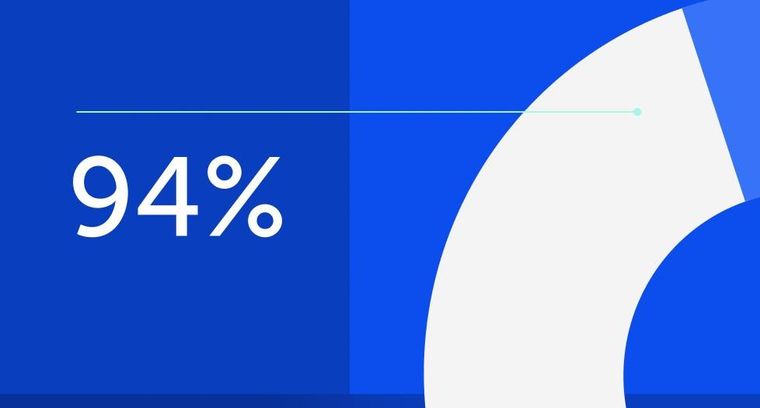
94% of researchers rate our articles as excellent or good
Learn more about the work of our research integrity team to safeguard the quality of each article we publish.
Find out more
HYPOTHESIS AND THEORY article
Front. Astron. Space Sci., 08 February 2023
Sec. Space Physics
Volume 10 - 2023 | https://doi.org/10.3389/fspas.2023.1060618
This article is part of the Research TopicThe Future of Space Physics 2022View all 66 articles
We highlight the importance of magnetic reconnection at the heliopause, both as one of the key processes driving the interaction between solar and interstellar media, but also as an element of the definition of the heliopause itself. We highlight the main observations that have fed the current debates on the definition, location and shape of the heliopause. We explain that discriminating between the current interpretations of plasma and magnetic field structures near the heliopause necessitates appropriate measurements which are lacking on Voyager 1 and 2, and describe some of the ensuing requirements for thermal plasma measurements on a future Interstellar Probe. The content of this article was submitted as a white paper contribution to the Decadal Survey for Solar and Space Physics 2024–2033 of the National Academy of Sciences.
Launched in 1977, the Voyager 1 and 2 spacecraft, after touring several Solar System planets, crossed the termination shock in 2004 and 2007, respectively, at distances of ∼94 (Decker et al., 2005; Stone et al., 2005) and ∼84 AU (Decker et al., 2008; Stone et al., 2008). Past the termination shock they observed a previously unexplored reservoir of energetic ions and electrons, the inner heliosheath. A key population there are pick-up ions (PUIs, produced when neutral particles are ionized by either solar radiation, charge exchange with solar wind protons or electron impact ionization; e.g., Holzer, 1972; Gloeckler et al., 1994; Zirnstein et al. (2022)). They make up a major part of the plasma pressure in the heliosheath (Richardson et al., 2008; Zank, 2015; Dialynas et al., 2020; Dialynas et al., 2022). As distance increases, a fall-off in outward radial flow should be observed in the heliosheath, consistent with approaching an interface—the heliopause—where pressure balance should occur between plasmas and magnetic field of solar and interstellar origins.
The crossings of the heliopause into the Very Local Interstellar Medium (VLISM) by Voyager 1 and 2 were then reported to have occurred in 2012 (Burlaga et al., 2013; Gurnett et al., 2013; Krimigis et al., 2013; Stone et al., 2013; Webber and MacDonald, 2013) and 2018 (Burlaga et al., 2019; Gurnett and Kurth, 2019; Krimigis et al., 2019; Richardson et al., 2019; Stone et al., 2019) at ∼122 AU and ∼119 AU, respectively. Until then, models often overestimated the distance of the heliopause, placing it further (up to 30 AU or more) than the actual crossings (Kornbleuth et al., 2021; Kleimann et al., 2022). Observations showed similar heliopause distances at Voyager 1 and 2 while the termination shock locations were different by 10 AU. This suggested that the termination shock location is more variable, likely due to a higher susceptibility to solar variability (e.g., Izmodenov et al., 2009; Krimigis et al., 2019).
