- 1Heliophysics Science Division, NASA Goddard Space Flight Center, Greenbelt, MD, United States
- 2Department of Physics and Astronomy, George Mason University, Fairfax, VA, United States
- 3Physics Department, The Catholic University of America, Washington, DC, United States
- 4Department of Physics, American University, Washington, DC, United States
- 5Grupo de Estudios en Heliofísica de Mendoza, CONICET, Universidad de Mendoza, Mendoza, Argentina
- 6ADNET Systems, Inc, Greenbelt, MD, United States
- 7Space Sciences Laboratory, University of California–Berkeley, Berkeley, CA, United States
- 8Space Science Center, University of New Hampshire, Durham, NH, United States
- 9Universidad de Alcalá, Space Research Group, Alcalá de Henares, Madrid, Spain
- 10Goucher College, Center for Natural, Computer, and Data Sciences, Baltimore, MD, United States
- 11Shell Global Solutions (United States) Inc, Houston, TX, United States
- 12Predictive Science Inc, San Diego, CA, United States
- 13NASA Postdoctoral Program Fellow, NASA Goddard Space Flight Center, Greenbelt, MD, United States
- 14Universitat Politècnica de Catalunya, Barcelona, Spain
Magnetic flux ropes manifest as twisted bundles of magnetic field lines. They carry significant amounts of solar mass in the heliosphere. This paper underlines the need to advance our understanding of the fundamental physics of heliospheric flux ropes and provides the motivation to significantly improve the status quo of flux rope research through novel and requisite approaches. It briefly discusses the current understanding of flux rope formation and evolution, and summarizes the strategies that have been undertaken to understand the dynamics of heliospheric structures. The challenges and recommendations put forward to address them are expected to broaden the in-depth knowledge of our nearest star, its dynamics, and its role in its region of influence, the heliosphere.
1 Introduction
This paper addresses the need to investigate the fundamental solar and heliospheric magnetic structures known as flux ropes (FRs). FRs are commonly associated with coronal mass ejections (CMEs, Webb and Howard, 2012), streamer blow-outs (SBOs, Vourlidas and Webb, 2018; Nitta et al., 2021), density blobs generated due to magnetic reconnection at the tip of helmet streamers within the heliospheric plasma sheet (HPS, Lavraud et al., 2020; Réville et al., 2022), small structures called “plasmoids” or “blobs” observed in 2D by heliospheric imagers (e.g., Khabarova et al., 2021; Pezzi et al., 2021), solar flares (e.g., Kumar and Cho, 2013), small magnetic structures observed by in situ instrumentation (e.g., Moldwin et al., 2000; Cartwright and Moldwin, 2010a; Chen et al., 2020; Liu et al., 2021; Chen and Hu, 2022), as substructures of a larger structure (see for instance, Chen et al., 2023), and magnetospheric flux transfer events (FTEs, Russell and Elphic, 1978; Slavin et al., 2012; Murphy et al., 2020). FRs contribute greatly to the transport of energy, mass, and helicity from the Sun through the heliosphere and from the heliosphere to the planets’ local environments. They are characterized by an organized bundle of magnetic field lines, twisting around a common axis, confining plasma, and dragging away a large part of the Sun’s or a planet’s atmosphere (e.g., Linton and Moldwin, 2009). Considering the diversity of FRs described above, a question still remains: are all these structures alike in terms of morphology, magnetic and plasma properties, and dynamics?.
The FR concept was borrowed from the laboratory plasma physics experiments in the 1950–60s to confine and reach a stable plasma equilibrium to produce thermonuclear fusion power (e.g., Lundquist, 1950). Helical magnetic field structures were produced by induced toroidal current densities in laboratory devices, such as Tokamaks, to determine their stability. However, as the Heliophysics discipline has matured, the idealized FR concept (i.e., that of a circularly-symmetric, force-free, twisted flux tube) has become insufficient to accurately describe the structures, which are not always static or in equilibrium but ubiquitous in Heliophysics.
In this paper, we will discuss some of the issues that prevent us from advancing our understanding of the origin of these structures and the physical processes associated with their evolution. For example, the interpretation of remote-sensing and in situ observations often suggests complex distortions of FRs that are ambiguous and open to debate, and current models are not equipped to reproduce and simulate such complexities. In our opinion, the challenges that we present here range from data returned by space-based observatories to more theoretical approaches, but also encompass the development of more robust plasma physics laboratory experiments. On the basis of current challenges in FR research, we envision strategies and future venues to be addressed in the upcoming years.
2 Flux rope formation
Despite countless observations, both remote and in situ, that account for the existence of FRs, we have only a vague idea of their formation. Most models that are focused on CME eruption include a FR as an essential part of the process. However, there is a long-standing debate about whether these FRs exist in the corona before the eruption and later become unstable (ideal or magnetohydrodynamic instability, e.g., Török et al., 2004) or whether the FR forms as a consequence of the take-off of an unstable sheared arcade that triggers magnetic reconnection in its wake (resistive magnetohydrodynamic instability, e.g., Antiochos et al., 1999). The nature of the pre-eruptive configuration of solar eruptions has been extensively debated (see the reviews of Klimchuk, 2001; Forbes et al., 2006; Green et al., 2018; Patsourakos et al., 2020). Episodes of magnetic flux emergence can be regarded as the manifestation of twisted magnetic flux tubes rising through the solar surface, which result from the buoyant rise of magnetic plasma from the convection zone into the overlying atmosphere (e.g., Lites, 2009; Cheung and Isobe, 2014; Pontin and Priest, 2022). It is currently believed that the combination of photospheric plasma flows and magnetic reconnection above polarity inversion lines (see for instance, van Ballegooijen and Martens, 1989; Jiang et al., 2021) leading to FR formation, also during flux emergence, is the most common mechanism.
In light of observations of SBOs, it has been proposed that FRs can also be created later in the corona through reconnection processes (Lynch et al., 2016). The same mechanism seems to be responsible for the formation of small FRs or blobs and plasmoids (e.g., Sheeley et al., 2009; Sanchez-Diaz et al., 2017; Khabarova et al., 2021). Although there is supporting evidence in favor of each of the different aforementioned mechanisms, there are no conclusive findings, and this prevents us from fully understanding the formation mechanisms of different FRs.
The FRs originating further away from the Sun in the heliosphere mainly result from the solar wind’s evolution. This corresponds to magnetic reconnection in the heliospheric current sheet (HCS, e.g., Moldwin et al., 2000; Eastwood et al., 2002; Lavraud et al., 2020) and discontinuities produced by the action of turbulence in the solar wind (e.g., Zheng and Hu, 2018). Daughton et al. (2011) showed that for the most common type of reconnection layer with a finite guide field, the 3D evolution is dominated by the formation and interactions of FRs.
