- 1Space Science Endeavors, LLC, Silver Spring, MD, United States
- 2Goddard Planetary Heliophysics Institute, University of Maryland, Baltimore, MD, United States
- 3Department of Astronomy, Boston University, Boston, MA, United States
Recently it has been identified that our Moon had an extensive magnetosphere for several hundred million years soon after it was formed when the Moon was within 20 Earth Radii (RE) from the Earth. Some aspects of the interaction between the early Earth-Moon magnetospheres are investigated by mapping the interconnected field lines between the Earth and the Moon and investigating how the early lunar magnetosphere affects the magnetospheric dynamics within the coupled magnetospheres over time. So long as the magnetosphere of the Moon remains strong as it moves away from the Earth in the antialigned dipole configuration, the extent of the Earth’s open field lines decreases. As a result, at times it significantly changes the structure of the field-aligned current system, pushing the polar cusp significantly northward, and forcing magnetotail reconnection sites into the deeper tail region. In addition, the combined magnetospheres of the Earth and the Moon greatly extend the number of closed field lines enabling a much larger plasmasphere to exist and connecting the lunar polar cap with closed field lines to the Earth. That configuration supports the transfer of plasma between the Earth and the Moon potentially creating a time capsule of the evolution of volatiles with depth. This paper only touches on the evolution of the early Earth and Moon magnetospheres, which has been a largely neglected space physics problem and has great potential for complex follow-on studies using more advanced tools and due to the expected new lunar data coming in the next decade through the Artemis Program.
1 Introduction
The prevailing model for the generation of our Moon is the giant impact hypothesis in which a differentiated Mars-sized proto-planet impacted the proto-Earth during the early formation of the Solar System (Cameron and Benz, 1991; Canup and Asphaug, 2001), with the resulting debris forming the Earth and the Moon. The formation of the Moon begins just outside the Roche limit of ∼2.9 Earth radii (RE). Due to the dissipation of tidal energy, the Moon moved away from the Earth to its present position of 60 RE. Soon after formation, internal dynamos, producing magnetospheres, were generated in both the Earth and the Moon (Green et al., 2020).
Except for Venus, all Solar System planets are known to have generated magnetospheres during their evolutionary history. There is mounting evidence that our Moon supported asignificant global intrinsic magnetic field for several hundred million years soon after it was formed (Tikoo et al., 2017; Tikoo and Jung, 2023). Planetary dynamos and the associated magnetic fields evolve over time in many significant ways, such as the decrease in intensity as the convective core cools down and periodical polarity reversals. Our understanding of the physics inside magnetospheres has been dominated by studying the Earth’s magnetosphere as a single system whose variations are produced by varying solar wind conditions. The continued analysis of the lunar samples returned from Apollo 17 has enabled researchers to determine that the remanent magnetic field in the basalts is consistent with an axial symmetric dipole and that it existed for several hundred million years (Nichols et al., 2021). However, additional analysis of other Apollo impact glass and silicate crystals bearing magnetic inclusions by Tarduno et al. (2021) led those authors to the conclusion that if the Moon had an intrinsic magnetic field, it would be for an even shorter period. Samples returned from the lunar south pole by NASA’s Artemis program, where the intrinsic magnetic field is expected to be the strongest, should resolve the intensity over time debate, orientation, and periods of reversals.
Recently, Green et al. (2020) have investigated the expected magnetic topology of the early Earth-Moon magnetospheres and found that they would couple in such a way as to protect the atmosphere of both the Earth and the Moon, which also has implications for the detection and habitability of exomoons and exoplanets (Green et al., 2021). Using the technique developed by Green et al. (2020), we will extend this analysis to include a discussion of expected changes in magnetospheric dynamics. The configuration that will be discussed is shown in Figure 1, which provides an overview of the expected magnetic topology under the Earth-Moon antialigned dipole configuration during the time when the Moon moved away from the Earth at three epochs (Moon at 4, 8, and 18 RE). The magnetic fields of Earth and the Moon are approximated by dipoles with magnetic moments directed in the Z direction of −3 × 104 and 1 × 103 nT RE3, respectively, located along the aberration-corrected X-axis. The Sun is located along the +X-axis. The top three panels are when the Moon is on the nightside, and the bottom three panels illustrate magnetic topology when the Moon is on the dayside of the Earth. Given the significant limitations to the above approach (e.g., one dipole orientation), this paper only serves as a beginning of a new area of investigation for heliophysics researchers that may also be important for future exoplanet and exomoon research.
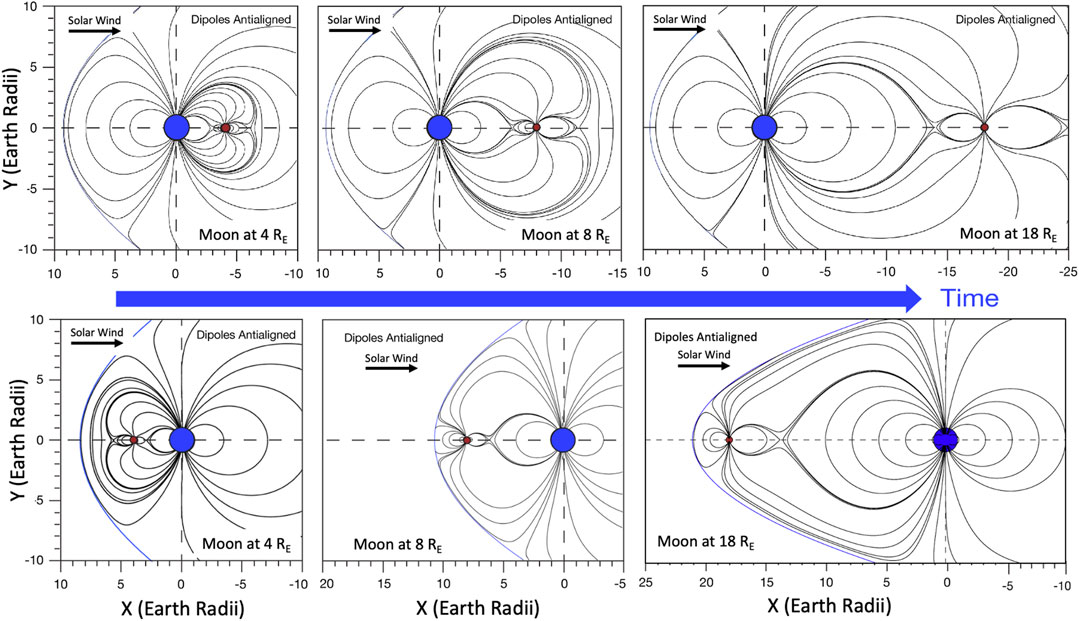
FIGURE 1. Overview of the changing magnetospheric topologies of the early Earth-Moon magnetosphere over about 800 million years at lunar locations of 4, 8, and 18 RE. The top three panels have the location of the lunar magnetosphere on the nightside, and the bottom three panels have the lunar magnetosphere on the dayside. All configurations are show with the Earth-Moon dipole field anitaligned.