Soon after the reported Voyager 1 heliopause crossing, a debate arose as to whether that boundary was indeed the heliopause (Fisk and Gloeckler, 2014; 2016; 2022; Gloeckler and Fisk, 2015). The identification of the observed boundary as the heliopause was primarily based on an apparent depletion in solar type plasma, a depletion in Anomalous Cosmic Rays (ACR) thought to originate at the termination shock or inner heliosheath (Jokipii and Giacalone 1998; Schwadron and McComas 2006; Strauss et al., 2010), and an abrupt increase in plasma density and Galactic Cosmic Rays (GCR) of interstellar origin. A large increase in magnetic field strength was also observed, as well as an unexpected absence of magnetic shear across the heliopause (Burlaga et al., 2013; 2019). Flow properties also do not fit expectations in the heliosheath, as well as at and beyond the heliopause (Decker et al., 2012; McComas and Schwadron, 2014; Cummings et al., 2021; Dialynas et al., 2021; Fuselier et al., 2021; Richardson, 2021; Richardson et al., 2022).
These confounding observations show that the physics governing the location, shape and very nature of the heliopause remains mostly unknown. This largely stems from the lack of appropriate data at both Voyager 1 and 2 for fully characterizing low energy plasma and PUIs, which directly hinders a proper definition of the heliopause. In Section 2 we thus first discuss the definition of the heliopause, and then highlight key science questions on the role of magnetic reconnection at the heliopause and the need for low energy plasma measurements in its vicinity and beyond. Phenomena other than magnetic reconnection are of importance at the heliopause, but are not addressed here. Like at other planetary magnetospheres and astrospheres, the Kelvin-Helmholtz, interchange and Rayleigh-Taylor instabilities (Ruderman and Fahr 1993; Florinski et al., 2005; Krimigis et al., 2013; Borovikov and Pogorelov, 2014; Strumik et al., 2014; Florinski, 2015; Zank, 2015; Korolkov et al., 2020; Dialynas et al., 2021; Opher et al., 2021), for instance, have been shown to play important roles at outer heliospheric boundaries, but vast amounts of works in many domains over the last decades have shown that magnetic reconnection is ubiquitous in the Universe and central to the properties and dynamics of numerous astrophysical objects.
Taking well-known boundaries at Earth as examples, by construction the plasmapause and magnetopause are well defined. The plasmapause (e.g., Pierrard et al., 2021) is defined as the boundary where a strong gradient in plasma density occurs in the inner magnetosphere such that there are almost no more low energy particles outside of this boundary. It is thus identified based on density measurements. The Earth’s magnetopause (e.g., Kivelson and Russell, 1995), by contrast, is defined as a magnetic boundary and thus typically identified using magnetic field data. It is the current sheet that separates regions where either the solar or the terrestrial magnetic field dominates. Importantly, it must be noted that for the magnetopause, because of the occurrence of magnetic reconnection, vast amounts of solar plasma are penetrating through the boundary and populating large regions of the Earth’s magnetosphere at all times (boundary layers, cusp, plasma mantle, etc.). Although there are regions on its inside with plasma from the external medium, the magnetopause is not defined based on which particle populations are observed, but is defined as the outermost current sheet where the magnetic field rotates to become solar-dominated.
The term “Heliopause”, by contrast, leaves some ambiguity on its definition. It refers to the outer boundary of the heliosphere but does not say if identification should be based on thermal plasma, energetic particles or field observations, and on what signature thereof. Voyager 1 and 2 have clearly entered a region that has much higher plasma density (Gurnett et al., 2013; Gurnett and Kurth 2019), contains significant amounts of interstellar plasma populations (e.g., GCR; Webber and MacDonald, 2013), where at least some particle populations of heliospheric origin (e.g., ACR; Webber and MacDonald, 2013) seem to have disappeared and where plasma flows have changed significantly (Richardson et al., 2019; Dialynas et al., 2021; Richardson, 2021; Giacalone et al., 2022; Richardson et al., 2022). The vast majority of the community holds these facts as overwhelming evidence that Voyager 1 and 2 have exited into the VLISM.