Several studies have correlated small FRs with interplanetary shock waves, particle energization, and stream interaction regions (SIRs, e.g., Feng et al., 2007; Cartwright and Moldwin, 2010b; Zank et al., 2014; le Roux et al., 2015). Thus, although the origin of large-scale FRs possesses well-defined observational signatures and unambiguously corresponds to CMEs and similar solar events, identification of the procedures involved in small-scale FR generation is still inconclusive.
In the ideal FR built in the laboratory, an axial current density induces the helical magnetic field topology. However, a non-idealized and more realistic heliospheric FR could be described by more complex internal current density distributions that, perhaps, impact the way the structure evolves. Therefore, does the formation mechanism determine the internal magnetic structure and impact the subsequent evolutionary processes?
3 Flux rope evolutionary processes
In the heliosphere, FRs are not static. They may continuously evolve through expansion, rotation, deflection, erosion, and distortion (e.g., Manchester et al., 2017; Kilpua et al., 2019; Luhmann et al., 2020). The physical processes associated with these effects are clearly related to the interaction with the local environment, but disentangling them is not an easy task. Most of the processes are coupled; for instance, the erosion with the distortion (Good et al., 2019; Nieves-Chinchilla et al., 2022a; Rodríguez-García et al., 2022), the expansion with the deflection (Nieves-Chinchilla et al., 2012, 2013), and they result in local significant changes within the global structures (Owens, 2020). Studies on the early evolution of FRs originating from the Sun estimate that the expansion and acceleration are probably due to the Lorentz force (e.g., Vršnak, 2008; Kay and Nieves-Chinchilla, 2021), but the range of influence of the different forces are not yet well defined.
In the interplanetary medium, the evolution of FRs is mostly dominated by interactions with the ambient solar wind. The magnetohydrodynamic (MHD) and/or aerodynamic drag affects FR kinematics and overall dynamics. It is also believed that with increasing heliocentric distance (e.g., Leitner et al., 2007; Gulisano et al., 2012) the FR radial expansion weakens, leading to FR deformations such as the “pancaking effect” (e.g., Cargill et al., 1996; Owens et al., 2006; Savani et al., 2010; Davies et al., 2021). However, the question of whether the global structure of FRs can be distorted or not is still open in the Heliophysics community. The interpretation of the remote-sensing and in situ observations that suggest complex distortions are ambiguous and open to debate (Owens, 2020). It is also important to highlight the importance of varied solar wind background structures that can distort longitudinally the coherent flux rope and significantly affect its local parameters probed at different places. Also, the interaction between structures can temporally change, even relatively quickly the FR properties (Kilpua et al., 2019) but, there are just a few physics-driven FR models flexible enough to advance such investigations (Hidalgo, 2003; Hidalgo and Nieves-Chinchilla, 2012; Nieves-Chinchilla et al., 2022a; Vršnak et al., 2004; 2008; 2013; Weiss et al., 2022).
The deflection or rotation effects are related to the change of the global orientation of a FR in the heliosphere, but their physical cause may be completely different. (e.g., Vourlidas et al., 2011; Nieves-Chinchilla et al., 2012). While the deflection is mostly driven by the force imbalance with the solar wind (Wang et al., 2004; Kay et al., 2017; Sahade et al., 2020), the rotation appears to be an internal magnetic instability (see for instance, Lynch et al., 2009; Florido-Llinas et al., 2020). Currently, running MHD simulations can be computationally expensive in time and resources and prevent us from testing different assumptions and conditions.
Finally, the erosion effect might significantly contribute to CME evolution. This well-known observed effect at the front, and sometimes also at the back, of in situ observations of FRs is due to the magnetic reconnection of the FR magnetic field with the ambient interplanetary magnetic field. This may impact the FR’s magnetic flux, twist, helicity, and cross-sectional area by “peeling off” its outer layers (Ruffenach et al., 2012; Pal et al., 2020, 2021; Pezzi et al., 2021; Pal et al., 2022a; Pal, 2022; Rodríguez-García et al., 2022). Magnetic reconnection is also associated with the internal changes of the FR, e.g., impacting the complexity of the in situ magnetic field profiles and/or the FR boundary layers (see, e.g., Feng et al., 2011; Hwang et al., 2020).
In this section, we have focused on the open challenges of large-scale FRs in the heliosphere associated with CMEs. However, all of these challenges can be extrapolated to other FRs in the heliosphere such as small-scale FRs or FTEs, for instance. In any case, we lack of a current effort to understand the physical characteristics of the FR internal structures, the changes as they evolve in the heliosphere, and the way the innate FR features connect to the matured structure’s features. Above all, there is a need to investigate how the temporal and spatial evolution impacts the stability, equilibrium, morphology, and entity of FRs.
4 The challenge of puzzling out flux ropes in the heliosphere
To study the FRs’ internal structure and evolution at any point of the heliosphere, it is customary to assemble observations from different assets in space, connect them with different models and data-analysis techniques, and elaborate on a scenario that reasonably describes their source region and the impact of the evolution on their structure. Figure 1 illustrates an exercise of connecting the remote and local in situ measurements of a FR at its source and in the inner heliosphere (see also Palmerio et al., 2018).
