- Space Research Institute, Russian Academy of Sciences, Moscow, Russia
Varying solar wind plays a crucial role in the processes inside the magnetosphere. Statistical studies generally reveal the geoeffectiveness of solar wind streams of different origins and types, characterized by various parameters such as dynamic pressure and magnetic field orientation. However, the predictions of the space weather are still not completely reliable. Small-scale structures (observed as high-amplitude variations with frequencies above 0.01 Hz) involved in the turbulent flow of the solar wind and in the magnetosheath may contribute to the models’ inaccuracies. Spacecraft measurements obtained during last 2 decades provide a great amount of new information about small-scale plasma processes in near-Earth space. However, the influence of solar wind on the dynamics of the small-scale structures in the magnetosheath has been rarely addressed. The present review summarizes experimental studies on this influence including features of turbulence around ion scales. The study aims to give a general picture of the problem and underline the gaps in current understanding of the role of the dynamics of the small-scale structures and turbulence in the solar–terrestrial relations.
1 Introduction
Interaction of the supersonic and super-Alfvenic solar wind (SW) flow with the Earth’s magnetosphere results in the formation of outstanding bow shock (BS) and a region behind it filled with turbulent shocked plasma—magnetosheath (MSH). The MSH plasma and magnetic field are in contact with the Earth’s magnetosphere. Therefore, the analysis of the MSH processes is of great importance for the exploration of solar–terrestrial relations and space weather forecasts.
Predictions of the magnetosphere response on the changing SW parameters are usually based on spacecraft measurements in the Lagrange L1 point (Yermolaev et al., 2010; Pallocchia et al., 2006; Boynton et al., 2012; Podladchikova and Petrukovich, 2012). These predictions do not account for alteration in plasma and magnetic field parameters in the course of passage from L1 to the magnetosphere, including substantial distortion of the flow in the foreshock region (Sibeck et al., 2008) and inside the MSH (Rakhmanova et al., 2015; 2016) and changes in the magnetic field orientation in front of the magnetopause (Šafránková et al., 2009; Pulinets et al., 2014). This approach provides high reliability only for intensive magnetosphere disturbances such as geomagnetic storms (Petrukovich et al., 2001). In addition to the statistical approach, models of the solar–terrestrial relations are based typically on MHD descriptions of plasma flow around the magnetosphere (Spreiter and Stahara, 1980; Kartalev et al., 1996; Toth et al., 2005). Though the MHD description is of great importance for global understanding of the MSH–magnetosphere system, it cannot reproduce high-amplitude small-scale variations (Zastenker et al., 2002; 2008; Hayosh et al., 2006). These variations are characterized by frequencies above 10−3–10–2 Hz and amplitudes above 5–10% and occur throughout the MSH (Němeček et al., 2000; Shevyrev et al., 2003; Lucek et al., 2005; Shevyrev and Zastenker, 2005; Gutynska et al., 2008; Gutynska et al., 2009; Gutynska et al., 2015). Moreover, typically, the description of the SW and MSH plasma and magnetic field is based on validity of the frozen-in hypothesis, which may be violated for low-speed plasma in the MSH (see Antonova et al., 2012 and references therein). Suggested mechanisms of plasma penetration through the magnetopause include finite gyroradius scattering, diffusion, wave-induced diffusion, impulsive penetration, and magnetic reconnection (Sibeck et al., 1999; Kirpichev et al., 2017; Fuselier, 2021). However, the presence of small-scale variations in the MSH favors additional ways for plasma transport across the magnetopause (Echim and Lemaire, 2002; Dmitriev and Suvorova, 2015); therefore, they can affect the magnetosphere processes. Observed discrepancies between models of the solar–terrestrial relations and reality may be a result of disregarding processes taking place at the scales around and below ion gyroradius (small-scale processes) in the MSH. Recent progress in technology made it possible to use hybrid models (Karimabadi et al., 2014; Omidi et al., 2014; Palmroth et al., 2018; Lu et al., 2020) which can successfully reproduce the basic properties of highly fluctuating MSH environments. Figure 1 presents the comparison of the MHD-modeled MSH (panel A, adopted from Toth et al., 2005) with results of three hybrid (electron fluid, kinetic ions) models (panels B–D). Small-scale structures are clearly captured by latter models, demonstrating the importance of their consideration. However, the stochastic nature of turbulent small-scale fluctuations makes them highly irreproducible both for distinct cases and monitoring of the MSH parameters. Therefore, in the exploration of mean characteristics of small-scale structures and turbulence, differences in its features for various background conditions may give information on when the existing forecasts would work well and when they should be corrected to account for processes in the MSH.
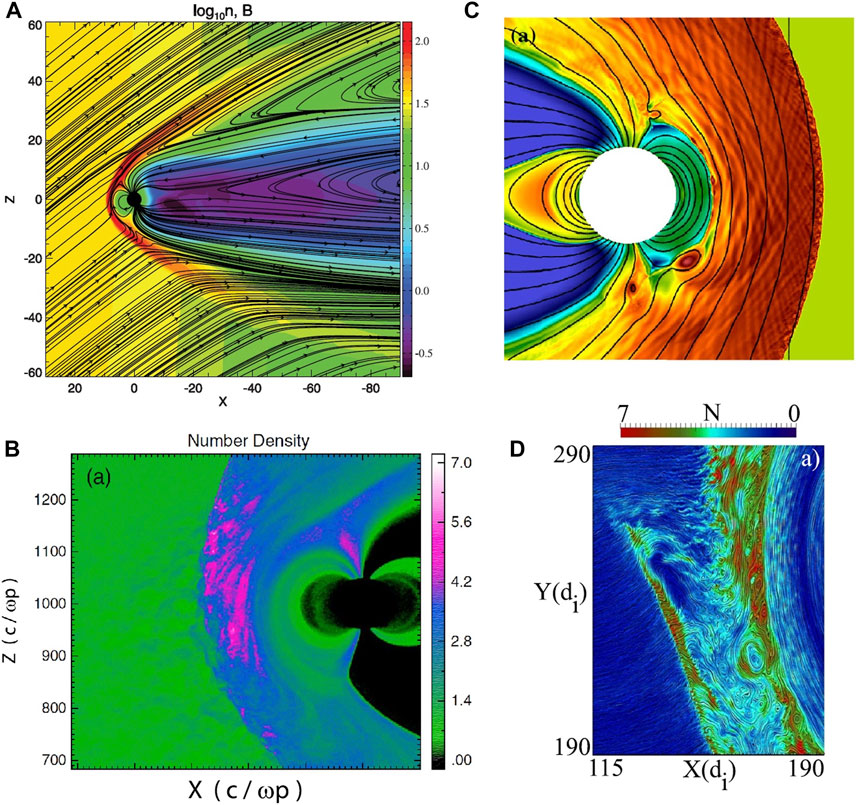
FIGURE 1. Magnetosheath density (shown by color) reproduced by (A) MHD model (adopted from Toth et al., 2005; figure 13), (B) 2.5-D electromagnetic hybrid simulations (adopted from Omidi et al., 2014; figure 10a), (C) hybrid Vlasov simulation (adopted from Palmroth et al., 2018; figure 7a (distributed under the terms of the Creative Commons Attribution 4.0 http://creativecommons.org/licenses/by/4.0/), and (D) 2D global hybrid simulation (the bow shock structure is shown, adopted from Karimabadi et al., 2014; figure 13a).