2 Solar wind-magnetosphere interactions
Figure 1 qualitatively illustrates six Earth-Moon magnetic field configurations during the early evolution of these two bodies (4.4–3.7 billion years ago) while the Moon had a significant magnetic field. The chosen locations are only representative and are not meant to be exhaustive but provide some end member examples. In all these cases, the orbital period of the Moon is significantly larger than the time of a geomagnetic storm which typically lasts several hours. The orbital periods of the Moon at 4, 8, and 18 RE are 11.25, 31.75, and 107 h, respectively. This enables the assumption that the Earth and Moon are in static positions during a geomagnetic storm.
A typical geomagnetic storm begins with an Interplanetary Coronal Mass Ejection (ICME) hitting the Earth’s magnetosphere. As shown in the bottom three panels of Figure 1, the dayside lunar magnetosphere provides significant additional magnetic flux that must be considered. When the Moon is at 4 RE and its magnetosphere is well within the Earth’s magnetosphere (lower left panel, Figure 1), the interplanetary magnetic field (IMF) interacts with the distant magnetopause of Earth’s field lines in which reconnection (from anti-parallel field lines) takes place, putting the reconnected magnetic flux into the Earth’s magnetotail. During the process, energy builds up in the magnetotail until it cannot be sustained and is explosively released, accelerating particles toward the Earth’s upper atmosphere. This loading and unloading of energy into the terrestrial system is known as a substorm (Petrukovich et al., 2016). This situation changes if the IMF starts to interact with the lunar magnetic field lines for which its magnetic field configuration is in the opposite direction and thus the reconnection cannot occur. In a similar manner, when the Moon is beyond about 8 RE (middle and right lower panels of Figure 1), it will take the brunt of the ICME in this configuration. In the case when IMF is antiparallel with the lunar field, reconnection can occur between IMF and the lunar magnetic field, but it will stop once the field erosion reaches the Earth’s magnetic field. In general, the two antiparallel dipole magnetospheres work together to keep ICME erosion to only specific regions protecting the inner Earth’s magnetosphere and, therefore its atmosphere.
When the Moon is on the nightside (upper three panels of Figure 1), an ICME with IMF antiparallel to the Earth’s dayside field will reconnect at the magnetopause, taking the magnetic flux into the tail region and the lunar magnetosphere plays an important role in the magnetotail dynamics. One of the consequences is that the lunar magnetosphere pushes the Earth’s magnetotail reconnection sites to distant tail regions. Because the Earth’s nightside field lines either connect to the Moon or to the solar wind in the higher latitude polar cap, it is not clear how the acceleration of plasma in the newly established distant tail region will be placed on field lines that connect to the Earth. However, it is expected that a portion of the solar wind plasma, convecting across the Earth’s polar cap by E X B (cross polar cap electric field (E) crossed with the Earth’s magnetic field (B)), will migrate onto closed field lines between the Earth and the Moon. In this qualitative topology, the Earthward streaming accelerated plasma may reach the Earth’s new auroral zone, but its size and its intensity are significantly reduced. It is expected that the Moon, however, will receive the bulk of the auroral precipitation. It is important to note that during the time of the lunar magnetosphere, the Moon is believed to have an atmosphere with a surface pressure of about 10 mb (Needham and Kring, 2017), due to significant bombardment and a volcanically active Moon releasing volatiles trapped in the lunar crust all protected by its own magnetosphere. Therefore, the major portion of an auroral substorm will produce significant aurora in the tenuous lunar atmosphere which may also provide the plasma for closing the current system.
2.1 Topics for further study
Full three-dimensional magnetohydrodynamic (MHD) codes and kinetic plasma modeling would be required to refine the above qualitative analysis, which is outside the scope of this paper. Significant MHD modeling has been used to study solar wind-Earth’s magnetosphere interactions (e.g., Ngwira et al., 2004) and stellar wind-exoplanetary magnetosphere interactions (e.g., Dong et al., 2020), but not with the addition of a lunar magnetosphere under two dipole orientations whose intensity changes over time and with the Moon’s distance from the Earth. The modeling would determine how and when an expanded ring current encompassing the outer magnetospheric regions of both Earth and the Moon would be formed, the extent of the plasmaspheres, and the auroras on both bodies. In addition, as the lunar magnetosphere begins to emerge from the Earth’s magnetosphere it will develop a bow shock and magnetosheath and interact with the Earth’s and it should be modeled to potentially reveal new phenomena. Recent modeling of the solar wind plasma interactions with a weak lunar paleomagnetosphere (∼2.5 billion years old) has been accomplished with the Moon isolated in the solar wind (Poppe et al., 2021). These studies demonstrate that the tools to apply to the very early Earth-Moon magnetospheres are available. More detailed analysis could also include the effects of magnetosheath mixing by Kelvin-Helmholtz waves in this dual magnetosphere configuration as it changes in local time.
It is noteworthy, for a long time, mainly influenced by the results of Geotail and ISEE-3 missions and back-of-envelope theoretical estimates, the open-closed field line boundary in Earth’s magnetotail was thought to be far beyond the orbit of the Moon (Petrukovich et al., 2016; Artemyev et al., 2017). However, the results of the THEMIS and ARTEMIS observations of the travelling compression regions (Imber et al., 2011; Zhao et al., 2016) indicate that boundary is located inside the present lunar orbit, between 31–55 RE. Despite many magnetospheric missions, that specific area remains practically unexplored (Tsyganenko et al., 2021).