However, in addition to locations closer than initially predicted by models, the purported heliopause crossings at Voyager 1 and 2 show increases in magnetic field strength higher than expected (but cf. Fuselier and Cairns, 2013; Cairns and Fuselier, 2017), as well as magnetic shears across the heliopause that are much lower than remote observations and models would predict at both spacecraft (Burlaga et al., 2013; 2019). The magnetic field past the heliopause was found to be very stable with unexpectedly high magnetic field and solar-like orientation (Burlaga and Ness 2016), and this remains true to the current day for both Voyager 1 and 2 for many AUs beyond the heliopause. The chances for both spacecraft to have crossed the heliopause, if defined based on magnetic field measurements alone, at locations where the magnetic shear is so low appears extremely unlikely. In addition, recent analyses suggest there exists a radial plasma flow at the heliopause at both Voyager 1 and 2, outward from the inner heliosheath out into the VLISM. Such observations are at odds with theoretical expectations for the outer heliosphere and heliopause (Cummings et al., 2021; Dialynas et al., 2021; Fuselier et al., 2021; Richardson et al., 2022), although they are proposed to be consistent with the model of Fisk and Gloeckler (2022), as discussed below. Note, however, that there are instrumental uncertainties with the particle measurements near the heliopause at Voyager 2 (Richardson, 2021).
There is currently no consensus on the interpretation of these magnetic field and flow observations. It points to the lack of appropriate low energy plasma measurements on Voyager 1 and 2, which are critical to determine bulk plasma properties in the few eV to few tens-of-keV range (thermal particles in solar wind, heliosheath, and VLISM, as well as PUIs). We argue in this WP that magnetic reconnection plays a pivotal role in solar-interstellar interaction and in structuring the neighborhood of the heliopause, whatever its definition.
Many recent works have studied the occurrence and impact of magnetic reconnection at the heliopause (e.g., Swisdak et al., 2010; 2013; Fisk and Gloeckler, 2014; 2022; Strumik et al., 2014; Fuselier and Cairns, 2017; Opher et al., 2017; Fuselier et al., 2020). A prime characteristic of magnetic reconnection is that despite being triggered at small scales (electron and ion scales), it affects the system on a global scale (MHD and much beyond, e.g., the dynamics of whole magnetospheres, solar corona, etc.). In particular, magnetic reconnection allows plasma to protrude across discontinuities that otherwise would be impermeable. A second fundamental impact of magnetic reconnection is that it affects the system’s magnetic topology at very large scales (e.g., Hesse and Cassak, 2019).
This latter fact is illustrated in Figure 1A for the Earth’s magnetosphere. The context displayed is that of an interplanetary magnetic field (IMF) that has an orientation similar (nearly parallel) to that at the nose of the Earth’s magnetosphere. In such a case, the magnetic shear across the magnetopause is low. Magnetic reconnection does not occur near the nose (as it does for southward IMF), but instead occurs at high latitudes above both polar cusps (as marked with blue arrows) and may lead the same IMF field line to reconnect twice with the Earth’s magnetic field (green field line labelled 3). Although reconnection occurs over extended X-lines (out of the plane of the figure), the reconnection region is very small in comparison to the large-scale topological changes that affect the whole dayside magnetopause surface (here the magnetopause is defined as the current sheet separating the solar and Earth’s magnetic fields, just inside of field line 3). This large-scale change in topology leads to significant changes in the plasma properties in the dayside regions. Particles flow across the open magnetopause and form various types of boundary layers on both sides of the current sheet (inside and outside), with signatures of the penetration, disappearance and mixing of populations of various types and energies as a function of time, location, species, etc. These effects are very well documented at Earth (e.g., Gosling et al., 1990).
FIGURE 1. (A) Earth’s dayside topology when magnetic fields are parallel at the nose of the magnetosphere, leading to complex boundary layers inside (cusp) and outside (magnetosheath) the magnetopause (red dotted line) as a result of reconnection occurring twice and sequentially on the same magnetic field line. Blue arrows adjacent to field lines (1) and (2) illustrate the presence and direction of streaming, cold solar wind electrons. Red arrows, on field lines (2) and (3), correspond to streaming heated electrons. Field line (1) is a pristine field line open to the solar wind at both ends. Field line (2) is a field line which has reconnected in only one hemisphere. Field line (3) has reconnected in both hemispheres. Adapted from Lavraud et al. (2006) (B) MHD simulation result of the heliospheric interaction with the Very Local Interstellar Medium (VLISM). The heliopause is approximated with an isocontour in temperature (green shading). A magnetic field lines is shown in black. It results from double reconnection of a VLISM field line (straight extensions of the field line to the top and bottom) with the wound field (spiral) of the heliosphere. Adapted from Opher et al. (2017).