For a long time, MSH fluctuations have been considered in a framework of waves (Anderson et al., 1994; Hubert, 1994; Lacombe and Belmont, 1995; Schwartz et al., 1996). Great contributions have been made to the understanding of the MSH fluctuations (and are still in progress) with the help of Cluster mission measurements (Lucek et al., 2005). Recent measurements by MMS helped to better understand the small-scale fluctuations and reconnection in the turbulent MSH plasma (Stawarz et al., 2016; 2022; Vörös et al., 2019; Yordanova et al., 2020). A number of studies treated the MSH fluctuations in a framework of turbulence (see Alexandrova, 2008; Sahraoui et al., 2020; Rakhmanova et al., 2021 and references therein). Thus, there is a general understanding of sources, spectral features, and spatial scales of the fluctuations. However, the effect of the varying SW conditions on the properties of the MSH fluctuations was addressed quite rarely.
The present mini-review aims to give an overview of recent studies of the SW influence on the small-scale structures behind the BS. Here, the small-scale MSH structures/variations refer to variations of plasma and/or magnetic field parameters, which are registered by a spacecraft during seconds to minutes. The review does not discuss reconnection, current sheets, waves, and instabilities, however, as this topic is far too large and these have been extensively described before (Retinò et al., 2007; Yordanova et al., 2016; 2020; Khabarova et al., 2021 and references therein; Lacombe and Belmont, 1995; Schwartz et al., 1996). Only those studies have been mentioned here, which include observational analysis of the SW influence on the small-scale MSH structures. The authors believe that the review will help find gaps in the exploration of the space weather forecasts and motivate revision of available large datasets of high-quality MSH measurements from this point of view.
2 Basic MSH properties
MSH formation is determined by the interaction of the SW and the magnetosphere. The basic parameter which influences the power of variations behind the BS is the angle between the interplanetary magnetic field and local BS normal—θBN (Greenstadt, 1972; Shevyrev and Zastenker, 2005). For quasi-perpendicular BS (θBN > 45°), the low-energy SW ions (namely, protons and alfa particles, which are dominant in the SW) pass through the BS front and are advected away from it. Generally, the amplitude of the downstream small-scale fluctuations (with frequencies 0.01–1 Hz) is around 7–10% of the mean parameter value in this case (Shevyrev and Zastenker, 2005). For quasi-parallel BS (θBN < 45°), the SW ions are reflected from the front, go upstream (forming the foreshock region), and are simultaneously advected with the plasma flow to the downstream region. This complex path leads to powerful fluctuations behind the BS. The amplitude of the fluctuations behind quasi-parallel BS is typically twice as high as that behind the quasi-perpendicular BS and reaches 30% for almost-parallel BS (θBN < 10°) (Shevyrev and Zastenker 2005). Another well-known difference between the two configurations of the MSH lies in wave activity. At the quasi-perpendicular BS, significant temperature anisotropy T⊥>>T∥ arises with respect to the mean magnetic field vector. Temperature anisotropy leads to excitation of wave processes and instabilities, with their type depending on local plasma beta parameter (Lacombe and Belmont, 1995; Schwartz et al., 1996). Substantial differences in properties of the two configurations of the MSH imply that they should be considered separately to reveal the SW influence on the processes there. The foreshock region, populated with ions reflected from the BS, presents a complex, highly fluctuating, and anisotropic environment in front of the quasi-parallel BS (see Blanco-Cano et al., 2006; Sibeck et al., 2008; Gutynska et al., 2015 and references in these papers). Effects of the foreshock processes on the downstream MSH variations combine both foreshock intrinsic processes and pure SW effects. For this reason, foreshock processes are not focused on in the present review.
Another property of the MSH parameters is their dawn–dusk asymmetry which partly represents differences of the flow parameters behind the different BS types. A number of studies were focused on the problem of asymmetry in parameters’ values and MSH thickness (Němeček et al., 2000; Paularena et al., 2001; Němeček et al., 2003; Longmore et al., 2005; Dimmock and Nykyri, 2013). Generally, the dusk flank of the MSH is characterized by higher magnetic field magnitude, plasma speed, lower density, and ion temperature than the dawn flank (Dimmock et al., 2017). Most of the studies suggested SW speed and direction of the IMF to be the main parameters which affect the asymmetry.
3 Properties of the MSH fluctuations for changing SW conditions
A number of studies have tried to reveal if there was a connection between the MSH fluctuations properties and the SW parameters. Němeček et al. (2002) analyzed statistics of Interball-1 crossing of the MSH at 30-min intervals, which corresponded to fluctuations in the range ∼[0.001–0.01] Hz. The authors showed the fluctuation level weakly depends on the IMF Bz component and not on the SW Alfven Mach number MA and plasma β. However, a higher level of fluctuations was observed for decreasing the IMF cone angle, which could refer to the influence of the BS topology.
Gutynska et al. (2009) presented extensive statistics of magnetic field fluctuations in the frequency range [0.001–0.125] Hz measured by Cluster and analyzed the correlation properties of these fluctuations as a function of the SW parameters. Correlation length of the fluctuations (being 1 RE in average) was shown to increase significantly for increasing SW velocity, stayed unchanged for different SW densities, and was slightly higher for southward IMF compared to the northward IMF. Gutynska et al. (2015) analyzed properties of density transients (density increase by a factor of 1.5 and more during 10–60 s) in the dayside MSH and concluded that they tended to occur during high SW speeds and low densities. The authors also showed preferable occurrence of the structures during low IMF cone and θBN angles and concluded that density transients were often associated with foreshock processes.
Dimmock et al. (2014) studied the influence of the SW velocity and IMF direction on the distribution of the MSH magnetic field fluctuations in the frequency range [0.1, 2] Hz and their dawn–dusk asymmetry based on 6 years of THEMIS measurements. The authors concluded that fluctuations with higher amplitudes were observed for periods of fast SW (with velocities above 400 km/s). Additionally, amplified fluctuations in the vicinity of the magnetopause and absence of dawn–dusk asymmetry were shown for southward IMF. The authors used a magnetosheath interplanetary medium reference frame (Verigin et al., 2006) which allowed to account for aberration and for dynamics of the BS and magnetopause positions with variations of the SW parameters.
Rakhmanova et al. (2015), Rakhmanova et al. (2016), and Rakhmanova et al. (2018a) presented a statistical study of the two-point correlation function in the SW and MSH with the help of THEMIS data for density and magnetic field magnitude variations in the range [0.001–0.01] Hz. The authors suggested that the SW variations are more likely to stay unchanged during the BS crossing for higher SW densities and IMF magnitude. Additionally, SW variations with higher amplitudes were shown to be less modified in the MSH. No significant dependencies were found on the SW plasma β, IMF direction, or SW speed.