3 Regions of closed and open field lines at low latitudes
The dynamics of the magnetosphere rely heavily on the magnetic topology of open and closed magnetic field lines. For instance, the Earth’s plasmasphere is a toroidally shaped high density low energy plasma region extending around the Earth that contains closed field lines and holds plasma that has diffused from the ionosphere, via ambipolar electric field, into the magnetosphere. This process either fills or repopulates regions emptied by storm time convection (Lemaire and Scherer, 1973; Ganguli, 1996; Gallagher and Comfort, 2016) with low L-shells coming to an equilibrium in about ∼24 h (Reinisch et al., 2004) after being depleted. Gallagher and Comfort (2016) calculated that the plasmasphere in the 3–5 L-shell range spends 13%–99% of the time, respectively, refilling from the ionosphere following storm time losses. The consequence is that for L > 3.5 the plasmasphere spends most of the time refilling, never reaching a state of equilibrium.
There is a clear distinction between the ionosphere and plasmasphere beginning with O+ dominance and limited space weather response in the ionosphere to H+ dominance at higher altitudes and strong space weather response in the plasmasphere. In addition, there is a notable transition from strong thermosphere-ionosphere collisional interactions and high-altitude chemistry to Coulomb collisions and the dominance of electrodynamic driven processes in the plasmasphere.
The location of the plasmapause, as shown in the three right panels from the IMAGE Extreme Ultraviolet (EUV) instrument in Figure 2, results from the interplay of the corotation with the Earth of the closed field lines and magnetospheric convection of the plasma together with plasmasphere refilling processes (Nishida, 1966) and can be variable in time as well as in local time as shown. The plasmapause separates the inner region where the plasma corotates with the Earth and the outer region where the magnetospheric convection dominates moving plasma to the magnetopause, where it is lost into the magnetosheath. Magnetospheric convection is created by the magnetosphere’s interaction with the solar wind producing an electric field that pushes plasma across field lines sunward which varies in magnitude with the solar wind conditions.
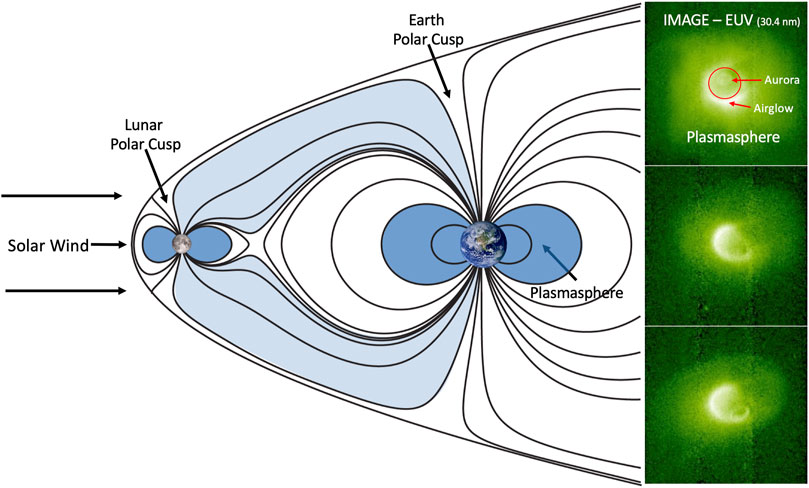
FIGURE 2. An example of the large regions of connected field line that would trap ionosphere plasmas that thermally diffuse outward into the magnetosphere. The IMAGE EUV observations on the right side illustrate the size and variations in today’s plasmasphere. The light blue colored field lines are of particular interest since they connect the polar regions of the Earth and the Moon.
The left panel of Figure 2 illustrates a new magnetic topology with the introduction of the lunar magnetosphere with the Moon at local noon at a location of about 18 RE. As a comparison with today’s conditions, the dark blue region around the Earth signifies a normal plasmasphere size observed by satellite with today’s Moon at 60 RE. Figure 2 also illustrates that with the addition of a strong lunar magnetosphere, an overall topology of the inner magnetosphere of largely closed magnetic field lines is developed, which likely blocks much of the plasma convection to the magnetopause establishing a new plasmapause between the dark and light blue shaded regions. In addition, during the era of the lunar atmosphere, a lunar ionosphere would also develop a plasmasphere around the Moon (see dark blue region of closed field lines around the Moon in Figure 2). At higher latitudes (light blue shading) the closed field lines would develop a lobe-plasmasphere which would contain counter-streaming outward flows from the Earth and the lunar ionospheres in addition to the solar-wind plasma that would E x B convect from the lunar cusp region into the dayside lobe. With the Moon on the dayside, on the nightside a traditional ring current, outer radiation belt, and terrestrial night-side aurora should arise. These current systems and aurora would significantly be modified with the Moon moving to the nightside within 2.25 days.
It is important to note that the coronal X-ray to EUV emissions of the young main-sequence Sun was 100–1000 times stronger than those of the present Sun (Ribas et al., 2005), which can energize ionospheric plasma, causing the rapid filling of connected flux tubes with the highly supersonic outflowing ionospheric plasma that corotate with the Earth and the Moon. The early Sun was characterized by enhanced solar activity in the form of strong X-ray to EUV emissions, frequent flares, and a powerful stellar wind (Dong et al., 2017; Airapetian et al., 2020). It is expected that as the Moon moves further from the Earth and its magnetosphere and atmosphere begin to decrease, magnetospheric convection will erode the plasma between the Earth and the Moon, which then evolves into a typical plasmasphere that is observed today.
3.1 Topics for further study
Additional research in this area could concentrate on the extent, growth rate, and erosion of the Earth and the Moon’s plasmasphere and lobe-plasmasphere, ring current location and evolution, and changes that would occur in the Van Allen radiation belts. For example, soon after the early Moon is formed, in the region of the outer radiation belt, the Moon will sweep out high-energy particles while others would diffuse inward to fill in the gap. As the Moon rapidly develops its magnetosphere, what other changes would develop? In addition, during storm time, the position of the Moon is expected to change the ring current development in the inner magnetosphere, but to what extent and how does the dipole orientation change the dynamics (e.g., does only a distant partial ring current form)? In addition, depending on how violent the volcanic outgassing is from the early Moon, does this enable a lunar torus of material to develop, much like the Io torus at Jupiter (Thomas et al., 2004), and if so, what additional effects would occur?
4 Regions of closed field lines at high latitudes
With the introduction of the lunar magnetosphere, the reconfigured field lines extend to greater distances and into the polar caps. The light blue shading of the Earth-Moon field lines, in Figure 2, shows the extent of pole-to-pole connected magnetic topology forming the lobe-plasmasphere. Figure 3 depicts the evolution of these lobe-plasmasphere connected field lines from a north pole looking downward perspective (x and y plane) where the Moon is at 4, 8, and 18 RE. The top three panels have the Moon on the nightside, and the bottom three panels are when the Moon is on the dayside. These field lines would provide a natural path for the movement of ionized material to the lunar poles.