The outer heliospheric system is obviously different, but similarities also exist. This is illustrated in Figure 1B that displays the complex, wound structure of the magnetic field in the outer heliosphere (adapted from Opher et al., 2017). Although Voyager 1 and 2 observations show that the magnetic shear across the heliopause is lower than expected, the magnetic field orientation in the VLISM is expected to have a dominant orientation mostly parallel to that in the inner heliosheath at the nose. For that reason, recent modeling found that reconnection is not expected to occur along the nose (e.g., Swisdak et al., 2010; Fuselier and Cairns, 2017). It is instead expected on the flanks of the heliopause, as marked with blue arrows in Figure 1B. As shown by Opher et al. (2017), in such a configuration the same wound (Parker spiral) heliospheric field line may reconnect twice with the VLISM at widely separated locations (blue reconnection regions marked with arrows). Because it allows the same field lines to reconnect twice, this process bears an interesting resemblance with the Earth’s magnetosphere under northward IMF, as depicted in Figure 1A. As explained next, such topologies would mean that there exists complex, intricate signatures in particle and field measurements in the vicinity of the heliopause (cf; Fuselier and Cairns (2017) for instance).
Figure 1A illustrates the complex signatures expected in suprathermal electron flow (i.e., pitch angle) anisotropies at Earth, as an example. Toward the top of Figure 1A, next to field lines numbered (1 - black), (2 - blue) and (3 - green) the small blue and red vectors highlight pristine (i.e., rather cold) and heated, respectively, suprathermal electron flows. Heated electrons are the signature of the spacecraft being located on a reconnected field line, and the direction of the suprathermal electron flow signals the direction of the reconnection region. The small red arrows pointing northward next to field lines 2) and 3) at the top of Figure 1A signal the fact that reconnection has occurred southward along those field lines. However, the reconnection region may very well be at a very distant location (given the very high speed of suprathermal electrons) as is the case here with the reconnection region being located all the way above the cusp in the opposite, southern hemisphere. Field line 3) shows heated electrons also coming from the north (southward red arrow) because it is reconnected there also (field line 3) is doubly reconnected), but field line 2) shows pristine (cold, blue arrow) electrons coming from the north because this field line has not reconnected (yet) at this end. Depending on the timing of magnetic field line reconnection at both locations, as the spacecraft traverses the magnetopause it typically observes heated solar wind electrons streaming in both parallel and anti-parallel directions outside the magnetopause on doubly reconnected field lines (field line 3)) as well as unidirectional heated electrons which signal singly reconnected field lines (field line 2). The latter unidirectional heated electrons are observed on the outermost, sunward side of this boundary layer. Although not detailed here, the newly closed field lines eventually sink into the magnetosphere and form broad boundary layers inside the magnetopause (e.g., Lavraud et al., 2006; Lavraud et al., 2018; Øieroset et al., 2008). In other words, crossing the magnetopause from any given side one expects to observe a very complex, layered structure with variable particle properties corresponding to the mixing of particles from both sides (such complex signatures are observed at Earth also at other energies and for other species).
The double reconnection scenario of heliospheric and VLISM field lines unveiled in the simulation of Figure 1B should similarly lead to specific particle signatures in the broad vicinity of the heliopause. Such topological considerations have been put forth to explain the fact that the drop in ACR fluxes is not collocated (it occurs further outside) with the main magnetic field increase at the Voyager 1 and 2 heliopause crossings (e.g., Krimigis et al., 2013; Fuselier et al., 2020). Because ACRs are produced within the Heliosphere, their drop outside the reported heliopause crossings (as well as the timings and anisotropies of various species at different energies) is taken as evidence of particle leakage due to reconnected field lines in the vicinity of the heliopause, but remote from Voyager 1 and 2 (e.g., Fuselier et al., 2020), or other instabilities (cf. introduction). But the complete disappearance (in all directions) of ACRs has also been interpreted as an escape (in both parallel and anti-parallel directions) along doubly reconnected field lines as shown in Figure 1B (since the ACRs originate inside the Heliosphere). In this scenario, even past the drop in ACRs, the Voyager 1 and 2 spacecraft would remain magnetically connected to an open heliopause and thus remain inside the main current sheet that would signal the effective transition into the pristine VLISM (e.g., Fisk and Gloeckler, 2022). Particle measurements with complete directionality information are critical to fully investigate the large-scale heliopause magnetic topology.