4 MSH jets and their dependence on the SW parameters
Presence of jets, usually described as transient enhancements of the dynamic pressure or density, is an important phenomenon in the magnetosheath as they can lead to local deformations in the magnetopause and plasma penetration (Lemaire, 1977; Lemaire and Roth, 1978; Dmitriev and Suvorova, 2015). There are several causes suggested for their formation including the BS ripples (Hietala et al., 2009; Hietala et al., 2012) and interaction of the SW discontinuities with the BS (Němeček et al., 1998; Savin et al., 2004; Savin et al. 2011; Savin et al. 2012; Dmitriev et al., 2021). A full overview of the phenomena can be found in Plaschke et al. (2018) and Dmitriev et al. (2021). Here, we highlight the results referring to the dependence of the jets on the SW parameters.
Archer and Horbury (2013) analyzed distribution of the high-amplitude transient (during the time intervals [10, 180] sec) enhancements of the dynamical pressure inside the MSH and their dependence on the SW conditions. The authors distinguished two basic regimes characterized by decrease or increase of density. The first group was typically observed during high-IMF cone angles, while the second group occurred predominantly for low-IMF cone angles. For the second regime (the most common), the enhancements were more frequent for increasing solar wind speed, while no dependencies were found on the IMF clock angle, SW plasma parameter β, or Mach number.
Plaschke et al. (2013) considered SW conditions favorable for jet formation in the subsolar MSH and concluded that the only significant factor was the IMF cone angle, which implied that the quasi-parallel BS was more favorable for jet formation. The authors used statistics of THEMIS measurements during 2008–2011, i.e., during solar minimum. Thus, the study included limited statistics of high values of the SW parameters such as ion density, speed, and IMF magnitude. Further analysis by LaMoury et al. (2021) considered enlarged statistics which covered the years 2008–2018 and showed additional SW parameters favorable for jet formation: SW speed around 300 km/s and 600 km/s, low IMF magnitude and dynamic pressure, high plasma β, and Alfven Mach number. The authors considered separately the SW conditions favorable for jets to reach the magnetopause if they were formed near the BS. These conditions included low-IMF cone angle and plasma β, high values of SW speed, IMF magnitude, and dynamic pressure. Thus, some of the SW properties (namely, the IMF magnitude) may contribute not only to the processes of jets’ formation but also to their dissipation in the MSH, and the relative portion of these effects are not clear so far.
The multiple SW factors, which may affect the magnetosphere and MSH, are not physically independent. Typically, their values are grouped according to the type of the SW, e.g., an origin of the SW stream at the Sun (Yermolaev et al., 2009; Borovsky et al., 2019). Generally, main types of the SW include slow or fast wind, interplanetary coronal mass ejections (ICMEs), and co-rotating interaction regions CIRs (or stream interaction regions, SIRs). In front of the ICMEs or CIRs, compressed regions may be formed (ICME Sheath or CIR Sheath); some of the catalogs of large-scale SW phenomena list them as a distinct SW type (Yermolaev et al., 2009). Different large-scale flows have varying geoeffectiveness (Borovsky and Denton, 2006; Yermolaev et al., 2012). Recently, the combination of the SW properties started to be considered as driving small-scale MSH processes as well. Koller et al. (2022) compared a list of the ICMEs and SIRs with the lists of the MSH jets and found clear differences in the jet’s occurrence rate for distinct large-scale SW types. For SIRs and high-speed streams, the number of jets was increased by up to 50% compared to the mean occurrence rate. On the other hand, the occurrence rate was decreased by 50% for ICMEs and associated Sheath regions. The authors noted that the study included only those jets which were aligned with the Sun–Earth line and are, therefore, geoeffective as they reached the magnetopause. This finding uncovers new information on geoeffectiveness of the SW events, as ICMEs and their Sheath regions are typically supposed to cause the most intense magnetospheric response.
5 Role of the large-scale SW streams in dynamics of the small-scale MSH structures
As mentioned in the previous section, large-scale SW structures have different effects on the small-scale MSH variations. However, typically their properties are considered as constant during the flow from the Lagrange L1 point to the magnetopause. At small scales, the plasma and magnetic field variations embedded in the large-scale structures could be modified across the BS and inside the MSH.
Turc et al. (2014) presented a study of simultaneous registration of the magnetic clouds (MCs and ICMEs with strong and regular magnetic field) in the MSH based on four representative cases. They have shown that behind the quasi-parallel BS, the smooth rotation of the magnetic field orientation in the MC may be dominated by the MSH variations, which results in difficulties in the magnetosphere response forecasts based on the SW data. Furthermore, Turc et al. (2017) presented a statistical study on simultaneous variations of the magnetic field in the MC upstream and downstream from the BS for 82 cases. The Bz component (which is believed to be one of the most geoeffective factors due to the processes of reconnection at the magnetopause) was shown to change its sign in the MSH, sometimes for periods as long as 30 min and more. These cases were observed for the IMF which lay in the equatorial plane. This result corresponds well with earlier results by Šafránková et al. (2009) who showed statistical differences between the sign of the Bz component in the SW and MSH. Turc et al. (2017) demonstrated that if the predominant IMF component was By, both positive and negative Bz could have equal geoeffectiveness.
Rakhmanova et al. (2018a) tried to connect the modification of the small-scale plasma structures in the MSH with the type of the upstream SW flow. Their results showed that during slow undisturbed SW, the small-scale SW structures stayed unchanged in the MSH just for 30% of cases. On the other hand, this percentage was higher by the factor of 2 during periods of the CIRs in the SW, that is, small-scale variations inside CIRs tend to survive across the BS. However, for other SW phenomena such as ICME ejecta, the small-scale variations tended to stay unmodified only for half of the cases.
Recently, Ala-Lahti et al. (2021) considered passage of the ICME Sheath through the BS. The authors compared magnetic field variations inside the Sheath, registered simultaneously in three points in the MSH, in the SW close to the BS, and at the Lagrange L1 point. The IMF Bz component was shown to be enhanced for a set of substructures, which made them more geoeffective. For some parts of the Sheath, the upstream small-scale structures survived during the transmission through the BS. On the other hand, for other periods, there was no correlation between upstream and downstream variations. The authors showed that variations with frequencies less than 0.01 Hz had increasing probability of surviving during passage through the BS.
Thus, the small-scale MSH structures may evolve in a different way for different large-scale SW types. Statistical relations between the SW parameters and magnetosphere responses often consider the SW type as a parameter. Therefore, an additional study of differences in its effect on the MSH small-scale structures would help improve predictability of the models.