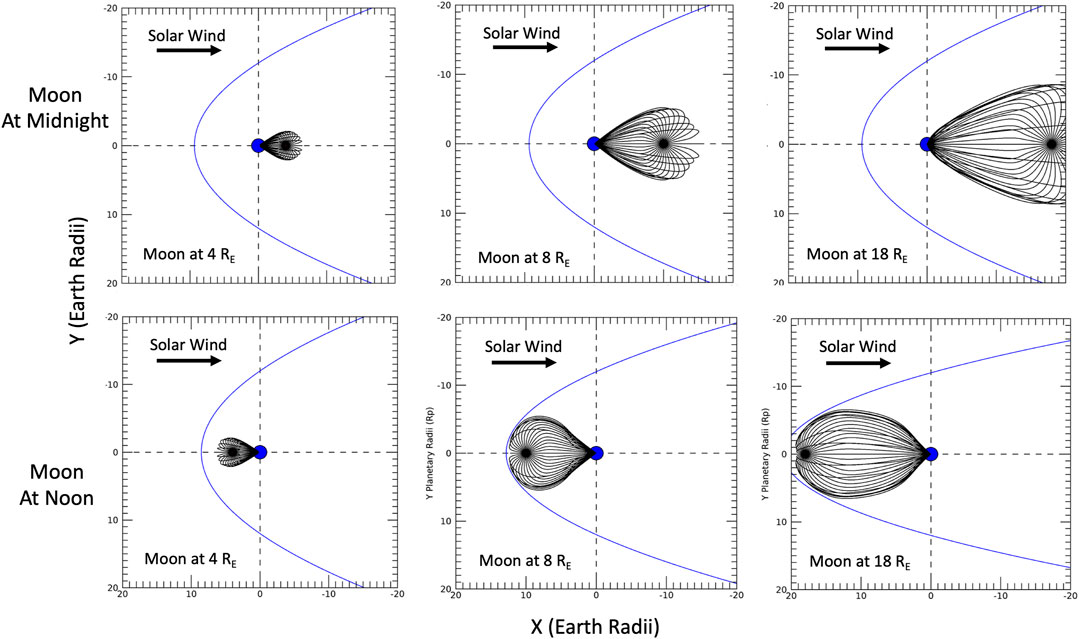
FIGURE 3. A top view of the Earth-Moon connected polar field lines referred to as the lobe-plasmasphere, showing their extent in local time and distance from the Earth when the Moon is located on the nightside (top three panels) and on the dayside (bottom three panels).
Craven et al. (1985) report the presence of molecular ion N2+, NO+, and O2+ flux outflowing from the Earth’s ionosphere into the plasmasphere without the assistance of lunar gravity, which would be active in the case of a lobe-plasmasphere. There is also good reason to expect that fumarolic gases would be present in the ionosphere during the volcanic active conditions 4 billion years ago (Byr) on the Moon and the Earth. These gases will be drawn outward and deposited on the lunar surface. Green (2011) reports that “common terrestrial fumarolic fluids may include water (H2O), carbon dioxide (CO2), sulfur (S), sulfur dioxide (SO2), carbon monoxide (CO), sulfur dichloride (SCl2), methane (CH4), ethane (C2H6), carbonyl sulfide (COS), hydrogen sulfide (H2S), ammonia (NH3), cyanogen (CN), and hydrogen cyanide (HCN) that are of major importance”.
Remote sensing data from the Lunar Reconnaissance Orbiter (LRO) has determined that the Moon holds a significant amount of frozen H2O and OH in and around its permanently shadowed regions (PSR) as shown in the left panel of Figure 4 (adapted from Sanin, et al., 2017) as a blue-shaded region over top of the lunar south pole image. The lunar north pole (not shown here) has a similar pattern.
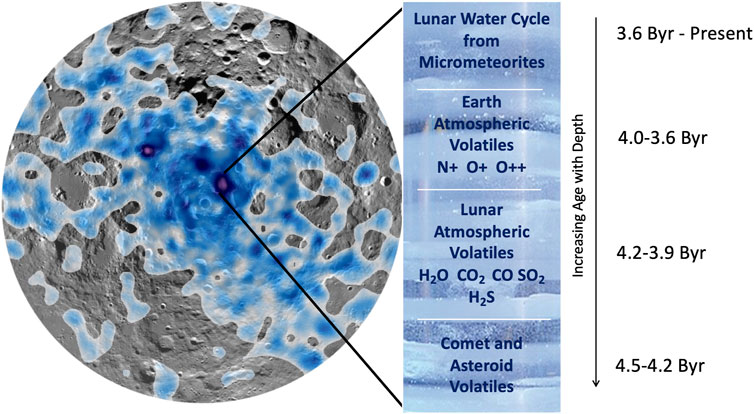
FIGURE 4. An expected distribution of water or hydroxyl in the lunar south pole is shown in the blue shading that has been adapted from Sanin et al., 2017. Based on the evolution of the lunar magnetic field and atmosphere over time, the right panel lists the expected dominant source of water and other volatiles deposited in the south polar cap over time.
Little is known about the transport of volatiles on the Moon into the surface and subsurface of PSRs. In 2009, to determine the nature and amount of hydrogen (water, H2O, and hydroxyl, OH) trapped in the permanently shadowed regions, the Lunar Crater Observation and Sensing Satellite (LCROSS) mission was designed to impact the shadowed Cabeus crater such that the ejecta could be spectrally analyzed. In addition to the water and hydroxyl the investigators were looking for, Colaprete et al. (2010) and Gladstone et al. (2010) identified, through a combination of IR and UV spectroscopy, a surprising number of other volatiles ejected from the impact region such as H2S, SO2, H2, NH3, C2H4, and CO2 and a host of other minor constituents such as CH3OH and CH4. The origin of these volatiles is still controversial, and the presence of sulfur compounds may even be indicative of volcanic sources. Recent further analysis by Mandt et al. (2022) stated that “no combination of known sources is able to match the large abundance of both sulfur and nitrogen compared to carbon measured by LCROSS” causing the authors to consider differences in what may be delivered to the lunar surface versus what eventually gets trapped in a PSR. But those authors did not consider the effect of the Earth-Moon coupled magnetospheres may have in the delivery over time of volatiles from all sources, including the Earth.
