B. (2014). Voyager 2 observations of plasmas and flows out to 104 AU. Astrophysical J. 792, 126. doi:10.1088/0004-637X/792/2/126
Ripken, H. W., and Fahr, H. J. (1983). Modification of the local interstellar gas properties in the heliospheric interface. Astronomy Astrophysics 122, 181–192.
Sahai, R., and Chronopoulos, C. K. (2010). The astrosphere of the asymptotic giant branch star IRC+10216. Astrophysical J. Lett. 711, L53–L56. doi:10.1088/2041-8205/711/2/L53
Savage, B. D., and Mathis, J. S. (1979). Observed properties of interstellar dust. Annu. Rev. Astron Astrophysis 17, 73–111. doi:10.1146/annurev.aa.17.090179.000445
Schlei, W., Atchison, J., Gomez-Cano, R., Lathrop, B., and Villac, B. (2021). “Pragmatic trajectory options applicable to an interstellar probe mission,” in 2021 IEEE Aerospace Conference, 1–15. doi:10.1109/AERO50100.2021.9438398
Schwadron, N. A., Adams, F. C., Christian, E. R., Desiati, P., Frisch, P., Funsten, H. O., et al. (2014). Global anisotropies in TeV cosmic rays related to the Sun’s local galactic environment from IBEX. Science 343, 988–990. doi:10.1126/science.1245026
Schwadron, N. A., Möbius, E., Leonard, T., Fuselier, S. A., McComas, D. J., Heirtzler, D., et al. (2015). Determination of interstellar He parameters using five years of data from the IBEX: beyond closed form approximations. Astrophysical J. Suppl. 220, 25. doi:10.1088/0067-0049/220/2/25
Schwadron, N. A., Moebius, E., Kucharek, H., Lee, M. A., French, J., Saul, L., et al. (2013). Solar radiation pressure and local interstellar medium flow parameters from interstellar boundary explorer low energy hydrogen measurements. Astrophysical J. 775, 86. doi:10.1088/0004-637X/775/2/86
Slavin, J. D., and Frisch, P. C. (2008). The boundary conditions of the heliosphere: photoionization models constrained by interstellar and in situ data. Astronomy Astrophysics 491, 53–68. doi:10.1051/0004-6361:20078101
Spitzer, S. A. (2022). Characterizing heliospheric and interstellar interactions using pickup ion measurements. Ann Arbor, MI: Ph.d., Rackham Graduate School, University of Michigan. doi:10.7302/4723
Spitzer, S. A., Lepri, S. T., Raines, J. M., Gilbert, J. A., Moebius, E., Bower, J., et al. (2024). Determining the interstellar wind longitudinal inflow evolution using pickup ions in the helium focusing cone. Astrophys. J. in press
Stern, S. A. (2008). The New Horizons pluto kuiper belt mission: an overview with historical context. Space Sci. Rev. 140, 3–21. doi:10.1007/s11214-007-9295-y
Stone, E. C. (1977). The voyager missions to the outer system. Space Sci. Rev. 21, 75. doi:10.1007/BF00200845
Stone, E. C., Cummings, A. C., Heikkila, B. C., and Lal, N. (2019). Cosmic ray measurements from Voyager 2 as it crossed into interstellar space. Nat. Astron. 3, 1013–1018. doi:10.1038/s41550-019-0928-3
Stone, E. C., Cummings, A. C., McDonald, F. B., Heikkila, B. C., Lal, N., and Webber, W. R. (2005). Voyager 1 explores the termination shock region and the heliosheath beyond. Science 309, 2017–2020. doi:10.1126/science.1117684
Stone, E. C., Cummings, A. C., McDonald, F. B., Heikkila, B. C., Lal, N., and Webber, W. R. (2008). An asymmetric solar wind termination shock. Nature 454, 71–74. doi:10.1038/nature07022
Stone, E. C., Cummings, A. C., McDonald, F. B., Heikkila, B. C., Lal, N., and Webber, W. R. (2013). Voyager 1 observes low-energy galactic cosmic rays in a region depleted of heliospheric ions. Science 341, 150–153. doi:10.1126/science.1236408