6 SW effects on the features of the MSH turbulence and its evolution
Turbulence of the SW has been proposed to be one of the drivers of the magnetosphere dynamics (see D’Amicis et al., 2020 and references therein). Changes in the turbulence properties in the MSH for various SW conditions may be, thus, important for the magnetosphere response on the varying SW flow. Typically, around ion scales, the fluctuation spectra of the magnetic field and ion flux/density are characterized by two power laws with a break both in the SW and MSH; at frequencies lower than the break (MHD scales), the power law exponent (slope) is close to −5/3 (so-called Kolmogorov spectrum), while at higher frequencies (kinetic scales) the slope is around −2.8 (Alexandrova et al., 2013; Sahraoui et al., 2020; Rakhmanova et al., 2021). Statistical studies demonstrated modification of the shape of turbulent cascade around ion scales at the BS and its restoration at the flanks and near the magnetopause (Gutynska et al., 2009; Huang et al., 2017a; Rakhmanova et al., 2018b; Rakhmanova et al., 2018c). These statistical studies addressed the mean parameters and clearly demonstrated non-Kolmogorov scaling at MHD scales at the dayside MSH regions in the vicinity of the BS. However, several case studies (Rakhmanova et al., 2020a; Rakhmanova et al., 2020b) showed differences in the evolution of spectra between two points inside the MSH and different scenarios of the spectrum modification at the BS. The authors have suggested that turbulent cascade may evolve differently for specific SW conditions. A number of studies considered the influence of the SW parameters on the MSH turbulent spectra. Gutynska et al. (2009) showed changes of the mean spectral slopes at the MHD scales from −1.2 to −0.9 with increasing SW velocity. A statistical study (Rakhmanova et al., 2020c) showed a tendency to observe typical Kolmogorov scaling at the MHD scales for high-amplitude variations of the SW density and not to observe Kolmogorov scaling for high SW density values. Also, the authors showed that the spectral slope at the kinetic scales tended to deviate substantially from typical values and from theoretical predictions for northward IMF, while for southward IMF, the slope values were around −2.8, usually registered in the SW and MSH. The authors did not find any significant dependence on the SW velocity. On the other hand, Li et al. (2020) used MMS statistics and suggested no influence of the SW velocity and IMF Bz value on the evolution of the turbulence inside the MSH.
The preliminary statistical study of Rakhmanova et al. (2020b) demonstrated that changes in turbulent cascade at the BS may be influenced by the large-scale SW type. Recently, Rakhmanova et al. (2022) presented a set of multipoint case studies, which compared properties of the turbulent spectra in the SW, in the dayside MSH and at the MSH flank, taking into account the plasma propagation delays. The study included 12 cases from three groups of the SW stream types: slow undisturbed SW, ICMEs, and compressed SW (Sheath and CIRs). The results demonstrated that for undisturbed SW, the quasi-perpendicular BS affected the turbulent cascade in a confined region close to the BS, and the shape of the SW spectra restored at the flank MSH. On the other hand, interaction of the disturbed SW streams with the BS always resulted in violation of the Kolmogorov scaling at the MHD scales and in significant steepening of spectra at the kinetic scales in the whole dayside MSH. Interestingly, for the ICMEs, the spectra at the MSH flank tended to have properties similar to those registered in the SW, but for compressed SW flows, the properties of the cascade at the kinetic scales were not restored. This result is demonstrated in Figure 2, where slopes of the spectra in the dayside MSH and in the flank MSH at frequencies higher (P2) than the ion spectral break are shown versus the same slopes in the SW for three groups of the large-scale SW types. The panel on the left demonstrates linear dependence of the turbulence properties downstream from the BS in the dayside MSH on those in the upstream region for the three groups of the SW types. At the flank, the right panel exhibits substantial differences in development of kinetic-scale turbulence for compressed SW flows.
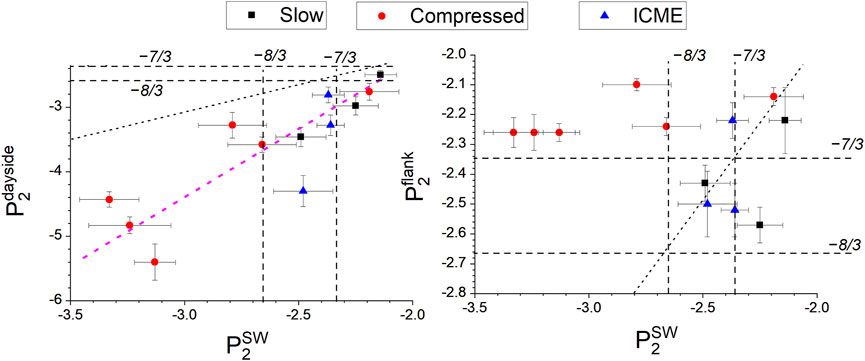
FIGURE 2. Spectral slopes at the frequencies higher than the ion spectral break in the dayside magnetosheath (left) and at the flank (right) versus same slopes upstream from the bow shock for three types of the solar wind; dotted lines show equal values of the downstream and upstream slopes, and magenta dashed line presents the linear fit (adopted from Rakhmanova et al., 2022; figure 6c, d, distributed under the terms of the Creative Commons Attribution 4.0 http://creativecommons.org/licenses/by/4.0/).
7 Summary
To date, few works were focused directly on the solar wind parameters, which influence the small-scale magnetosheath structures. The present mini-review underlines that
• Varying solar wind conditions contribute to the dynamics of the small-scale structures inside the magnetosheath; however, the contribution of this factor is much less examined than the influence of the foreshock, reconnection, and wave activity.
• IMF parameters (magnitude, Bz component, and cone angle) and ion bulk speed are most frequently reported solar wind parameters, which affect the dynamics of small-scale structures behind the bow shock.
• Recently, enlarged statistics of high-quality measurements in the magnetosheath helped to find additional factors which contributed to the dynamics of the small-scale structures in the magnetosheath. These factors were plasma density, ion parameter β, and Alfven Mach number.
• Ratio between the contribution of the aforementioned factors and their dependence on each other are still a matter of investigation; complex factors such as type of the solar wind flow may be more perspective for further exploration.
Solar wind parameters contribute to the solar–terrestrial relations not only determining the large-scale dynamics of the magnetosphere system, but through the changes in dynamics of small-scale MSH structures as well. A set of small-scale structures, found with the help of kinetic simulations and reported in the observations, was still not considered in the context of their relation to the upstream solar wind conditions. These structures include magnetic islands (Huang et al., 2017b), magnetic holes (Huang et al., 2016; Yao et al., 2021), plasmoids (Goncharov et al., 2020), and Alfven vortices (Alexandrova, 2008). A combination of different mission measurements during all parts of the solar cycle is required to get a full picture of properties of the small-scale MSH structures, their sources and contribution to the solar–terrestrial relations. To date, a set of missions with high-quality plasma and magnetic field measurements, complemented with realistic simulations, allows for such a complex exploration (Dimmock et al., 2020). The present review shows what has been achieved and what could be achieved in this scope for understanding the role of small-scale magnetosheath structures.
Author contributions
All authors listed have made a substantial, direct, and intellectual contribution to the work and approved it for publication. In particular, the paper was conceived and written by LR with the support and critical feedback of MR, GZ, and YY.
Funding
The study is supported by the Russian Ministry of Education by the theme “Plasma”.
Conflict of interest
The authors declare that the research was conducted in the absence of any commercial or financial relationships that could be construed as a potential conflict of interest.
Publisher’s note
All claims expressed in this article are solely those of the authors and do not necessarily represent those of their affiliated organizations, or those of the publisher, the editors, and the reviewers. Any product that may be evaluated in this article, or claim that may be made by its manufacturer, is not guaranteed or endorsed by the publisher.