It is probable that the volatiles in the PSRs were collected over time during important events in the history of the evolution of the early Earth-Moon couple magnetospheres, since the connected field lines, in Figure 3, provide significant pathways for volatiles to collect into the cold traps of the lunar poles over hundreds of millions of years. The right panel of Figure 4 provides an overview of the potential evolution of volatiles in the polar region explained below. Current estimates are that there may be as much as a hundred billion metric tons of frozen water in these PSRs (Rubanenko et al., 2019). The early bombardment history of the Moon and Earth leads to having volatiles, especially water from comets and asteroids, naturally being captured in the PSRs. The late heavy bombardment (LHB) period would have provided another era in which large amounts of volcanic molten rock material filled huge basins creating the Mare and, in addition, the lunar volcanos liberated huge amounts of trapped subsurface gases, creating a thin non-negligible lunar atmosphere (Needam and Kring, 2017). Over a short period of time, it is expected that the lunar atmospheric volatiles made their way to the poles and collected in the cold traps of the PSR.
One fascinating aspect of these connected field lines is the distinct possibility that volatiles in the early Earth atmosphere would have had a clear pathway to the lunar poles, for long periods of time (Garrick-Bethell et al., 2019; Green et al., 2020; Poppe et al., 2021). Earth atmosphere volatiles moving up the closed field lines and accumulating into the lunar polar cold traps would be enhanced due to the expected intense ionizing ultraviolet radiation from the early Sun (Ribas, et al., 2005). In addition to water, it now appears that the PSRs may also hold volatiles like carbon dioxide, sulfur dioxide, and nitrogen. Once these volatiles get into a PSR they will stay there since the light from the Sun is blocked, and therefore there is no energy to break the frozen volatiles into their components, causing them to drift away in the solar wind.
Another important process of lunar polar cold traps accumulating water continues to this day but on a much-reduced level. The continual micrometeoroid impacts on the Moon, during Earth’s encounters with comet and asteroid debris trails, produces the liberation of sub-surface water (Benna et al., 2019), forming clouds of water vapor around the Moon. In addition to this process of losing water that was either delivered in the distant past or present at its formation, some of this water should migrate and become trapped in the lunar polar cold traps. In summary, the right-hand panel of Figure 4 illustrates potential layers of volatiles from the earliest bombardment with asteroids and comets, the early lunar and Earth atmospheres, and finally, the current micrometeoroid gardening.
4.1 Topics for further study
Even a tenuous lunar atmosphere/ionosphere may be sufficient to support a current between the Earth and the Moon that would create a constant lunar aurora much like the Jupiter-Ganymede magnetospheric configuration we see today (Wang et al., 2018; Waite et al., 2023). The lunar atmosphere would also be bathed in solar energetic particles and cosmic rays all of which would produce a variety of new atmospheric chemistry that may explain the large variety of volatiles found by the LCROSS impact in the Cabeus crater. In addition, interpreting future observations of volatiles that will be measured by several missions going into the lunar polar PSRs will produce several new theories and relationships not realized in this short paper. For instance, the Volatiles Investigating Polar Exploration Rover, or VIPER, with three spectrometers and a 1-m drill will investigate surface and subsurface volatiles in and near the Nobile Region of the lunar south pole (https://www.nasa.gov/press-release/nasa-s-artemis-rover-to-land-near-nobile-region-of-moon-s-south-pole).
5 Regions of open field lines at high latitudes
The location of the modern-day polar cap, or the region of open field lines connected to the solar wind at high latitudes, changes significantly during substorms (see, for example, Milan et al., 2003). During very quiet periods, the furthest extent of the open polar field lines resides near 80o latitude. The migration of this open field line region to lower latitudes is due to the reconfiguration of the magnetosphere when the IMF reconnect with the Earth’s magnetosphere on the dayside, resulting in peeling off dayside flux tubes, now connected to the solar wind, and moving them to the nightside. With the addition of the early lunar magnetosphere this situation becomes much more complicated.
As a static example, the mapping of the Earth-Moon closed fields of Figure 3 is shown in greater detail in Figure 5 in both Mercator (top panel) and polar (bottom panel) projections. Figure 5 considers the situation with the Moon at midnight at distances of 4 RE (black), 8 RE (blue), 10 RE (green), and 18 RE (red). These oval shaped regions will contain the closed field lines. It is important to point out in this simple analysis that since the lunar magnetosphere completely resides within the Earth’s magnetosphere the lunar polar region field lines connect only to the Earth and not the solar wind as shown in the bottom right-hand panel of Figure 5. In addition, this configuration does not mean that solar wind plasma cannot reach the lunar polar cap region since the E X B will push solar wind plasma into these closed field lines resulting in precipitation into the lunar polar cap atmosphere or surface. Determining the extent of the solar wind lunar polar cap penetration is beyond the scope of this paper, but this penetration may be limited (Chappell et al., 2021).
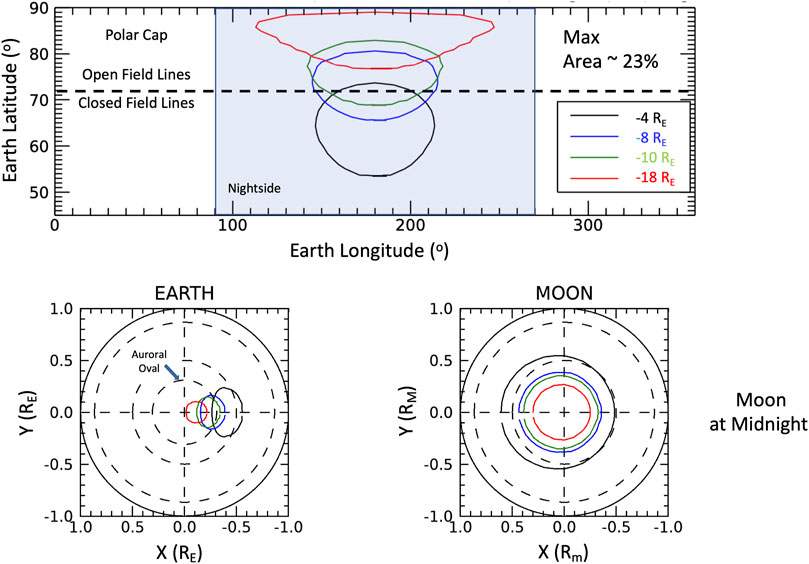
FIGURE 5. Top panel is a Mercator projection of the Earth-Moon interconnected field lines when the Moon is at midnight. The bottom two panels show the same connected regions on the Earth and the Moon in polar coordinates. The curves are for four positions of the Moon at 4, 8, 10, and 18 RE from the Earth. The expected location of the open-closed field lines during a moderate substorm conditions is shown as a dashed line.