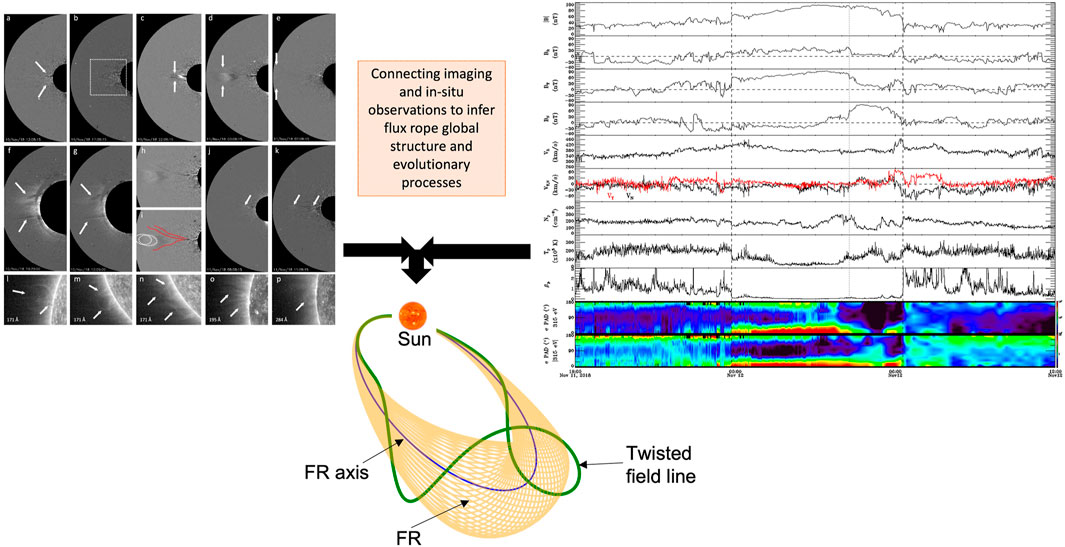
FIGURE 1. Example featuring the process of connecting the remote-sensing observations (from STEREO/EUVI and STEREO/COR2 and COR1) of a FR (left) and its local in-situ measurements (right) to infer the global internal structure and heliospheric evolution (middle). The two top images are reproduced from Nieves-Chinchilla et al. (2020) and the bottom cartoon is an adapted version of the Figure 4.5 in Carcaboso Morales (2021).
However, the unavailability of enough multi-point observations often misleads us in interpreting the global structure of FRs. We use different models and data-analysis techniques to bridge the gap resulting from the lack of observations with the caveat that these models can differ significantly from each other and can lead to different conclusions. For example, most models that use white-light observations (coronagraphs and heliospheric imagers) to study FR evolution fit static geometrical structures to match the morphology of a CME in simultaneous images (Thernisien et al., 2006; Rodríguez-García et al., 2022). These models do not include magnetic field information, and require multi-view points to (very often poorly) reproduce the 3D structure of the FR (see the discussion in Nieves-Chinchilla et al., 2022a). Furthermore, they do not provide thorough information about the evolutionary physical processes.
On the other hand, physical models that include magnetic field estimations (i.e., FR fitting models) are designed to match local in situ measurements and rely on, in the best scenarios, on single/few-point observations with relatively small spatial and varied temporal separations (e.g., Palmerio et al., 2021; Weiss et al., 2021; Pal et al., 2022b). Contemporary FR fitting approaches are not necessarily guaranteed to work well on larger scale separations (e.g., Weiss et al., 2021) as the simplifications in these models can break down. However, it is not well understood if by increasing the number of local FR measurement points, the FR reconstruction capabilities will improve unless the appropriate modeling techniques are developed in lockstep.
The aforementioned aspects prevent us from reaching a comprehensive understanding of FRs in the heliosphere. The ultimate challenge is to develop a model that is able to consistently respond to the wealth of observations and the evolution of these structures. From our perspective, to address this challenge, in addition to increasing space-based observations, the community should also make an effort to develop fundamental physics to explore the diversity of FRs in the heliosphere as well as to develop new techniques and approaches to further investigate their stability, dynamics, and interaction with the surrounding environment.
5 Proposed strategies
Here we summarize the challenges that result from the discussion in the previous sections and strategies to address those challenges. The goal of this perpective article is to raise awareness in the scientific community of the importance of magnetic FRs as a fundamental and ubiquitous magnetic structure in Heliophysics.
5.1 Challenges that have arisen from studies
The primary question that challenges our current understanding of FRs in the heliosphere is:
Are all flux ropes in the heliosphere alike in terms of morphology, magnetic and plasma properties, and dynamics?
To address this main issue, in the coming years, we, as a community, should aim to answer the following questions.
• Does the FR formation mechanism determine its internal magnetic structure and the impact of its subsequent evolution?
• How does the temporal and spatial evolution impact the stability, equilibrium, morphology, and entity of FRs?
• Can all FRs be understood via a single model?
5.2 Strategies to address the challenges
• Future Missions
As for any research in Space Physics, space assets tailored to solve specific problems are required. Here, we enumerate the most relevant instrumentation needed to tackle the pending fundamental questions regarding FRs. However, one of the pending tasks is to integrate the current observations into a single meta-data base. Thus, as the Heliophysics fleet of spacecraft grows, the upcoming observations can be seamlessly integrated.
Constellations of spacecraft should bring the opportunity to develop techniques and approaches to the problem from different perspectives. An example of this is the novel approach developed by Ayora Mexia. (2022) to evaluate the internal magnetic field current density distribution within FRs. Figure 2 illustrates the different spacecraft constellation formations to implement the curl-meter technique and to obtain the internal current density distribution within a FR.
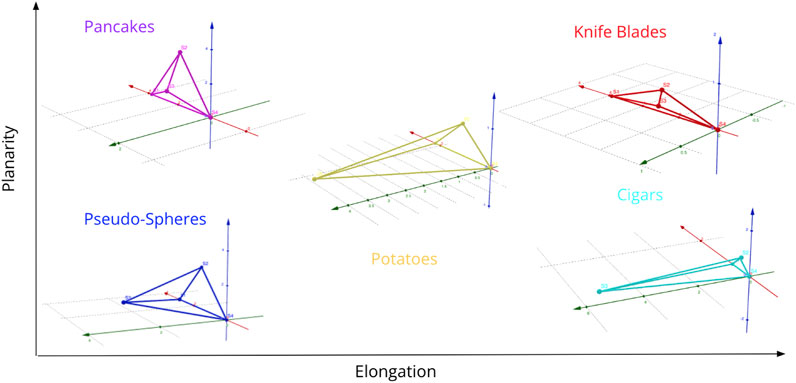
FIGURE 2. Exploring the efficiency of the curl-meter technique using five different types of tetrahedra as a function of elongation and planarity. According to Ayora Mexia. (2022), the pseudo-sphere is the best constellation formation to obtain the internal current density distribution within a FR.
In the case of the formation and early evolution of FRs, it is crucial to improve remote-sensing capabilities at low coronal heights. Upcoming new instrumentation filling the prevailing gap between 1.3 and 2.2 solar radii for uninterrupted coronal observations is of vital priority in this regard. Moreover, tracking and understanding the continuous evolution of solar FRs in the interplanetary medium as they propagate towards Earth, requires L4/L5 remote-sensing instrumentation with improved detection capabilities (e.g., Bemporad, 2021).
Multiple probing of FRs at different heliocentric distances and at different latitudes and longitudes may be used for classifying the large and small-scale FRs’ spatial and temporal behavior and their evolution, which in turn may lead us to uncover their origin. Multi-point observations will help in validating the model results meant for reconstructing complex FR structures and thereby leading to improvements in the models.