References
Ala-Lahti, M., Dimmock, A. P., Pulkkinen, T. I., Good, S. W., Yordanova, E., Turc, L., et al. (2021). Transmission of an ICME sheath into the Earth's magnetosheath and the occurrence of traveling foreshocks. J. Geophys. Res. Space Phys. 126, e2021JA029896. doi:10.1029/2021JA029896
Alexandrova, O., Chen, C. H. K., Sorriso-Valvo, L., Horbury, T. S., and Bale, S. D. (2013). Solar wind turbulence and the role of ion instabilities. Space Sci. Rev. 178, 101–139. doi:10.1007/s11214-013-0004-8
Alexandrova, O. (2008). Solar wind vs magnetosheath turbulence and Alfvén vortices. Geophys 15, 95–108. doi:10.5194/npg-15-95-2008
Anderson, B. J., Fuselier, S. A., Gary, S. P., and Denton, R. E. (1994). Magnetic spectral signatures in the Earth’s magnetosheath and plasma depletion layer. J. Geophys. Res. 99, 5877–5891. doi:10.1029/93ja02827
Antonova, E. E., Pulinets, M. S., Riazantseva, M. O., Znatkova, S. S., Kirpichev, I. P., and Stepanova, M. V. (2012). in Turbulence in the magnetosheath and the problem of plasma penetration inside the magnetosphere Exploring the solar wind. Editor M. Lazar (Berlin, Germany: Springer), 417–438.
Archer, M. O., and Horbury, T. S. (2013). Magnetosheath dynamic pressure enhancements: Occurrence and typical properties. Ann. Geophys. 31 (2), 319–331. doi:10.5194/angeo-31-319-2013
Blanco-Cano, X., Omidi, N., and Russell, C. T. (2006). Macrostructure of collisionless bow shocks: 2. ULF waves in the foreshock and magnetosheath. J. Geophys. Res. 111, A10205. doi:10.1029/2005JA011421
Borovsky, J. E., and Denton, M. H. (2006). Differences between CME-driven storms and CIR-driven storms. J. Geophys. Res. 111, A07S08. doi:10.1029/2005ja011447
Borovsky, J. E., Denton, M. H., and Smith, C. W. (2019). Some properties of the solar wind turbulence at 1 AU statistically examined in the different types of solar wind plasma. J. Geophys. Res. Space Phys. 124, 2019JA026580–2424. doi:10.1029/2019JA026580
Boynton, R. J., Balikhin, M. A., Billings, S. A., Sharma, A. S., and Amariutei, O. A. (2012). Data derived NARMAX Dst model. Ann. Geophys. 29, 965–971. doi:10.5194/angeo-29-965-2011
D’Amicis, R., Telloni, D., and Bruno, R. (2020). The effect of solar-wind turbulence on magnetospheric activity. Front. Phys. 8, 604857. doi:10.3389/fphy.2020.604857
Dimmock, A. P., Hietala, H., and Zou, Y. (2020). Compiling magnetosheath statistical data sets under specific solar wind conditions: Lessons learnt from the dayside kinetic southward IMF GEM challenge. Earth Space Sci. 7, e2020EA001095. doi:10.1029/2020EA001095
Dimmock, A. P., Nykyri, K., Osmane, A., Karimabadi, H., and Pulkkinen, T. I. (2017). “Dawn-dusk asymmetries of the Earth's dayside magnetosheath in the magnetosheath interplanetary medium reference frame,” in Dawn-dusk asymmetries in planetary plasma environments (Washington, D.C., United States: American Geophysical Union), 49–72. doi:10.1002/9781119216346.ch5
Dimmock, A. P., Nykyri, K., and Pulkkinen, T. I. (2014). A statistical study of magnetic field fluctuations in the dayside magnetosheath and their dependence on upstream solar wind conditions. J. Geophys. Res. Space Phys. 119 (8), 6231–6248. doi:10.1002/2014JA020009
Dimmock, A. P., and Nykyri, K. (2013). The statistical mapping of magnetosheath plasma properties based on THEMIS measurements in the magnetosheath interplanetary medium reference frame. J. Geophys. Res. Space Phys. 118, 4963–4976. doi:10.1002/jgra.50465
Dmitriev, A. V., Lalchand, B., and Ghosh, S. (2021). Mechanisms and evolution of geoeffective large-scale plasma jets in the magnetosheath. Universe 7, 152. doi:10.3390/universe7050152
Dmitriev, A. V., and Suvorova, A. V. (2015). Large-scale jets in the magnetosheath and plasma penetration across the magnetopause: THEMIS observations. J. Geophys. Res. Sp. Phys. 120, 4423–4437. doi:10.1002/2014JA020953
Echim, M. M., and Lemaire, J. (2002). Positive density gradients at the magnetopause: Interpretation in the framework of the impulsive penetration mechanism. J. Atmos. Sol. Terr. Phys. 64, 2019–2028. doi:10.1016/s1364-6826(02)00229-8
Fuselier, S. A. (2021) Dayside magnetopause processes space Physics and aeronomy collection volume 2: Magnetospheres in the solar system, geophysical monograph 259. Editors Romain Maggiolo, Nicolas André, Hiroshi Hasegawa, and Daniel T. Welling First Edition (Netherland: John Wiley & Sons). doi:10.1002/9781119815624.ch10
Goncharov, O., Gunell, H., Hamrin, M., and Chong, S. (2020). Evolution of high-speed jets and plasmoids downstream of the quasi-perpendicular bow shock. J. Geophys. Res. Space Phys. 125, e2019JA027667. doi:10.1029/2019JA027667
Greenstadt, E. W. (1972). Binary index for assessing local bow shock obliquity. J. Geophys. Res. 77, 927 5467–5479. doi:10.1029/JA077i028p05467
Gutynska, O., Šafránková, J., and Němeček, Z. (2008). Correlation length of magnetosheath fluctuations: Cluster statistics. Ann. Geophys. 26, 2503–2513. doi:10.5194/angeo-26-2503-2008
Gutynska, O., Šafránková, J., and Němeček, Z. (2009). Correlation properties of magnetosheath magnetic field fluctuations. J. Geophys. Res. Sp. Phys. 114, A8. doi:10.1029/2009JA014173
Gutynska, O., Sibeck, D. G., and Omidi, N. (2015). Magnetosheath plasma structures and their relation to foreshock processes. J. Geophys. Res. Space Phys. 120, 7687–7697. doi:10.1002/2014JA020880
Hayosh, M., Šafránková, J., and Němeček, Z. (2006). MHD-modelling of the magnetosheath ion plasma flow and magnetic field and their comparison with experiments. Adv. Space Res. 37, 507–514. doi:10.1016/j.asr.2005.07.059
Hietala, H., Andréeová, K., Vainio, R., Vaivads, A., Palmroth, M., Rème, H., et al. (2009). Supermagnetosonic jets behind a collisionless quasiparallel shock. Phys. Rev. Lett. 103 (24), 245001–245023. doi:10.1103/PhysRevLett.103.245001