The bottom left-hand panel of Figure 5 shows the corresponding mapping of the lunar field lines onto the Earth’s polar cap with a modern average auroral oval shown only as a comparison. The extent and location of these closed field lines do connect into the traditional open polar cap field lines. The left hand-panel polar view is expanded in a Mercator projection in the top panel of Figure 5. The nightside is the shaded light blue region. An average modern auroral oval, during a low to modest substorm, is delineated by a dashed line separating notional open and closed field lines which extends to both the day and night sides. The dashed line would move to lower latitudes during times of increased substorm intensity. The dashed line is only the modern view of the polar cap, within this simple analysis, and does not consider the addition of the Earth-Moon connected field lines.
It is expected that the location of the early Moon from the Earth will affect the size of the open field line polar cap region since the closed field lines between the Earth and the Moon do not participate in the polar cap ionospheric loss processes in the same way, because they are not connected directly to the solar wind. To illustrate this affect, the top panel of Figure 5 also shows the connected Earth-Moon magnetosphere field lines with lunar distance. Qualitatively, the area of the open polar cap field lines would refer to the area outside the color contours and above the dashed line.
As a qualitative estimate of this effect, the polar cap open field line region, shown in Figure 5, in each of the lunar distances, decreases in size as the Moon moves further from the Earth, reaching a maximum of ∼23% reduction in open polar cap field lines with the Moon at 18 RE. This reduction in open polar cap field lines is significant and would greatly vary during an entire substorm period.
Similarly, Figure 6 has the Moon at noon with the Mercator projection in the top panel and the polar coordinate of the connected field lines at the lunar pole (lower left panel) and Earth pole (lower right panel). The Mercator projection is oriented with the Earth’s local noon at the center of the plot. It is important to note that the polar cusp would appear at higher latitudes, above the connected field line ovals for each of the lunar positions, which minimizes the solar wind entry into the Earth’s polar cap and reduces the ionospheric heating from the cusp. Using this simple analysis as before, the lunar connected field lines, on the dayside, to the Earth’s polar cap will limit the number of open field lines limiting the amount of atmospheric escape by nearly 20% as a qualitive estimate. This example is just illustrative of the major change in magnetospheric dynamics expected from an embedded early lunar magnetosphere.
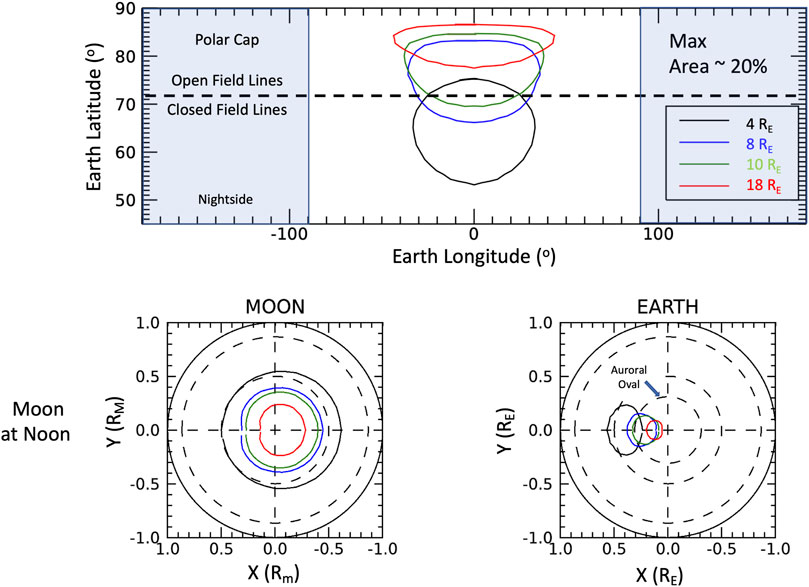
FIGURE 6. The same structure of Mercator and polar projections as in Figure 5 but when the Moon is at local noon.
5.1 Topics for further study
The example of a low or modest substorm conditions as given above is only the start of a more comprehensive analysis where a more active young Sun would extend the polar cap to much lower latitudes over longer periods of time. Rates of atmospheric escape under conditions of higher fluxes of extreme solar ultraviolet radiation with the early Earth and Moon atmospheric compositions during the period of the young Sun should also be explored (Airapetian et al., 2017). The dynamics of the plasma within the Earth-Moon connected field lines within the polar cap regions should also be investigated since it would contain not only the low energy ionospheric plasmas from the Earth and Moon upper atmospheres but also the convected solar wind plasma entering these flux tubes. MHD modeling would be of significance in quantifying total atmospheric loss under the range of expected solar storms considering the closed field lines of the connected early Earth and Moon magnetospheres.
6 Conclusion
This paper conducts a high-level first-order qualitative analysis of the circulation of plasma in the early Earth-Moon magnetospheres by developing an expected magnetic topology showing that the magnetosphere of the Moon must produce more closed field lines and restricts the escape of the early Earth atmosphere into space under certain conditions. In addition, this configuration would allow pathways along magnetic field lines to the lunar poles for volatiles to collect in the PSRs. The ravaging of planetary atmospheres in our young Solar System due to extreme solar radiation and particle fluxes is believed to be a significant factor in understanding how the Solar System planets develop and retain an atmosphere. This paper illustrates that the Moon may have played an unexpected and significant role in protecting the Earth’s atmosphere from the ravages of the young Sun’s intense space weather.
This paper also made a prediction, illustrated by Figure 4, as to what might be found in the PSRs since it must be a time capsule of the evolution of volatiles in the Earth-Moon system. Today, planetary scientists want to enter these regions and core them to provide a proper assessment of what is there. That assessment would be critical to verifying the prediction presented in this paper. As shown in Figure 4, the trapped volatiles may be able to tell us about the composition of the very early asteroids and comets, and the early atmospheres of the Earth and Moon. Because of the continual micrometeoroid impacts on the Moon mixing the soils and creating regolith, these cores are unlikely to capture an obvious perfect record of the time history of the volatiles, but they will reveal clues (composition, relative abundance, and isotopic ratios) available in no other way about where these volatiles came from and how they were preserved.