• Data Assimilation and Visualization
In order to decipher the internal structure and evolution of complex FRs, we need to enable the human mind to synthesize and make sense of the existing remote-sensing and in situ measurements by bringing clarity to how and where diverse observations connect. 1D, 2D, and multi-point observations from a variety of missions may all hold a piece of the story but are separated in space, time, and instrumental focus. As mentioned above, one of the pending tasks in the Heliophysics community is to integrate the current observations into a single meta-data base, enabling the focus on the scientific problem without the burden of the inter-calibration of instruments. Efforts in this direction have been made by the community, see for instance https://parker.gsfc.nasa.gov/icme_lists.html or http://fluxrope.info/. The first link attempts to provide a catalog of in situ CME events and reconstructions based on a circular-cylindrical (CC) and elliptical-cylindrical (EC) model (see Nieves-Chinchilla et al., 2016; Nieves-Chinchilla et al., 2018). The Second link systematically lists the small-scale FRs observed in situ by different missions using an automatic method based on the Grad-Shafranov reconstruction technique (see Hu and Sonnerup, 2002; Hu et al., 2018; Hu, 2021; Hu et al., 2022, for more information). The next step will be the development of visualization tools that will allow tackling the multidimensional problem and connecting with modeling in an integrated fashion. Working in this direction may be also connected with artificial intelligence techniques.
• Artificial Intelligence and Machine Learning
There has been a recent increase in machine learning applications in space weather, with the community identifying three key usages (Camporeale et al., 2018): 1) automatically identifying events/features that are traditionally time-consuming and error-prone via manual selection; 2) methods to study causality and cluster similar events with the aim of deepening our physical understanding; and 3) techniques to forecast space weather events from solar images, solar wind, and geospace in situ data. Because there are only sparse sets of measured data from within identified FRs, we should continue the work to leverage the combination of machine learning techniques with both measured data and synthetic data, from simulated FR models. Early results have shown a tantalizing glimpse of how this synergy of methods can inform our understanding of the structure and evolution of FRs, while also validating physics-based models. Using a convolutional neural network, dos Santos et al. (2020) created a binary classifier that learned to predict if a FR was or was not present in a given interval of solar wind data. Narock et al. (2022) subsequently used a related deep neural network to predict the orientation of the identified FRs. Nguyen et al. (2018) have explored machine learning techniques for automated identification of CMEs in situ, and Reiss et al. (2021) used machine learning to predict the minimum Bz value as a FR was sweeping past a spacecraft. This recent research demonstrates the potential for an integrated machine learning workflow to autonomously identify and classify FR events, alleviating much of the tedious and time-consuming manual component.
• Exploring New Flux Rope Models by Developing More Theory and Laboratory Research
Currently we lack a comprehensive understanding of realistic FR morphology and internal distribution of the plasma and magnetic field (see examples in Weiss et al., 2022). As we evolve in this knowledge, we need more physics-driven models, both numerical and analytical, to connect observations and understand the physical processes associated with FR interaction with the space environment. We recommend developing specific programs that support this goal, including long-term studies to develop FR models and fundamental investigations to analyze the effects of evolutionary processes from a theoretical perspective. We also recommend the coordination with laboratory plasma physics to test advances in a controlled laboratory environments (see e.g., Zweibel and Yamada, 2016; Gekelman et al., 2020)
As a final remark, we emphasize that improving our understanding of heliospheric FRs using technologies and modeling techniques would not only have an impact on fundamental physics understanding and on deep-space exploration, but also result in a significant societal benefit by enhancing the predictability of adverse space weather conditions.
Data availability statement
The original contributions presented in the study are included in the article, further inquiries can be directed to the corresponding author.
Author contributions
TNC was responsible for the organization and preparation of this article. All authors contributed and provided inputs on the manuscript. All authors revised the manuscript before submission. This paper is a version of the white paper submitted to the Heliophysics Decadal Survey (Nieves-Chinchilla et al. 2022a).
Funding
TN-C acknowledges the support of NASA Solar Orbiter, STEREO, PSP missions, and Heliophysics Internal Funds (HIF) programs. LRG acknowledges the financial support by the Spanish Ministerio de Ciencia, Innovación y Universidades FEDER/MCIU/AEI Projects ESP2017-88436-R and PID2019-104863RB-I00/AEI/10.13039/501100011033 and by the European Union’s Horizon 2020 research and innovation program under grant agreement No. 101004159 (SERPENTINE). RMW and EED acknowledge support from NASA grant 80NSSC19K0914 and partial support from the NASA STEREO grant 80NSSC20K0431. EP acknowledges NASA’s HTMS program (grant no. 80NSSC20K1274). HC is member of the “Carrera del Investigador Científico” of CONICET.
Conflict of interest
Authors AN and NA were employed by the company ADNET Systems, Inc. LD was employed by the company Shell Global Slutions (United States) Inc. EP was employed by the company Predictive Science Inc.
The remaining authors declare that the research was conducted in the absence of any commercial or financial relationships that could be construed as a potential conflict of interest.
Publisher’s note
All claims expressed in this article are solely those of the authors and do not necessarily represent those of their affiliated organizations, or those of the publisher, the editors and the reviewers. Any product that may be evaluated in this article, or claim that may be made by its manufacturer, is not guaranteed or endorsed by the publisher.
References
Antiochos, S. K., DeVore, C. R., and Klimchuk, J. A. (1999). A model for solar coronal mass ejections. Astrophys. J. 510, 485–493. doi:10.1086/306563
Ayora Mexia, M. (2022). Exploration of the internal flux rope current density using a spacecraft constellation. B.S. thesis. Barcelona, Spain: Universitat Politècnica de Catalunya.