Swaczyna, P., Bzowski, M., Kubiak, M. A., Sokół, J. M., Fuselier, S. A., Galli, A., et al. (2018). Interstellar neutral helium in the heliosphere from IBEX observations. V. Observations in IBEX-lo ESA steps 1, 2, and 3. Astrophysical J. 854, 119. doi:10.3847/1538-4357/aaabbf
Swaczyna, P., Kubiak, M. A., Bzowski, M., Bower, J., Fuselier, S. A., Galli, A., et al. (2022). Very local interstellar medium revealed by a complete solar cycle of interstellar neutral helium observations with IBEX. Astrophysical J. Suppl. 259, 42. doi:10.3847/1538-4365/ac4bde
Swaczyna, P., McComas, D. J., Zirnstein, E. J., Sokół, J. M., Elliott, H. A., Bzowski, M., et al. (2020). Density of neutral hydrogen in the Sun’s interstellar neighborhood. Astrophysical J. 903, 48. doi:10.3847/1538-4357/abb80a
Taut, A., Berger, L., Möbius, E., Drews, C., Heidrich-Meisner, V., Keilbach, D., et al. (2018). Challenges in the determination of the interstellar flow longitude from the pickup ion cutoff. Astronomy Astrophysics 611, A61–A12. doi:10.1051/0004-6361/201731796
Thomas, G. E., and Krassa, R. F. (1971). OGO 5 measurements of the lyman alpha sky background. Astronomy Astrophysics 11, 218.
Turner, D. L., Michael, A., Provornikova, E., Kornbleuth, M., Opher, M., Eriksson, S., et al. (2024). Evidence of a thick heliopause boundary layer resulting from active magnetic reconnection with the interstellar medium. Astrophysical J 960 (2), 130. doi:10.3847/1538-4357/ad05d3
Vallerga, J., Lallement, R., Lemoine, M., Dalaudier, F., and McMullin, D. (2004). EUVE observations of the helium glow: interstellar and solar parameters. Astronomy Astrophysics 426, 855–865. doi:10.1051/0004-6361:20035887
Vasyliunas, V. M., and Siscoe, G. L. (1976). On the flux and the energy spectrum of interstellar ions in the solar system. J. Geophys. Res. 81, 1247–1252. doi:10.1029/JA081i007p01247
Vincent, F. E., Ben-Jaffel, L., and Harris, W. M. (2012). “What does the interplanetary hydrogen tell us about the heliospheric interface,” in SF2A-2012: Proceedings of the Annual meeting of the French Society of Astronomy and Astrophysics. Editors S. Boissier, P. de Laverny, N. Nardetto, R. Samadi, D. Valls-Gabaud, and H. Wozniak, 53–58.
Wallner, A., Feige, J., Kinoshita, N., Paul, M., Fifield, L. K., Golser, R., et al. (2016). Recent near-Earth supernovae probed by global deposition of interstellar radioactive 60Fe. Nature 532, 69–72. doi:10.1038/nature17196
Weekes, T. C., Badran, H., Biller, S. D., Bond, I., Bradbury, S., Buckley, J., et al. (2002). VERITAS: the very energetic radiation imaging telescope array system. Astropart. Phys. 17, 221–243. doi:10.1016/S0927-6505(01)00152-9
Weller, C. S., and Meier, R. R. (1974). Observations of helium in the interplanetary/interstellar wind: the solar-wake effect. Astrophysical J. 193, 471–476. doi:10.1086/153182
Witte, M. (2004). Kinetic parameters of interstellar neutral helium. Review of results obtained during one solar cycle with the Ulysses/GAS-instrument. Astronomy Astrophysics 426, 835–844. doi:10.1051/0004-6361:20035956
Witte, M., Rosenbauer, H., Banaszkiewicz, M., and Fahr, H. (1993). The Ulysses neutral gas experiment: determination of the velocity and temperature of the interstellar neutral helium. Adv. Space Res. 13, 121–130. doi:10.1016/0273-1177(93)90401-V
Witte, M., Rosenbauer, H., Keppler, E., Fahr, H., Hemmerich, P., Lauche, H., et al. (1992). The interstellar neutral-gas experiment on ULYSSES. Astronomy Astrophysics, Suppl. 92, 333–348.