This paper only touches on the evolution of the early Earth and Moon magnetospheres, which has been a largely neglected space physics problem and has great potential for complex follow-up studies using more advanced tools such as MHD simulations. The study of this very early period of a lunar magnetosphere will also be greatly aided through NASA’s Artemis Program with the recovery and careful analysis of lunar polar samples that preserve sample orientation allowing the paleomagnetism to be much better characterized over time. In addition, the potential discovery of previously unrecognized volatiles in PSRs would also stimulate new research in this area. There are many open questions and areas of future study that could be explored that should provide insight as to how the early Earth survived the period known as the faint young Sun.
Data availability statement
The original contributions presented in the study are included in the article/Supplementary material, further inquiries can be directed to the corresponding author.
Author contributions
JG was responsible for the initial concept, guided the modeling, and managed co-author contributions to the paper. SB developed and executed the computer analysis. CD was involved in the parameterization of the model. All authors contributed to the article and approved the submitted version.
Funding
This research was funded by NASA. CD was supported by Princeton Plasma Physics Laboratory through the Laboratory Directed Research and Development (LDRD) Program under DOE Prime Contract No. DE-AC02-09CH11466 and NSF Grant No. AGS-2149787.
Conflict of interest
Author JG was employed by Space Science Endeavors.
The remaining authors declare that the research was conducted in the absence of any commercial or financial relationships that could be construed as a potential conflict of interest.
Publisher’s note
All claims expressed in this article are solely those of the authors and do not necessarily represent those of their affiliated organizations, or those of the publisher, the editors and the reviewers. Any product that may be evaluated in this article, or claim that may be made by its manufacturer, is not guaranteed or endorsed by the publisher.
References
Airapetian, V. S., Barnes, R., Cohen, O., Collinson, G. A., Danchi, W. C., Dong, C. F., et al. (2020). Impact of space weather on climate and habitability of terrestrial-type exoplanets. Int. J. Astrobiol. 19 (2), 136–194. doi:10.1017/s1473550419000132
Airapetian, V. S., Glocer, A., Khazanov, G. V., Loyd, R. O. P., France, K., Sojka, J., et al. (2017). How Hospitable are space weather affected habitable zones? The role of Ion Escape. Ap. J. L 836, L3. doi:10.3847/2041-8213/836/1/L3
Artemyev, A. V., Angelopoulos, V., Runov, A., and Vasko, I. Y. (2017). Hot ion flows in the distant magnetotail: ARTEMIS observations from lunar orbit to∼−200 RE. J. Geophys. Res. 122, 9898–9909. doi:10.1002/2017JA024433
Benna, M., Hurley, D. M., Stubbs, T. J., Mahaffy, P. R., and Elphic, R. C. (2019). Lunar soil hydration constrained by exospheric water liberated by meteoroid impacts. Nat. Geosci. 12, 333–338. doi:10.1038/s41561-019-0345-3
Cameron, A. G. W., and Benz, W. (1991). The origin of the Moon and the single impact hypothesis: IV. Icarus 92, 204–216. doi:10.1016/0019-1035(91)90046-v
Canup, R. M., and Asphaug, E. (2001). Origin of the Moon in a giant impact near the end of the Earth’s formation. Nature 412, 708–712. doi:10.1038/35089010
Chappell, C. R., Glocer, A., Giles, B. L., Moore, T. E., Huddleston, M. M., and Gallagher, D. L. (2021). The key role of cold ionospheric ions as a source of hot magnetospheric plasma and as a driver of the dynamics of substorms and storms. Front. Astron. Space Sci. 8, 746283. doi:10.3389/fspas.2021.746283
Colaprete, A., Schultz, P., Heldmann, J., Wooden, D., Shirley, M., Ennico, K., et al. (2010). Detection of water in the LCROSS ejecta plume. Science 330, 463–468. doi:10.1126/science.1186986
Craven, P., Olsen, R., Chappell, C., and Kakani, L. (1985). Observations of molecular ions in the Earth's magnetosphere. J. Geophys. Res. 90 (A8), 7599–7605. doi:10.1029/JA090iA08p07599
Dong, C., Huang, Z., Lingam, M., Tóth, G., Gombosi, T., and Bhattacharjee, A. (2017). The dehydration of water worlds via atmospheric losses. Astrophys. J. 847 (1), L4. doi:10.3847/2041-8213/aa8a60
Dong, C., Meng, J., and Lingam, M. (2020). Atmospheric escape from TOI-700 d: Venus versus Earth analogs. Astrophys. J. 896 (2), L24. doi:10.3847/2041-8213/ab982f
Gallagher, D. L., and Comfort, R. H. (2016). Unsolved problems in plasmasphere refilling. J. Geophys. Res. 121, 1447–1451. doi:10.1002/2015JA022279
Garrick-Bethell, I., Poppe, A., and Fatemi, S. (2019). The lunar paleo-magnetosphere: implications for the accumulation of polar volatile deposits. Geophys. Res. Lett. 46, 5778–5787. doi:10.1029/2019GL082548
Gladstone, G. R., Hurley, D. M., Retherford, K. D., Feldman, P. D., Pryor, W. R., Chaufray, J. Y., et al. (2010). LRO-LAMP observations of the LCROSS impact plume. Science 330, 472–476. doi:10.1126/science.1186474
Green, J. (2011). Academic aspects of lunar water resources and their relevance to lunar proto-life. Int. J. Mol. Sci. 12, 6051–6076. doi:10.3390/ijms12096051
Green, J., Boardsen, S., and Dong, C. (2021). Magnetospheres of terrestrial exoplanets and exomoons: implications for habitability and detection. Astrophys. J. 907 (2), L45. doi:10.3847/2041-8213/abd93a
Green, J. L., Draper, D., Boardsen, S., and Dong, C. (2020). When the moon had a magnetosphere. Sci. Adv. 6, eabc0865. doi:10.1126/sciadv.abc0865
Imber, S. M., Slavin, J. A., Auster, H. U., and Angelopoulos, V. (2011). A THEMIS survey of flux ropes and traveling compression regions: location of the near-Earth reconnection site during solar minimum. J. Geophys. Res. 116, A02201. doi:10.1029/2010JA016026