To first order, the analogy between Figures 1A and 1B stops at the fact that the same field lines may reconnect twice at widely separated distances (when conditions at the nose are not favorable for reconnection to occur there). This is because the signatures in terms of magnetic field (pile-up, orientation) and particles species, energies, and anisotropies are widely different in both contexts (different particle sources, coexistence of multiple populations (e.g., PUIs), magnetic geometries, system size, presence/absence of shocks, instabilities at play, etc.). As further discussed in the next section, the width of these possible heliopause boundary layers, although not known, could be substantial. Furthermore, the non-vanishing outward radial flow observed at both Voyager 1 and 2 near the heliopause is not consistent with most models, and comparison to the Earth’s magnetosphere does not provide insight in that respect. Determining where reconnection occurs, how plasma flows near the heliopause, and the ensuing large-scale magnetic topology requires more complete observations, in particular in the few eV to few tens-of-keV range (thermal and PUI). These are critical to better define the heliopause, its location and its shape.
Finally, it should be noted that magnetic reconnection along the heliopause has been proposed as one of the possible mechanisms to explain the ribbon structure of energetic neutral atom fluxes in global observations from the Interstellar Boundary Explorer (IBEX). However, given the alternating polarity structure of the heliospheric magnetic field along significant portions of the heliopause it is unclear how this process could explain the configuration and steadiness of the ribbon (McComas et al., 2009; McComas et al., 2011). The latter point has another important implication, and that is that the locations of magnetic reconnection on the heliopause surface, and therefore the large-scale magnetic topology of the heliopause, should change over the course of the solar cycle given the alternating magnetic field polarity.
The plasma properties of the outer heliosphere differ greatly from those of the inner heliosphere where magnetic reconnection has been widely studied. In particular, the pressure in the accumulated PUIs becomes larger than the solar wind thermal pressure already upstream of the termination shock, and at the termination shock the pickup ions are heated more than the thermal solar wind (Richardson et al., 2008; Mostafavi et al., 2017; Mostafavi et al., 2018). The plasma Beta conditions also largely change in the outer Heliosphere, past the termination shock, at and beyond the heliopause in the VLISM. Also, while asymmetric reconnection should be expected at the heliopause, symmetric reconnection may be occurring at current sheets in the inner (Drake et al., 2017) and outer heliosheath, as well as in the VLISM (although surprisingly no evidence has been reported for reconnection in the inner heliosheath so far, and in the VLISM current sheets are expected to be much less frequent). Studying magnetic reconnection in all these regions would thus provide significant new insights into our understanding of magnetic reconnection in new plasma regimes.
For example, concerning the inner heliosheath, Opher et al. (2011) proposed that the magnetic field and flows are not laminar, but instead consist of magnetic islands that develop upstream at the heliopause through reconnection (see also Schwadron and McComas (2013) regarding flux ropes at the heliopause). As discussed above the location of reconnection at the heliopause remains unclear, but it has been suggested that large pressure gradients across the heliopause due to PUIs produce a diamagnetic drift that may stabilize magnetic reconnection. This adds to the low magnetic shear observed at the Voyager 1 and 2 crossings, which likely precludes reconnection from occurring locally at the nose (Swisdak et al., 2010; 2013; Fuselier and Cairns, 2017; Opher et al., 2017; Fuselier et al., 2020). Magnetic reconnection may, however, develop at other locations on the flanks of the heliopause where conditions are satisfied thanks to higher magnetic shear (Opher et al., 2017).
Near the Earth’s magnetosphere and in the solar wind, where high accuracy thermal plasma measurements are available, detailed studies of the energy conversion and partitioning associated with reconnection can be made (e.g., Burch et al., 2016). Although there are obvious payload limitations compared to near-Earth missions, studying such questions in the vastly different outer heliosphere regimes would require dedicated low energy thermal plasma measurements, including PUIs, on a future Interstellar Probe. Studying the properties of reconnection in different regimes would also benefit from different types of wave measurements. This is true for example for the study of wave—particle interactions, but also because wave data can provide complementary measurements of plasma moments (such as density, as has been done on Voyager 1 and 2 in the absence of density measurement from thermal particle instruments (e.g., Gurnett and Kurth 2019; Kurth and Gurnett, 2020).