Hietala, H., Laitinen, T. V., Clausen, L. B., Facskò, G., Vaivads, A., Lucek, E. A., et al. (2012). Supermagnetosonic subsolar magnetosheath jets and their effects: From the solar wind to the ionospheric convection. Ann. Geophys. 30 (1), 33–48. doi:10.5194/angeo-30-33-2012
Huang, S. Y., Hadid, L. Z., Sahraoui, F., Yuan, Z. G., and Deng, X. H. (2017a). On the existence of the Kolmogorov inertial range in the terrestrial magnetosheath turbulence. Astrophys. J. Lett. 836, L10. doi:10.3847/2041-8213/836/1/L10
Huang, S. Y., Sahraoui, F., Retino, A., Le Contel, O., Yuan, Z. G., Chasapis, A., et al. (2016). MMS observations of ion-scale magnetic island in the magnetosheath turbulent plasma. Geophys. Res. Lett. 43, 7850–7858. doi:10.1002/2016GL070033
Huang, S. Y., Sahraoui, F., Yuan, Z. G., He, J. S., Zhao, J. S., et al. (2017b). A statistical study of kinetic-size magnetic holes in turbulent magnetosheath: MMS observations. J. Geophys. Res. Space Phys. 122, 8577–8588. doi:10.1002/2017JA024415
Hubert, D. (1994). Nature and origin of wave modes in the dayside Earth magnetosheath. Adv. Sp. 960 Res. 14, 55–64. doi:10.1016/0273-1177(94)90048-5
Karimabadi, H., Roytershteyn, V., Vu, H. X., Omelchenko, Y. A., Scudder, J., Daughton, W., et al. (2014). The link between shocks, turbulence, and magnetic reconnection in collisionless plasmas. Phys. Plasmas 21, 062308. doi:10.1063/1.4882875
Kartalev, M. D., Nikolova, V. I., Kamenetsky, V. F., and Mastikov, I. P. (1996). On the self-consistent determination of dayside magnetopause shape and position. Planet. Space Sci. 44, 1195–1208. doi:10.1016/S0032-0633(96)00040-2
Khabarova, O., Malandraki, O., Malova, H., Kislov, R., Greco, A., Bruno, R., et al. (2021). Current sheets, plasmoids and flux ropes in the heliosphere. Space Sci. Rev. 217, 38. doi:10.1007/s11214-021-00814-x
Kirpichev, I. P., Antonova, E. E., and Stepanova, M. (2017). Ion leakage at dayside magnetopause in case of high and low magnetic shears. J. Geophys. Res. Space Phys. 122, 8078–8095. doi:10.1002/2016JA023735
Koller, F., Temmer, M., Preisser, L., Plaschke, F., Geyer, P., Jian, L. K., et al. (2022). Magnetosheath jet occurrence rate in relation to CMEs and SIRs. J. Geophys. Res. Space Phys. 127, e2021JA030124. doi:10.1029/2021JA030124
Lacombe, C., and Belmont, G. (1995). Waves in the Earth's magnetosheath: Observations and interpretations. Adv. Sp. Res. 15, 329–340. doi:10.1016/0273-1177(94)00113-F
LaMoury, A. T., Hietala, H., Plaschke, F., Vuorinen, L., and Eastwood, J. P. (2021). Solar wind control of magnetosheath jet formation and propagation to the magnetopause. J. Geophys. Res. Space Phys. 126 (9), e2021JA029592. doi:10.1029/2021ja029592
Lemaire, J. (1977). Impulsive penetration of filamentary plasma elements into the magnetospheres of the Earth and Jupiter. Planet. Space Sci. 25, 887–890. doi:10.1016/0032-0633(77)90042-3
Lemaire, J., and Roth, M. (1978). Penetration of solar wind plasma elements into the magnetosphere. J. Atmos. Terr. Phys. 40, 331–335. doi:10.1016/0021-9169(78)90049-1
Li, H., Jiang, W., Wang, C., Verscharen, D., Zeng, C., Russell, C. T., et al. (2020). Evolution of the Earth’s magnetosheath turbulence: A statistical study based on MMS observations. Astrophys. J. 898, L43. doi:10.3847/2041-8213/aba531
Longmore, M., Schwartz, S. J., Geach, J., Cooling, B. M. A., Dandouras, I., Lucek, E. A., et al. (2005). Dawn-dusk asymmetries and sub-Alfvénic flow in the high and low latitude magnetosheath. Ann. Geophys. 23, 3351–3364. doi:10.5194/angeo-23-3351-2005
Lu, Q., Wang, H., Wang, X., Lu, S., Wang, R., Gao, X., et al. (2020). Turbulence-Driven magnetic reconnection in the magnetosheath downstream of a quasi-parallel shock: A three-dimensional global hybrid simulation. Geophys. Res. Lett. 47, e2019GL085661. doi:10.1029/2019gl085661
Lucek, E. A., Constantinescu, D., Goldstein, M. L., Pickett, J., Pinçon, J. L., Sahraoui, F., et al. (2005). The magnetosheath. Space Sci. Rev. 118, 95–152. doi:10.1007/s11214-005-3825-2
Němeček, Z., Hayosh, M., Šafránková, J., Zastenker, G. N., and Richardson, J. D. (2003). The dawn-dusk asymmetry of the magnetosheath: INTERBALL-1 observations. Adv. Space Res. 31, 1333–1340. doi:10.1016/S0273-1177(03)00007-3
Němeček, Z., Šafránková, J., Přech, L., Sibeck, D. G., Kokubun, S., and Mukai, T. (1998). Transient flux enhancements in the magnetosheath. Geophys. Res. Lett. 25, 1273–1276. doi:10.1029/98GL50873
Němeček, Z., Šafránková, J., Zastenker, G. N., Pišoft, P., and Jelínek, K. (2002). Low-frequency variations of the ion flux in the magnetosheath. Planet. Space Sci. 50, 567–575. doi:10.1016/s0032-0633(02)00036-3
Němeček, Z., Šafránková, J., Zastenker, G. N., Pišoft, P., Paularena, K. I., and Richardson, J. D. (2000). Observations of the radial magnetosheath profile and a comparison with gasdynamic model predictions. Geophys. Res. Lett. 27, 2801–2804. doi:10.1029/2000GL000063
Omidi, N., Sibeck, D., Gutynska, O., and Trattner, K. J. (2014). Magnetosheath filamentary structures formed by ion acceleration at the quasi-parallel bow shock. J. Geophys. Res. Space Phys. 119, 2593–2604. doi:10.1002/2013JA019587
Pallocchia, G., Amata, E., Consolini, G., Marcucci, M. F., and Bertello, I. (2006). Geomagnetic <i&gt;D&lt;sub&gt;st&lt;/sub&gt;</i&gt; index forecast based on IMF data only. Ann. Geophys. 24 (3), 989–999. doi:10.5194/angeo-24-989-2006
Palmroth, M., Ganse, U., Pfau-Kempf, Y., Battarbee, M., Turc, L., Brito, T., et al. (2018). Vlasov methods in space physics and astrophysics. Living Rev. comput. Astrophys. 4, 1. doi:10.1007/s41115-018-0003-2