Lemaire, J., and Scherer, M. (1973). Kinetic models of the solar and polar winds. Rev. Geophys. 11, 427–468. doi:10.1029/RG011i002p00427
Mandt, K. E., Mousis, O., Hurley, D., Bouquet, A., Retherford, K. D., Magaña, L. O., et al. (2022). Exogenic origin for the volatiles sampled by the Lunar Crater Observation and sensing satellite impact. Nat. Commun. 13, 642. doi:10.1038/s41467-022-28289-6
Milan, S. E., Lester, M., Cowley, S. W. H., Oksavik, K., Brittnacher, M., Greenwald, R. A., et al. (2003). Variations in the polar cap area during two substorm cycles. Ann. Geophys. 21, 1121–1140. doi:10.5194/angeo-21-1121-2003
Needham, D. H., and Kring, D. A. (2017). Lunar volcanism produced a transient atmosphere around the ancient Moon. Earth Planet. Sci. Lett. 478, 175–178. doi:10.1016/j.epsl.2017.09.002
Ngwira, C. M., Pulkkinen, A., Kuznetsova, M. M., and Glocer, A. (2004). Modeling extreme “Carrington-type” space weather events using three-dimensional global MHD simulations. J. Geophys. Res. 119 (6), 4456–4474. doi:10.1002/2013JA019661
Nichols, C. I. O., Weiss, B. P., Getzin, B. L., Schmitt, H. H., Béguin, A., Rae, A. S. P., et al. (2021). The palaeoinclination of the ancient lunar magnetic field from an Apollo 17 basalt. Nat. Astron 5, 1216–1223. doi:10.1038/s41550-021-01469-y
Nishida, A. (1966). Formation of plasmapause, or magnetospheric plasma knee, by the combined action of magnetospheric convection and plasma escape from the tail. J. Geophys. Res. 71, 5669–5679. doi:10.1029/jz071i023p05669
Petrukovich, A., Artemyev, A., and Nakamura, R. (2016). “Magnetotail reconnection,” in Magnetic reconnection astrophysics and space science library. Editors W. Gonzalez, and E. Parker (Cham: Springer). doi:10.1007/978-3-319-26432-5_7
Poppe, A. R., Garrick-Bethell, I., and Fatemi, S. (2021). Fractionation of solar wind minor ion precipitation by the lunar paleomagnetosphere. PSJ 2, 60. doi:10.3847/PSJ/abea7d
Reinisch, B. W., Huang, X., Song, P., Green, J. L., Fung, S. F., Vasyliunas, V. M., et al. (2004). Plasmaspheric mass loss and refilling as a result of a magnetic storm. J. Geophys. Res. 109, A01202. doi:10.1029/2003JA009948
Ribas, I., Guinan, E. F., Gudel, M., and Audard, M. (2005). Evolution of the solar activity over time and effects on planetary atmospheres. I. High-Energy irradiances (1–1700 A). Astrophys. J. 622, 680–694. doi:10.1086/427977
Rubanenko, L., Venkatraman, J., and Paige, D. A. (2019). Thick ice deposits in shallow simple craters on the Moon and Mercury. Nat. Geosci. 12, 597–601. doi:10.1038/s41561-019-0405-8
Sanin, A. B., Mitrofanov, I. G., Litvak, M. L., Bakhtin, B., Bodnarik, J., Boynton, W., et al. (2017). Hydrogen distribution in the lunar polar regions. Icarus 283, 20–30. doi:10.1016/j.icarus.2016.06.002
Tarduno, J., Cottrell, R., Lawrence, K., Bono, R. K., Huang, W., Johnson, C. L., et al. (2021). Absence of a long-lived lunar paleomagnetosphere. Sci. Adv. 7, eabi7647. doi:10.1126/sciadv.abi7647
Thomas, N., Bagenal, F., Hill, T. W., and Wilson, J. K. (2004). “Chapter 23: the Io neutral clouds and plasma tours,” in Jupiter: the planet, satellites and magnetosphere. Editors F. Bagenal, T. Dowling, and W. McKinnon (Cambridge, United Kingdom: (Cambridge University Press), 561–591.
Tikoo, S. M., and Jung, J. (2023). Establishing a lunar origin for paleomagnetic records in Apollo samples. Geophys. Res. Lett. 50, e2023GL105152. doi:10.1029/2023GL105152
Tikoo, S. M., Weiss, B. P., L Shuster, D., Suavet, C., Wang, H., and Grove, T. L. (2017). A two-billion-year history for the lunar dynamo. Sci. Adv. 3, e1700207. doi:10.1126/sciadv.1700207
Tsyganenko, N., Andreeva, V., Kubyshkina, M., Sitnov, M., and Stephens, G. (2021). “Data-based modeling of the Earth’s magnetic field,” in Magnetospheres in the solar system, geophysical monograph 259. Editors R. Maggiolo, N. André, H. Hasegawa, and D. Welling (Hoboken, United States: (Wiley and Sons, Inc), 617–633. doi:10.1002/9781119815624
Waite, J. H., Greathouse, T., and Carberry Morgan, S. (2023). How the juno ganymede flyby has changed our understanding of its aurora and atmosphere. Vienna, Austria: EGU General Assembly 2023, 24–28. doi:10.5194/egusphere-egu23-9005
Wang, L., Germaschewski, K., Hakim, A., Dong, C., Raeder, J., and Bhattacharjee, A. (2018). Electron physics in 3-D two-fluid 10-moment modeling of ganymede's magnetosphere. J. Geophys. Res. Space Phys. 123 (4), 2815–2830. doi:10.1002/2017ja024761
Keywords: magnetospheric dynamics, geomagnetic storms and substorms, solar wind-magnetosphere interactions, space weather, early Earth-Moon magnetospheres
Citation: Green J, Boardsen S and Dong C (2023) Effects of the evolving early Moon and Earth magnetospheres. Front. Astron. Space Sci. 10:1112233. doi: 10.3389/fspas.2023.1112233
Received: 30 November 2022; Accepted: 27 October 2023;
Published: 10 November 2023.
Edited by:
Joseph E. Borovsky, Space Science Institute (SSI), United StatesReviewed by:
Justin Holmes, Los Alamos National Laboratory (DOE), United StatesDennis Gallagher, National Aeronautics and Space Administration, United States
Copyright © 2023 Green, Boardsen and Dong. This is an open-access article distributed under the terms of the Creative Commons Attribution License (CC BY). The use, distribution or reproduction in other forums is permitted, provided the original author(s) and the copyright owner(s) are credited and that the original publication in this journal is cited, in accordance with accepted academic practice. No use, distribution or reproduction is permitted which does not comply with these terms.
*Correspondence: James Green, SmxncmVlbjFAZWFydGhsaW5rLm5ldA==