Bemporad, A. (2021). Possible advantages of a twin spacecraft Heliospheric mission at the Sun-Earth Lagrangian points L4 and L5. Front. Astron. Space Sci. 8, 11. doi:10.3389/fspas.2021.627576
Camporeale, E., Wing, S., Johnson, J., Jackman, C. M., and McGranaghan, R. (2018). Space weather in the machine learning era: A multidisciplinary approach. Space weather. 16, 2–4. doi:10.1002/2017SW001775
Carcaboso Morales, F. (2021). Suprathermal and energetic electrons as probes of interplanetary magnetic field topology
Cargill, P. J., Chen, J., Spicer, D. S., and Zalesak, S. T. (1996). Magnetohydrodynamic simulations of the motion of magnetic flux tubes through a magnetized plasma. J. Geophys. Res. 101, 4855–4870. doi:10.1029/95JA03769
Cartwright, M. L., and Moldwin, M. B. (2010a). Heliospheric evolution of solar wind small-scale magnetic flux ropes. J. Geophys. Res. (Space Phys. 115, A08102. doi:10.1029/2009JA014271
Cartwright, M. L., and Moldwin, M. B. (2010b). Heliospheric evolution of solar wind small-scale magnetic flux ropes. J. Geophys. Res. 115, A08102. doi:10.1029/2009JA014271
Chen, Y., Hu, Q., Allen, R. C., and Jian, L. K. (2023). Small-scale magnetic flux ropes in stream interaction regions from parker solar probe and wind spacecraft observations. Astrophys. J. 943, 33. doi:10.3847/1538-4357/aca894
Chen, Y., and Hu, Q. (2022). Small-scale magnetic flux ropes and their properties based on in situ measurements from the parker solar probe. Astrophys. J. 924, 43. doi:10.3847/1538-4357/ac3487
Chen, Y., Hu, Q., Zhao, L., Kasper, J. C., Bale, S. D., Korreck, K. E., et al. (2020). Small-scale magnetic flux ropes in the first two parker solar probe encounters. Astrophys. J. 903, 76. doi:10.3847/1538-4357/abb820
Cheung, M. C. M., and Isobe, H. (2014). Flux emergence (theory). Living Rev. Sol. Phys. 11, 3. doi:10.12942/lrsp-2014-3
Daughton, W., Roytershteyn, V., Karimabadi, H., Yin, L., Albright, B. J., Bergen, B., et al. (2011). Role of electron physics in the development of turbulent magnetic reconnection in collisionless plasmas. Nat. Phys. 7, 539–542. doi:10.1038/nphys1965
Davies, E. E., Möstl, C., Owens, M. J., Weiss, A. J., Amerstorfer, T., Hinterreiter, J., et al. (2021). In situ multi-spacecraft and remote imaging observations of the first CME detected by Solar Orbiter and BepiColombo. Astron. Astrophys. 656, A2. doi:10.1051/0004-6361/202040113
dos Santos, L. F. G., Narock, A., Nieves-Chinchilla, T., Nuñez, M., and Kirk, M. (2020). Identifying flux rope signatures using a deep neural network. Sol. Phys. 295, 131. doi:10.1007/s11207-020-01697-x
Eastwood, J. P., Balogh, A., Dunlop, M. W., and Smith, C. W. (2002). Cluster observations of the heliospheric current sheet and an associated magnetic flux rope and comparisons with ACE. J. Geophys. Res. 107, 1365. doi:10.1029/2001JA009158
Feng, H. Q., Wu, D. J., and Chao, J. K. (2007). Size and energy distributions of interplanetary magnetic flux ropes. J. Geophys. Res. 112, A02102. doi:10.1029/2006JA011962
Feng, H. Q., Wu, D. J., Wang, J. M., and Chao, J. W. (2011). Magnetic reconnection exhausts at the boundaries of small interplanetary magnetic flux ropes. Astron. Astrophys. 527, A67. doi:10.1051/0004-6361/201014473
Florido-Llinas, M., Nieves-Chinchilla, T., and Linton, M. G. (2020). Analysis of the helical kink stability of differently twisted magnetic flux ropes. Sol. Phys. 295, 118. doi:10.1007/s11207-020-01687-z
Forbes, T. G., Linker, J. A., Chen, J., Cid, C., Kóta, J., Lee, M. A., et al. (2006). CME theory and models. Space Sci. Rev. 123, 251–302. doi:10.1007/s11214-006-9019-8
Gekelman, W., DeHaas, T., Prior, C., and Yeates, A. (2020). Using topology to locate the position where fully three-dimensional reconnection occurs. SNAS 2, 2187. doi:10.1007/s42452-020-03896-4
Good, S. W., Kilpua, E. K. J., LaMoury, A. T., Forsyth, R. J., Eastwood, J. P., and Möstl, C. (2019). Self-similarity of ICME flux ropes: Observations by radially aligned spacecraft in the inner heliosphere. J. Geophys. Res. (Space Phys. 124, 4960–4982. doi:10.1029/2019JA026475
Green, L. M., Török, T., Vršnak, B., Manchester, W., and Veronig, A. (2018). The origin, early evolution and predictability of solar eruptions. Space Sci. Rev. 214, 46. doi:10.1007/s11214-017-0462-5
Gulisano, A. M., Démoulin, P., Dasso, S., and Rodriguez, L. (2012). Expansion of magnetic clouds in the outer heliosphere. Astron. Astrophys. 543, A107. doi:10.1051/0004-6361/201118748
Hidalgo, M. A. (2003). A study of the expansion and distortion of the cross section of magnetic clouds in the interplanetary medium. J. Geophys. Res. 108, 1320. doi:10.1029/2002JA009818
Hidalgo, M. A., and Nieves-Chinchilla, T. (2012). A global magnetic topology model for magnetic clouds. I. Astrophys. J. 748, 109. doi:10.1088/0004-637X/748/2/109
Hu, Q. (2021). Optimal fitting of the freidberg solution to in situ spacecraft measurements of magnetic clouds. Sol. Phys. 296, 101. doi:10.1007/s11207-021-01843-z
Hu, Q., and Sonnerup, B. U. Ö. (2002). Reconstruction of magnetic clouds in the solar wind: Orientations and configurations. J. Geophys. Res. 107, 1142. doi:10.1029/2001JA000293
Hu, Q., Zheng, J., Chen, Y., le Roux, J., and Zhao, L. (2018). Automated detection of small-scale magnetic flux ropes in the solar wind: First results from the wind spacecraft measurements. Astrophys. J. Suppl. S. 239, 12. doi:10.3847/1538-4365/aae57d
Hu, Q., Zhu, C., He, W., Qiu, J., Jian, L. K., and Prasad, A. (2022). Validation and interpretation of a three-dimensional configuration of a magnetic cloud flux rope. Astrophys. J. 934, 50. doi:10.3847/1538-4357/ac7803