Using again an analogy with the Earth’s magnetopause: the timing, location, geometry and rate of magnetic reconnection should produce various layers of different widths as a function of species, energy and pitch angle. These boundary layers are not solely confined to one side (i.e., magnetosheath boundary layer marked on the outside of the magnetopause in Figure 1A), but rather are present on both sides of the current sheet (solar wind populates boundary layers on the inside of the magnetopause and the entire cusp region). If magnetic reconnection affects the topology of the heliopause on very large scales as illustrated in the simulation of Figure 1B, there is no doubt that significant effects in particle and field properties should be observed near the heliopause, but also possibly up to large distances inside and outside, although that remains unknown.
Indeed, the widths of the reconnection boundary layers on both sides of the current sheet depend on the reconnection rate (which depends on local plasma properties), on the distance from the reconnection region, as well as on the profile in Alfvén speed along the field lines as the reconnection kinks propagate on either side of the boundary. Again, the expected widths of the layers remain unclear for the heliopause. Some studies propose rather thin boundary layers, based on particle properties around the heliopause (Fuselier and Cairns, 2017; Fuselier et al., 2020). But other studies suggest the observed ACR leakage is actually occurring well inside the actual heliopause (which would not be crossed yet), in a much broader inner heliopause boundary layer that results from a reconnection process akin to that depicted in Figure 1B (e.g.; Fisk and Gloeckler, 2016; Fisk and Gloeckler, 2022). Again, in an analogy to the case of the Earth depicted in Figure 1A, it should be noted that the boundary layer that forms on the Earthward side (the high magnetic field side) of the magnetopause as a result of the process of double magnetic reconnection is typically of the order of one Earth radius, which is a significant size compared to the scale size of the dayside magnetosphere (∼10% of the ∼10 Earth radii magnetopause stand-off distance). If similar types of boundary layers were forming at the heliopause, they could be of very significant extents, possibly tens of AUs inside or outside the heliopause (depending on its definition). However, we lack the appropriate measurements on Voyager 1 and 2, particularly thermal plasma properties, to undoubtedly estimate the key parameters and decipher the complex structuring of all the expected types of boundary layers and their extents.
Boundary layers produced by magnetic reconnection are often intricately linked to draping and pile-up processes. This is true at the Earth’s magnetopause and is likely also true at the heliopause. Draping has been proposed to occur in the outer heliosheath, outside the heliopause, with an associated magnetic pile-up and plasma depletion region (e.g., Schwadron et al., 2009; Burlaga et al., 2013; Fuselier et al., 2020; Kornbleuth et al., 2021; Mostafavi et al., 2022), the thickness of which has been estimated as ∼5 AU (Fuselier and Cairns, 2013). The location of the plasma depletion layer has been proposed to be displaced from the nose, unlike at Earth, based on calculations that account for the different partitioning between thermal, kinetic and magnetic pressures in a high Beta environment (Fuselier and Cairns, 2013). As is the case for the Earth’s magnetosphere, the width, extent and location of this layer may impact where reconnection is triggered, as well as the stability of the reconnection process (Fuselier et al., 2000; Lavraud et al., 2005). An open question regarding these layers, however, is that while Gurnett et al. (2013) first reported densities of ∼0.09–0.11 cm−3 past the heliopause, higher densities up to ∼0.14 cm−3 were actually measured at distances ∼20 AU beyond, as reported in Gurnett and Kurth (2019) and Kurth and Gurnett (2020) for both Voyager spacecraft.
A plasma depletion layer has also been studied (Izmodenov and Alexashov 2015) on the inside of the heliopause. As for the outer heliosheath pile-up region, the magnetic field pile-up forms gradients that decelerate and deflect plasma flows around and to the side of the obstacle formed by the heliopause. As a result, plasma density is depleted from the middle of the inner heliosheath to the heliopause. However, the effects of charge exchange between interstellar ions and neutrals should be significant and affect plasma flows and density depletion both inside and outside the heliopause. How these plasma depletion and magnetic pile-up layers develop and affect the interactions at the heliopause remain open questions. Proper low energy plasma measurements are required to address these questions.
Finally, using MHD simulations Opher and Drake (2013) suggested that the draping outside the heliopause is strongly affected by the solar magnetic field, although the underlying physical mechanism was not understood. They showed that as the VLISM magnetic field approaches the heliopause, it twists and acquires an east–west component, an effect that did not occur if the solar magnetic field was not present in the simulation. This draping could explain the twist of the magnetic field and the unexpectedly low magnetic shear at Voyager 1 and 2 (cf. also Grygorczuk et al., 2014; Isenberg et al., 2015; Opher et al., 2017). This possibility, however, remains to be confronted by observations and such observations, in particular in terms of low energy particles, are lacking.