Wood, B. E., Müller, H.-R., and Witte, M. (2015). Revisiting Ulysses observations of interstellar helium. Astrophysical J. 801, 62. doi:10.1088/0004-637X/801/1/62
Wood, B. E., Müller, H. R., Zank, G. P., Izmodenov, V. V., and Linsky, J. L. (2004). The heliospheric hydrogen wall and astrospheres. Adv. Space Res. 34, 66–73. doi:10.1016/j.asr.2003.01.035
Xu, L., Li, H., Pei, Z., Zou, Y., and Wang, C. (2022). A brief introduction to the international lunar research station program and the interstellar express mission. Chin. J. Space Sci. 42, 511. doi:10.11728/cjss2022.04.yg28
Zank, G. P., Heerikhuisen, J., Wood, B. E., Pogorelov, N. V., Zirnstein, E., and McComas, D. J. (2013). Heliospheric structure: the bow wave and the hydrogen wall. Astrophysical J. 763, 20. doi:10.1088/0004-637X/763/1/20
Zank, G. P., Sterken, V., Giacalone, J., Möbius, E., von Steiger, R., Stone, E. S., et al. (2022). The early history of heliospheric science and the spacecraft that made it possible. Space Sci. Rev. 218, 34. doi:10.1007/s11214-022-00900-8
Zirnstein, E. J., Funsten, H. O., Heerikhuisen, J., McComas, D. J., Schwadron, N. A., and Zank, G. P. (2016a). Geometry and characteristics of the heliosheath revealed in the first five years of interstellar boundary explorer observations. Astrophysical J. 826, 58. doi:10.3847/0004-637x/826/1/58
Zirnstein, E. J., Heerikhuisen, J., Funsten, H. O., Livadiotis, G., McComas, D. J., and Pogorelov, N. V. (2016b). Local interstellar magnetic field determined from the interstellar boundary explorer ribbon. Astrophysical J. Lett. 818, L18. doi:10.3847/2041-8205/818/1/L18
Zirnstein, E. J., Heerikhuisen, J., Funsten, H. O., Livadiotis, G., McComas, D. J., and Pogorelov, N. V. (2016c). Local interstellar magnetic field determined from the interstellar boundary explorer ribbon. Astrophysical J. Lett. 818, L18. doi:10.3847/2041-8205/818/1/l18
Keywords: Interstellar Probe, interstellar medium, shape of the heliosphere, heliotail, outer heliosphere, modeling, instrumentation
Citation: Spitzer SA, Kornbleuth MZ, Opher M, Gilbert JA, Raines JM and Lepri ST (2024) Complementary interstellar detections from the heliotail. Front. Astron. Space Sci. 10:1163519. doi: 10.3389/fspas.2023.1163519
Received: 10 February 2023; Accepted: 19 December 2023;
Published: 08 February 2024.
Edited by:
Alexa Jean Halford, National Aeronautics and Space Administration, United StatesReviewed by:
Hans Mueller, Dartmouth College, United StatesCopyright © 2024 Spitzer, Kornbleuth, Opher, Gilbert, Raines and Lepri. This is an open-access article distributed under the terms of the Creative Commons Attribution License (CC BY). The use, distribution or reproduction in other forums is permitted, provided the original author(s) and the copyright owner(s) are credited and that the original publication in this journal is cited, in accordance with accepted academic practice. No use, distribution or reproduction is permitted which does not comply with these terms.
*Correspondence: Sarah A. Spitzer, c2FyYXlsZXRAdW1pY2guZWR1