Hwang, K. J., Dokgo, K., Choi, E., Burch, J. L., Sibeck, D. G., Giles, B. L., et al. (2020). Magnetic reconnection inside a flux rope induced by kelvin-helmholtz vortices. J. Geophys. Res. (Space Phys. 125, e2019JA027665. doi:10.1029/2019JA027665
Jiang, C., Feng, X., Liu, R., Yan, X., Hu, Q., Moore, R. L., et al. (2021). A fundamental mechanism of solar eruption initiation. Nat. Astron. 5, 1126–1138. doi:10.1038/s41550-021-01414-z
Kay, C., Gopalswamy, N., Xie, H., and Yashiro, S. (2017). Deflection and rotation of CMEs from active region 11158. Sol. Phys. 292, 78. doi:10.1007/s11207-017-1098-z
Kay, C., and Nieves-Chinchilla, T. (2021). Modeling interplanetary expansion and deformation of cmes with anteatr parade: Relative contribution of different forces. J. Geophys. Res. Space Phys. 126, e2020JA028911. doi:10.1029/2020JA028911
Khabarova, O., Malandraki, O., Malova, H., Kislov, R., Greco, A., Bruno, R., et al. (2021). Current sheets, plasmoids and flux ropes in the heliosphere. Part I. 2-D or not 2-D? General and observational aspects. Space Sci. Rev. 217, 38. doi:10.1007/s11214-021-00814-x
Kilpua, E. K. J., Lugaz, N., Mays, M. L., and Temmer, M. (2019). Forecasting the structure and orientation of earthbound coronal mass ejections. Space weather. 17, 498–526. doi:10.1029/2018SW001944
Klimchuk, J. A. (2001). Theory of coronal mass ejections. Geophys. Monogr. 125, 143–157. doi:10.1029/GM125p0143
Kumar, P., and Cho, K.-S. (2013). Simultaneous EUV and radio observations of bidirectional plasmoids ejection during magnetic reconnection. Astron. Astrophys. 557, A115. doi:10.1051/0004-6361/201220999
Lavraud, B., Fargette, N., Réville, V., Szabo, A., Huang, J., Rouillard, A. P., et al. (2020). The heliospheric current sheet and plasma sheet during parker solar probe’s first orbit. Astrophys. J. Lett. 894, L19. doi:10.3847/2041-8213/ab8d2d
le Roux, J. A., Zank, G. P., Webb, G. M., and Khabarova, O. (2015). A kinetic transport theory for particle acceleration and transport in regions of multiple contracting and reconnecting inertial-scale flux ropes. Astrophys. J. 801, 112. doi:10.1088/0004-637X/801/2/112
Leitner, M., Farrugia, C. J., Möstl, C., Ogilvie, K. W., Galvin, A. B., Schwenn, R., et al. (2007). Consequences of the force-free model of magnetic clouds for their heliospheric evolution. J. Geophys. Res. 112, A06113. doi:10.1029/2006JA011940
Linton, M. G., and Moldwin, M. B. (2009). A comparison of the formation and evolution of magnetic flux ropes in solar coronal mass ejections and magnetotail plasmoids. J. Geophys. Res. 114, A00B09. doi:10.1029/2008JA013660
Lites, B. W. (2009). The topology and behavior of magnetic fields emerging at the solar photosphere. Space Sci. Rev. 144, 197–212. doi:10.1007/s11214-008-9437-x
Liu, Q., Zhao, Y., and Zhao, G. (2021). Statistical study of small-scale interplanetary magnetic flux ropes in the vicinity of the heliospheric current sheet. Front. Phys. 9, 637. doi:10.3389/fphy.2021.745152
Luhmann, J. G., Gopalswamy, N., Jian, L. K., and Lugaz, N. (2020). ICME evolution in the inner heliosphere. Sol. Phys. 295, 61. doi:10.1007/s11207-020-01624-0
Lynch, B. J., Antiochos, S. K., Li, Y., Luhmann, J. G., and DeVore, C. R. (2009). Rotation of coronal mass ejections during eruption. Astrophys. J. 697, 1918–1927. doi:10.1088/0004-637X/697/2/1918
Lynch, B. J., Masson, S., Li, Y., DeVore, C. R., Luhmann, J. G., Antiochos, S. K., et al. (2016). A model for stealth coronal mass ejections. J. Geophys. Res. Space Phys. 121, 10677–10697. doi:10.1002/2016JA023432
Manchester, W., Kilpua, E. K. J., Liu, Y. D., Lugaz, N., Riley, P., Török, T., et al. (2017). The physical processes of CME/ICME evolution. Space Sci. Rev. 212, 1159–1219. doi:10.1007/s11214-017-0394-0
Moldwin, M. B., Ford, S., Lepping, R., Slavin, J., and Szabo, A. (2000). Small-scale magnetic flux ropes in the solar wind. Geophys. Res. Lett. 27, 57–60. doi:10.1029/1999GL010724
Murphy, A. K., Winslow, R. M., Schwadron, N. A., Lugaz, N., Yu, W., Farrugia, C. J., et al. (2020). A survey of interplanetary small flux ropes at mercury. Astrophys. J. 894, 120. doi:10.3847/1538-4357/ab8821
Narock, T., Narock, A., Dos Santos, L. F. G., and Nieves-Chinchilla, T. (2022). Identification of flux rope orientation via neural networks. Front. Astron. Space Sci. 9, 838442. doi:10.3389/fspas.2022.838442
Nguyen, G., Fontaine, D., Aunai, N., Vandenbossche, J., Jeandet, A., Lemaitre, G., et al. (2018). “Machine learning methods to identify icmes automatically,” in 20th EGU General Assembly, EGU2018, Proceedings from the conference held, Vienna, Austria, 4-13 April, 2018, 1963.
Nieves-Chinchilla, T., Alzate, N., Cremades, H., Rodríguez-García, L., Dos Santos, L. F. G., Narock, A., et al. (2022a). Direct first parker solar probe observation of the interaction of two successive interplanetary coronal mass ejections in 2020 november. Astrophys. J. 930, 88. doi:10.3847/1538-4357/ac590b
Nieves-Chinchilla, T., Colaninno, R., Vourlidas, A., Szabo, A., Lepping, R. P., Boardsen, S. A., et al. (2012). Remote and in situ observations of an unusual Earth-directed coronal mass ejection from multiple viewpoints. J. Geophys. Res. 117, A06106. doi:10.1029/2011JA017243
Nieves-Chinchilla, T., Linton, M. G., Hidalgo, M. A., and Vourlidas, A. (2018). Elliptic-cylindrical analytical flux rope model for magnetic clouds. Astrophys. J. 861, 139. doi:10.3847/1538-4357/aac951
Nieves-Chinchilla, T., Linton, M. G., Hidalgo, M. A., Vourlidas, A., Savani, N. P., Szabo, A., et al. (2016). A circular-cylindrical flux-rope analytical model for magnetic clouds. Astrophysical J. 823, 27. doi:10.3847/0004-637x/823/1/27
Nieves-Chinchilla, T., Pal, S., Salman, T. M., Carcaboso, F., Guidoni, S. E., Cremades, H., et al. (2022b). What is a heliospheric flux rope? White paper submitted to the 2024–2033 Heliophysics decadal survey.