A dedicated outer heliosphere and interstellar space mission is required to understand the interaction of our heliosphere with the VLISM at the heliopause, as proposed in McNutt et al. (2022) and Brandt et al. (2022). The Mission Concept study Report (MCR) may be found at https://interstellarprobe.jhuapl.edu/Interstellar-Probe-MCR.pdf).
To tackle science questions related to reconnection at the heliopause, a number of important and complementary measurements are needed on such a mission. These include magnetic field, plasma wave, suprathermal and energetic particles (up to ACR and GCR energies) instruments (since such are available on Voyager 1 and 2 they are not the focus of this WP), but also importantly low energy particle measurements that are not present onboard Voyager 1 and 2 (although operating the Faraday Cups do not have appropriate fields-of-view (FoV) on Voyager 2 at and beyond the heliopause). Low-energy particle measurements should cover the few-eV to few-tens-of-keV range, which include the thermal solar wind, heliosheath and VLISM populations, as well as PUIs. Other science questions and requirements related to PUIs are addressed in other WPs. We thus focus on measurement requirements for the lowest energy thermal populations here.
Bulk flow and thermal energies of low energy ions and electrons typically fall in the following ranges as a function of regions: solar wind from a few eV to 20 keV, inner heliosheath from eV to keV and outer heliosheath and VLISM are likely only in the few eV range, although this is not well constrained (e.g., Zank, 2015). Interstellar Probe shall fly further than 200 AU (possibly beyond 400 AU) into interstellar space. This leads to constraints in terms of requirements and design, the most critical being as follows.
• Ion and electron populations range from the dense solar wind at one AU to the very tenuous ionized medium at 200 AU and beyond, with a factor 10–6 lower fluxes/count rates expected. Measurements thus require low noise, high dynamic range detectors.
• The instrument shall be designed to work for at least 50 years. Technologies (electronics components) and detectors should have comparable life-time expectancies.
• Sampling time resolution should range from second (inner heliosphere) to hour (VLISM) time scales. Control and commanding (firmware), as well as onboard computations and packaging shall handle a large dynamic range of modes and operation cycles.
Two types of instruments can be foreseen to address these requirements, in a complementary fashion: Faraday cup (FC) and electrostatic analyzer (ESA). Having both would permit important redundancy of parts of the critical measurements (basic moments of the distribution function such as density, velocity and temperatures).
FCs are simpler in design and require fewer resources. FCs have proven to be a robust design for long term use on many missions, as for example on Voyager 2. An advantage of FCs is that it is straightforward to increase their geometry factor, as would be critical to detect low plasma densities. FCs are typically designed for measuring low energy ions, but given the low cadences required in the outer heliosphere the FCs for an interstellar mission should also include an electron mode. Because the spacecraft is expected to be spin-stabilized, three to four FC heads with appropriate FoV should be sufficient to make three-dimensional sampling and provide appropriate plasma moments. Each head may be sectorized to perform flow measurements. FCs have naturally a low response to noise from energetic particles, as these do not provide a significant current. But appropriate electronics should be used to suppress internal noise sources, and accurate high-voltage power supplies should be designed for the very low energies of the inner and outer heliosheath and VLISM.
An ESA instrument is fundamental as it provides information on the full particle distribution function (temperature anisotropies, pitch angles measurements, easier separation of species, etc.). It should have a 360° planar FoV (possibly 180°) and measure the full three-dimensional distribution function thanks to the spacecraft spin. A critical design aspect for that type of instrument concerns the high dynamic range of fluxes expected, which impacts the type of detector—Channel Electron Multipliers or Micro-Channel Plates—that should be used. While the former is typically more robust with time (lifetime) and has higher intrinsic dynamic range, the latter requires significantly lower resources (power). Another critical design aspect for such type of instrument is the signal-to-noise ratio, which owing to penetrating radiation and electronics clearly will require appropriate mitigation measures. An obvious concept to be used is a coincidence scheme, which should allow to reduce system background noise down to <10–4 s−1
As for the FCs, the ESA should be designed with low noise electronics and accurate high-voltage supply system down to low voltages (3 eV baseline, which is consistent with measurements to below the expected spacecraft potential of +5 V). To save significant resources, the instrument ideally should be able to measure both ions and electrons with the same detector head. State-of-the-art methods should be designed to perform such alternated measurements of ions and electrons to optimize front-end and high-voltage resources. Performing such alternated measurements of ions and electrons does not impact science requirements since measurement cadences do not need to be high in the outer heliosphere and VLISM.