Paularena, K. I., Richardson, J. D., Kolpak, M. A., Jackson, C. R., and Siscoe, G. L. (2001). A dawn-dusk density asymmetry in Earth’s magnetosheath. J. Geophys. Res. 106, 25377–25394. doi:10.1029/2000JA000177
Petrukovich, A. A., Lazarus, A., Lepping, R. P., and Klimov, S. I. (2001). Comparison of the solar wind energy input to the magnetosphere measured by Wind and Interball-1. J. Atmos. Sol. Terr. Phys. 63, 1643–1647. doi:10.1016/s1364-6826(01)00039-6
Plaschke, F., Hietala, H., and Angelopoulos, V. (2013). Anti-sunward high-speed jets in the subsolar magnetosheath. Ann. Geophys. 31 (10), 1877–1889. doi:10.5194/angeo-31-1877-2013
Plaschke, F., Hietala, H., Blanco-Cano, X., Kajdič, P., Karlsson, T., Sibeck, D., et al. (2018). Jets downstream of collisionless shocks. Space Sci. Rev. 214 (5), 81. doi:10.1007/s11214-018-0516-3
Podladchikova, T. V., and Petrukovich, A. A. (2012). Extended geomagnetic storm forecast ahead of available solar wind measurements. Space weather. 10, S07001. doi:10.1029/2012SW000786
Pulinets, M. S., Antonova, E. E., Riazantseva, M. O., Znatkova, S. S., and Kirpichev, I. P. (2014). Comparison of the magnetic field before the subsolar magnetopause with the magnetic field in the solar wind before the bow shock. Adv. Space Res. 54, 604–616. doi:10.1016/j.asr.2014.04.023
Rakhmanova, L., Riazantseva, M. O., Zastenker, G. N., Yermolaev, Y. I., Lodkina, I. G., and Chesalin, L. S. (2020a). Turbulent cascade in the magnetosheath affected by the solar wind’s plasma turbulence. Cosm. Res. 57, 443–450. doi:10.1134/S0010952519060066
Rakhmanova, L., Riazantseva, M., and Zastenker, G. (2016). Correlation level between solar wind and magnetosheath plasma and magnetic field parameters. Adv. Space Res. 58, 157–165. doi:10.1016/j.asr.2015.09.036
Rakhmanova, L., Riazantseva, M., and Zastenker, G. (2021). Plasma and magnetic field turbulence in the Earth’s magnetosheath at ion scales. Front. Astron. Space Sci. 7, 616635. doi:10.3389/fspas.2020.616635
Rakhmanova, L., Riazantseva, M., Zastenker, G., and Šafránková, J. (2015). Modification of small- and middle-scale solar wind structures by the bow shock and magnetosheath: Correlation analysis. Planet. Space Sci. 115, 12–18. doi:10.1016/j.pss.2015.03.003
Rakhmanova, L., Riazantseva, M., Zastenker, G., and Yermolaev, Y. (2022). Large-scale solar wind phenomena affecting the turbulent cascade evolution behind the quasi-perpendicular bow shock. Universe 8 (12), 611. doi:10.3390/universe8120611
Rakhmanova, L., Riazantseva, M., Zastenker, G., Yermolaev, Y., and Lodkina, I. (2020c). Dependence of the properties of a turbulent cascade behind the bow shock on the dynamics of the solar wind parameters. Cosm. Res. 58, 478–486. doi:10.1134/S0010952520060088
Rakhmanova, L., Riazantseva, M., Zastenker, G., Yermolaev, Y., and Lodkina, I. (2020b). Dynamics of plasma turbulence at Earth’s bow shock and through the magnetosheath. Astrophys. J. 901, 30. doi:10.3847/1538-4357/abae00
Rakhmanova, L. S., Riazantseva, M. O., Zastenker, G. N., and Verigin, M. I. (2018c). Effect of the magnetopause and bow shock on characteristics of plasma turbulence in the Earth’s magnetosheath. Geomagn. Aeron. 58, 718–727. doi:10.1134/S0016793218060129
Rakhmanova, L. S., Riazantseva, M. O., Zastenker, G. N., Verigin, M. I., Yermolaev, Yu. I., and Lodkina, I. G. (2018a). The influence of parameters of the interplanetary medium and magnetosheath boundaries on the correlation coefficient between the ion flux measured in the solar wind and the magnetosheath. Geomagn. Aeron. 58, 449–456. doi:10.1134/S0016793218040138
Rakhmanova, L. S., Riazantseva, M., Zastenker, G., and Verigin, M. (2018b). Kinetic-scale ion flux fluctuations behind the quasi-parallel and quasi-perpendicular bow shock. J. Geophys. Res. Sp. Phys. 123, 5300–5314. doi:10.1029/2018JA025179
Retinò, A., Sundkvist, D., Vaivads, A., Mozer, F., André, M., and Owen, C. J. (2007). In situ evidence of magnetic reconnection in turbulent plasma. Nat. Phys. 3, 235–238. doi:10.1038/nphys574
Šafránková, J., Hayosh, M., Gutynska, O., Němeček, Z., and Přech, L. (2009). Reliability of prediction of the magnetosheathB<i>Z</i>component from interplanetary magnetic field observations. J. Geophys. Res. Space Phys. 114. doi:10.1029/2009JA014552
Sahraoui, F., Hadid, L., and Huang, S. (2020). Magnetohydrodynamic and kinetic scale turbulence in the near-earth space plasmas: A (short) biased review. Rev. Mod. Plasma Phys. 4, 4–1132. doi:10.1007/s41614-020-0040-2
Savin, S., Amata, E., Zelenyi, L., Lutsenko, V., Safrankova, J., Nemecek, Z., et al. (2012). Super fast plasma streams as drivers of transient and anomalous magnetospheric dynamics. Ann. Geophys. 30, 1–7. doi:10.5194/angeo-30-1-2012
Savin, S., Budaev, V., Zelenyi, L., Amata, E., Sibeck, D., Lutsenko, V., et al. (2011). Anomalous interaction of a plasma flow with the boundary layers of a geomagnetic trap. JETP Lett. 93, 754–762. doi:10.1134/S0021364011120137
Savin, S. P., Zelenyi, L. M., Amata, E., Buechner, J., Blecki, J., Klimov, S. I., et al. (2004). Dynamic interaction of plasma flow with the hot boundary layer of a geomagnetic trap. Jetp Lett. 79, 368–371. doi:10.1134/1.1772433
Schwartz, S. J., Burgess, D., and Moses, J. J. (1996). Low-frequency waves in the Earth’s magnetosheath: Present status. Ann. Geophys. 14, 1134–1150. doi:10.1007/s00585-996-1134-z
Shevyrev, N. N., Zastenker, G. N., Nozdrachev, M. N., Němeček, Z., Šafránková, J., and Richardson, J. D. (2003). High and low frequency large amplitude variations of plasma and magnetic field in the magnetosheath: Radial profile and some features. Adv. Space Res. 31, 1389–1394. doi:10.1016/S0273-1177(03)00008-5