Nieves-Chinchilla, T., Szabo, A., Korreck, K. E., Alzate, N., Balmaceda, L. A., Lavraud, B., et al. (2020). Analysis of the internal structure of the streamer blowout observed by the parker solar probe during the first solar encounter. Astrophys. J. Suppl. S. 246, 63. doi:10.3847/1538-4365/ab61f5
Nieves-Chinchilla, T., Vourlidas, A., Stenborg, G., Savani, N. P., Koval, A., Szabo, A., et al. (2013). Inner heliospheric evolution of a “stealth” CME derived from multi-view imaging and multipoint in situ observations. I. Propagation to 1 AU. Astrophys. J. 779, 55. doi:10.1088/0004-637X/779/1/55
Nitta, N. V., Mulligan, T., Kilpua, E. K. J., Lynch, B. J., Mierla, M., O’Kane, J., et al. (2021). Understanding the origins of problem geomagnetic storms associated with “stealth” coronal mass ejections. Space Sci. Rev. 217, 82. doi:10.1007/s11214-021-00857-0
Owens, M. J. (2020). Coherence of coronal mass ejections in near-earth space. Sol. Phys. 295, 148. doi:10.1007/s11207-020-01721-0
Owens, M. J., Merkin, V. G., and Riley, P. (2006). A kinematically distorted flux rope model for magnetic clouds. J. Geophys. Res. Space Phys. 111, A03104. doi:10.1029/2005JA011460
Pal, S., Dash, S., and Nandy, D. (2020). Flux erosion of magnetic clouds by reconnection with the Sun’s open flux. Geophys. Res. Lett. 47, e2019GL086372. doi:10.1029/2019GL086372
Pal, S., Kilpua, E., Good, S., Pomoell, J., and Price, D. J. (2021). Uncovering erosion effects on magnetic flux rope twist. Astron. Astrophys. 650, A176. doi:10.1051/0004-6361/202040070
Pal, S., Lynch, B. J., Good, S. W., Palmerio, E., Asvestari, E., Pomoell, J., et al. (2022a). Eruption and interplanetary evolution of a stealthy streamer-blowout CME observed by PSP at ∼0.5 AU. Front. Astron. Space Sci. 9, 903676. doi:10.3389/fspas.2022.903676
Pal, S., Nandy, D., and Kilpua, E. K. J. (2022b). Magnetic cloud prediction model for forecasting space weather relevant properties of Earth-directed coronal mass ejections. Astron. Astrophys. 665, A110. doi:10.1051/0004-6361/202243513
Pal, S. (2022). Uncovering the process that transports magnetic helicity to coronal mass ejection flux ropes. Adv. Space Res. 70, 1601–1613. doi:10.1016/j.asr.2021.11.013
Palmerio, E., Kilpua, E. K. J., Möstl, C., Bothmer, V., James, A. W., Green, L. M., et al. (2018). Coronal magnetic structure of earthbound CMEs and in situ comparison. Space weather. 16, 442–460. doi:10.1002/2017SW001767
Palmerio, E., Nieves-Chinchilla, T., Kilpua, E. K. J., Barnes, D., Zhukov, A. N., Jian, L. K., et al. (2021). Magnetic structure and propagation of two interacting CMEs from the Sun to saturn. J. Geophys. Res. Space Phys. 126, e2021JA029770. doi:10.1029/2021JA029770
Patsourakos, S., Vourlidas, A., Török, T., Kliem, B., Antiochos, S. K., Archontis, V., et al. (2020). Decoding the pre-eruptive magnetic field configurations of coronal mass ejections. Space Sci. Rev. 216, 131. doi:10.1007/s11214-020-00757-9
Pezzi, O., Pecora, F., Le Roux, J., Engelbrecht, N. E., Greco, A., Servidio, S., et al. (2021). Current sheets, plasmoids and flux ropes in the heliosphere. Part II: Theoretical aspects. Space Sci. Rev. 217, 39. doi:10.1007/s11214-021-00799-7
Pontin, D. I., and Priest, E. R. (2022). Magnetic reconnection: MHD theory and modelling. Living Rev. Sol. Phys. 19, 1. doi:10.1007/s41116-022-00032-9
Reiss, M. A., Möstl, C., Bailey, R. L., Rüdisser, H. T., Amerstorfer, U. V., Amerstorfer, T., et al. (2021). Machine learning for predicting the Bz magnetic field component from upstream in situ observations of solar coronal mass ejections. Space weather. 19, e2021SW002859. doi:10.1029/2021SW002859
Réville, V., Fargette, N., Rouillard, A. P., Lavraud, B., Velli, M., Strugarek, A., et al. (2022). Flux rope and dynamics of the heliospheric current sheet. Study of the parker solar probe and solar orbiter conjunction of june 2020. Astron. Astrophys. 659, A110. doi:10.1051/0004-6361/202142381
Rodríguez-García, L., Nieves-Chinchilla, T., Gómez-Herrero, R., Zouganelis, I., Vourlidas, A., Balmaceda, L. A., et al. (2022). Evidence of a complex structure within the 2013 August 19 coronal mass ejection. Radial and longitudinal evolution in the inner heliosphere. Astron. Astrophys. 662, A45. doi:10.1051/0004-6361/202142966
Ruffenach, A., Lavraud, B., Owens, M. J., Sauvaud, J.-A., Savani, N. P., Rouillard, A. P., et al. (2012). Multispacecraft observation of magnetic cloud erosion by magnetic reconnection during propagation. J. Geophys. Res. 117, A09101. doi:10.1029/2012JA017624
Russell, C. T., and Elphic, R. C. (1978). Initial ISEE magnetometer results: Magnetopause observations. Space Sci. Rev. 22, 681–715. doi:10.1007/BF00212619
Sahade, A., Cécere, M., and Krause, G. (2020). Influence of coronal holes on CME deflections: Numerical study. Astrophys. J. 896, 53. doi:10.3847/1538-4357/ab8f25
Sanchez-Diaz, E., Rouillard, A. P., Davies, J. A., Lavraud, B., Sheeley, N. R., Pinto, R. F., et al. (2017). Observational evidence for the associated formation of blobs and raining inflows in the solar corona. Astrophys. J. Lett. 835, L7. doi:10.3847/2041-8213/835/1/L7
Savani, N. P., Owens, M. J., Rouillard, A. P., Forsyth, R. J., and Davies, J. A. (2010). Observational evidence of a coronal mass ejection distortion directly attributable to a structured solar wind. Astrophys. J. Lett. 714, L128–L132. doi:10.1088/2041-8205/714/1/L128
Sheeley, J., N. R., Lee, D. D. H., Casto, K. P., Wang, Y. M., and Rich, N. B. (2009). The structure of streamer blobs. Astrophys. J. 694, 1471–1480. doi:10.1088/0004-637X/694/2/1471
Slavin, J. A., Imber, S. M., Boardsen, S. A., DiBraccio, G. A., Sundberg, T., Sarantos, M., et al. (2012). MESSENGER observations of a flux-transfer-event shower at Mercury. J. Geophys. Res. 117, A00M06. doi:10.1029/2012JA017926
Thernisien, A. F. R., Howard, R. A., and Vourlidas, A. (2006). Modeling of flux rope coronal mass ejections. Astrophys. J. 652, 763–773. doi:10.1086/508254
Török, T., Kliem, B., and Titov, V. S. (2004). Ideal kink instability of a magnetic loop equilibrium. Astron. Astrophys. 413, L27–L30. doi:10.1051/0004-6361:20031691
van Ballegooijen, A. A., and Martens, P. C. H. (1989). formation and eruption of solar prominences. Astrophys. J. 343, 971. doi:10.1086/167766
Vourlidas, A., Colaninno, R., Nieves-Chinchilla, T., and Stenborg, G. (2011). The first observation of a rapidly rotating coronal mass ejection in the middle corona. Astrophys. J. Lett. 733, L23. doi:10.1088/2041-8205/733/2/L23
Vourlidas, A., and Webb, D. F. (2018). Streamer-blowout coronal mass ejections: Their properties and relation to the coronal magnetic field structure. Astrophys. J. 861, 103. doi:10.3847/1538-4357/aaca3e
Vršnak, B. (2008). Processes and mechanisms governing the initiation and propagation of CMEs. Ann. Geophys. 26, 3089–3101. doi:10.5194/angeo-26-3089-2008
Vršnak, B., Ruždjak, D., Sudar, D., and Gopalswamy, N. (2004). Kinematics of coronal mass ejections between 2 and 30 solar radii. What can be learned about forces governing the eruption? Astron. Astrophys. 423, 717–728. doi:10.1051/0004-6361:20047169
Vršnak, B., Vrbanec, D., and Čalogović, J. (2008). Dynamics of coronal mass ejections. The mass-scaling of the aerodynamic drag. Astron. Astrophys. 490, 811–815. doi:10.1051/0004-6361:200810215
Vršnak, B., Žic, T., Vrbanec, D., Temmer, M., Rollett, T., Möstl, C., et al. (2013). Propagation of interplanetary coronal mass ejections: The drag-based model. Sol. Phys. 285, 295–315. doi:10.1007/s11207-012-0035-4
Wang, Y., Shen, C., Liu, R., Liu, J., Guo, J., Li, X., et al. (2018). Understanding the twist distribution inside magnetic flux ropes by anatomizing an interplanetary magnetic cloud. J. Geophys. Res. Space Phys. 123, 3238–3261. doi:10.1002/2017JA024971
Wang, Y., Shen, C., Wang, S., and Ye, P. (2004). Deflection of coronal mass ejection in the interplanetary medium. Sol. Phys. 222, 329–343. doi:10.1023/b:sola.0000043576.21942.aa
Webb, D. F., and Howard, T. A. (2012). Coronal mass ejections: Observations. Living Rev. Sol. Phys. 9, 3. doi:10.12942/lrsp-2012-3
Weiss, A. J., Möstl, C., Davies, E. E., Amerstorfer, T., Bauer, M., Hinterreiter, J., et al. (2021). Multi-point analysis of coronal mass ejection flux ropes using combined data from Solar Orbiter, BepiColombo, and Wind. Astron. Astrophys. 656, A13. doi:10.1051/0004-6361/202140919
Weiss, A. J., Nieves-Chinchilla, T., Möstl, C., Reiss, M. A., Amerstorfer, T., and Bailey, R. L. (2022). Writhed analytical magnetic flux rope model. J. Geophys. Res. (Space Phys. 127, e2022JA030898. doi:10.1029/2022JA030898
Zank, G. P., le Roux, J. A., Webb, G. M., Dosch, A., and Khabarova, O. (2014). Particle acceleration via reconnection processes in the supersonic solar wind. Astrophys. J. 797, 28. doi:10.1088/0004-637X/797/1/28
Zheng, J., and Hu, Q. (2018). Observational evidence for self-generation of small-scale magnetic flux ropes from intermittent solar wind turbulence. Astrophys. J. Lett. 852, L23. doi:10.3847/2041-8213/aaa3d7
Keywords: sun, heliosphere, magnetic field, flux rope, coronal mass ejection, solar wind
Citation: Nieves-Chinchilla T, Pal S, Salman TM, Carcaboso F, Guidoni SE, Cremades H, Narock A, Balmaceda LA, Lynch BJ, Al-Haddad N, Rodríguez-García L, Narock TW, Dos Santos LFG, Regnault F, Kay C, Winslow RM, Palmerio E, Davies EE, Scolini C, Weiss AJ, Alzate N, Jeunon M and Pujadas R (2023) Redefining flux ropes in heliophysics. Front. Astron. Space Sci. 10:1114838. doi: 10.3389/fspas.2023.1114838
Received: 02 December 2022; Accepted: 15 February 2023;
Published: 08 March 2023.
Edited by:
Gian Luca Delzanno, Los Alamos National Laboratory (DOE), United StatesReviewed by:
Jiansen He, Peking University, ChinaOlga V. Khabarova, Tel Aviv University, Israel
Emilia Kilpua, University of Helsinki, Finland
Copyright © 2023 Nieves-Chinchilla, Pal, Salman, Carcaboso, Guidoni, Cremades, Narock, Balmaceda, Lynch, Al-Haddad, Rodríguez-García, Narock, Dos Santos, Regnault, Kay, Winslow, Palmerio, Davies, Scolini, Weiss, Alzate, Jeunon and Pujadas. This is an open-access article distributed under the terms of the Creative Commons Attribution License (CC BY). The use, distribution or reproduction in other forums is permitted, provided the original author(s) and the copyright owner(s) are credited and that the original publication in this journal is cited, in accordance with accepted academic practice. No use, distribution or reproduction is permitted which does not comply with these terms.
*Correspondence: Teresa Nieves-Chinchilla , dGVyZXNhLm5pZXZlc0BuYXNhLmdvdg==