Shevyrev, N. N., and Zastenker, G. N. (2005). Some features of the plasma flow in the magnetosheath behind quasi-parallel and quasi-perpendicular bow shocks. Planet. Space Sci. 53, 95–102. doi:10.1016/j.pss.2004.09.033
Sibeck, D. G., Omidi, N., Dandouras, I., and Lucek, E. (2008). On the edge of the foreshock: Model-data comparisons. Ann. Geophys. 26, 1539–1544. doi:10.5194/angeo-26-1539-2008
Sibeck, D. G., Paschmann, G., Treumann, R. A., Fuselier, S. A., Lennartsson, W., Lockwood, M., et al. (1999). Plasma transfer processes at the magnetopause. Space Sci. Rev. 88, 207–283. doi:10.1023/A:1005255801425
Spreiter, J. R., and Stahara, S. S. (1980). A new predictive model for determining solar wind-terrestrial planet interactions. J. Geophys. Res. 85, 6769–6777. doi:10.1029/JA085iA12p06769
Stawarz, J. E., Eastwood, J. P., Phan, T. D., Gingell, I. L., Pyakurel, P. S., Shay, M. A., et al. (2022). Turbulence-driven magnetic reconnection and the magnetic correlation length: Observations from Magnetospheric Multiscale in Earth's magnetosheath. Phys. Plasmas 29, 012302. doi:10.1063/5.0071106
Stawarz, J. E., Eriksson, S., Wilder, F. D., Ergun, R. E., Schwartz, S. J., Pouquet, A., et al. (2016). 1177 observations of turbulence in a kelvin-helmholtz event on 8 september 2015 by the magnetospheric 1178 multiscale mission. J. Geophys. Res. Sp. Phys. 121 (11), 021–111. doi:10.1002/2016JA023458
Tóth, G., Sokolov, I. V., Gombosi, T. I., Chesney, D. R., Clauer, C. R., De Zeeuw, D. L., et al. (2005). Space weather modeling framework: A new tool for the space science community. J. Geophys. Res. Space Phys. 110, A12226. doi:10.1029/2005JA011126
Turc, L., Fontaine, D., Escoubet, C. P., Kilpua, E. K. J., and Dimmock, A. P. (2017). Statistical study of the alteration of the magnetic structure of magnetic clouds in the Earth's magnetosheath. J. Geophys. Res. Space Phys. 122 (3), 2956–2972. doi:10.1002/2016JA023654
Turc, L., Fontaine, D., Savoini, P., and Kilpua, E. K. J. (2014). Magnetic clouds’ structure in the magnetosheath as observed by Cluster and Geotail: Four case studies. Ann. Geophys. 32 (10), 1247–1261. doi:10.5194/angeo-32-1247-2014
Verigin, M. I., Tátrallyay, M., Erdös, G., and Kotova, G. A. (2006). Magnetosheath interplanetary medium reference frame: Application for a statistical study of mirror type waves in the terrestrial plasma environment. Adv. Space Res. 37, 515–521. doi:10.1016/j.asr.2005.03.042
Vörös, Z., Yordanova, E., Graham, D. B., Khotyaintsev, Y. V., and Narita, Y. (2019). MMS observations of whistler and lower hybrid drift waves associated with magnetic reconnection in the turbulent magnetosheath. J. Geophys. Res. Space Phys. 124, 8551–8563. doi:10.1029/2019ja027028
Yao, S. T., Yue, Z. S., Shi, Q. Q., Degeling, A. W., Fu, H. S., Tian, A. M., et al. (2021). Statistical properties of kinetic-scale magnetic holes in terrestrial space. Earth Planet. Phys. 5 (1), 63–72. doi:10.26464/epp2021011
Yermolaev, Y. I., Nikolaeva, N. S., Lodkina, I. G., and Yermolaev, M. Y. (2009). Catalog of large-scale solar wind phenomena during 1976-2000. Cosm. Res. 47, 81–94. doi:10.1134/S0010952509020014
Yermolaev, Y. I., Nikolaeva, N. S., Lodkina, I. G., and Yermolaev, M. Y. (2012). Geoeffectiveness and efficiency of CIR, Sheath and ICME in generation of magnetic storms. J. Geophys. Res. 117. doi:10.1029/2011JA017139
Yermolaev, Yu. I., Nikolaeva, N. S., Lodkina, I. G., and Yermolaev, M. Yu. (2010). Specific interplanetary conditions for CIR-Sheath-and ICME-induced geomagnetic storms obtained by double superposed epoch analysis. Ann. Geophys. 28, 2177–2186. doi:10.5194/angeo-28-2177-2010
Yordanova, E., Vörös, Z., Raptis, S., and Karlsson, T. (2020). Current sheet statistics in the 1209 magnetosheath. Front. Astron. Sp. Sci. 7, 2. doi:10.3389/fspas.2020.00002
Yordanova, E., Vörös, Z., Varsani, A., Graham, D. B., Norgren, C., Khotyaintsev, Y. V., et al. (2016). Electron scale structures and magnetic reconnection signatures in the turbulent magnetosheath. Geophys. Res. Lett. 43, 5969–5978. doi:10.1002/2016GL069191
Zastenker, G. N., Kartalev, M. D., Dobreva, P. S., Shevyrev, N. N., and Koval, A. (2008). A comparison of measured parameters of magnetosheath plasma with predictions of a new magnetosheath–magnetosphere model. Cosmic Res. 46, 469–483. doi:10.1134/S0010952508060014
Zastenker, G. N., Nozdrachev, M. N., Němeček, Z., Šafránková, J., Paularena, K. I., Richardson, J. D., et al. (2002). Multispacecraft measurements of plasma and magnetic field variations in the magnetosheath: Comparison with spreiter models and motion of the structures. Planet. Space Sci. 50, 601–612. doi:10.1016/S0032-0633(02)00039-9
Keywords: solar wind, bow shock, magnetosheath, Sun–Earth coupling, turbulence
Citation: Rakhmanova L, Riazantseva M, Zastenker G and Yermolaev Y (2023) Role of the variable solar wind in the dynamics of small-scale magnetosheath structures. Front. Astron. Space Sci. 10:1121230. doi: 10.3389/fspas.2023.1121230
Received: 11 December 2022; Accepted: 10 February 2023;
Published: 06 March 2023.
Edited by:
Olga V. Khabarova, Tel Aviv University, IsraelReviewed by:
Laxman Adhikari, University of Alabama in Huntsville, United StatesOlga Gutynska, Charles University, Czechia
Copyright © 2023 Rakhmanova, Riazantseva, Zastenker and Yermolaev. This is an open-access article distributed under the terms of the Creative Commons Attribution License (CC BY). The use, distribution or reproduction in other forums is permitted, provided the original author(s) and the copyright owner(s) are credited and that the original publication in this journal is cited, in accordance with accepted academic practice. No use, distribution or reproduction is permitted which does not comply with these terms.
*Correspondence: Liudmila Rakhmanova, cmFraGx1ZEBnbWFpbC5jb20=