The ESA instrument may be designed with a Time-of-Flight system to perform composition measurements down to low energies. In such a case, a clever design would be needed to perform both ions and electrons with the same head. Alternatively, half of the 360° FoV could be devoted to electrons and the other for ions. Or yet two separate heads/instruments could be used to ensure that ion composition is performed. It may be argued, however, that a low energy ESA instrument could be limited to measuring all ions without composition, but yet providing some composition information through energy separation (if the bulk flow is larger than the thermal speed), given that a dedicated PUI instrument with composition is required anyway and may go down to complementary low energies. While a PUI instrument could have limited angular resolution (e.g., 22.5°), an ESA instrument dedicated to the solar wind and VLISM should ideally have higher angular resolution (≤10°), at least in appropriate portions of the FoV. This aspect requires more detailed trade-off studies to find the appropriate design. Finally, we shall also note that the ESA instrument could be designed with a deflection system at the entrance to increase the FoV of the instrument when the spacecraft will be three-axis stabilized during planetary fly-bys, but this would be at the expense of reasonable, but non-negligible resources. We remind that more details on the measurement requirements (resolutions in energy, angle, time, mass etc.) are provided in the Mission Concept study Report (MCR) at https://interstellarprobe.jhuapl.edu/Interstellar-Probe-MCR.pdf).
The original contributions presented in the study are included in the article, any further inquiries can be directed to the corresponding author.
All authors listed have made a direct, intellectual contribution to the work and approved it for publication.
Work performed at LAB and IRAP in France were supported by CNES, CNRS, and the Universities of Bordeaux and Toulouse. The work at JHU/APL was supported also by NASA under contracts NAS5 97271, NNX07AJ69G, and NNN06AA01C and by subcontract at the Office for Space Research and Technology.
The authors are grateful to the Voyager and Interstellar Probe mission teams that have, and will continue, making the science discussed in this article possible. The authors additionally acknowledge valuable discussions with the Cassini/MIMI and SHIELD DRIVE Science Center teams.
The authors declare that the research was conducted in the absence of any commercial or financial relationships that could be construed as a potential conflict of interest.
All claims expressed in this article are solely those of the authors and do not necessarily represent those of their affiliated organizations, or those of the publisher, the editors and the reviewers. Any product that may be evaluated in this article, or claim that may be made by its manufacturer, is not guaranteed or endorsed by the publisher.
Borovikov, S., and Pogorelov, N. (2014). Voyager 1 near the heliopause. Astrophysical J. Lett. 783, L16. article id. L16. doi:10.1088/2041-8205/783/1/l16
Brandt, P. C., Provornikova, E., Cocoros, A., Turner, D., DeMajistre, R., Runyon, K., et al. (2022). Interstellar probe: Humanity's exploration of the galaxy begins. Acta Astronaut. 199, 364–373. doi:10.1016/j.actaastro.2022.07.011
Burch, J. L., Moore, T. E., Torbert, R. B., and Giles, B. L. (2016). Magnetospheric multiscale overview and science objectives. Space Sci. Rev. 199 (1-4), 5–21. doi:10.1007/s11214-015-0164-9
Burlaga, L. F., Ness, N. F., and Stone, E. C. (2013). Magnetic field observations as voyager 1 entered the heliosheath depletion region. Science 341 (6142), 147–150. doi:10.1126/science.1235451
Burlaga, L. F., Ness, N. F., Berdichevsky, D. B., Park, J., Jian, L. K., Szabo, A., et al. (2019). Magnetic field and particle measurements made by Voyager 2 at and near the heliopause. Nat. Astron. 3, 1007–1012. doi:10.1038/s41550-019-0920-y
Burlaga, L. F., and Ness, N. F. (2016). Observations of the interstellar magnetic field in the outer heliosheath:voyager 1. Astrophysical J. 829 (2), 134. article id. 134. doi:10.3847/0004-637X/829/2/134
Cairns, I. H., and Fuselier, S. A. (2017). The plasma depletion layer beyond the heliopause: Evidence, implications, and predictions forvoyager 2andnew horizons. Astrophysical J. 834 (2), 197. article id. 197. doi:10.3847/1538-4357/834/2/197
Cummings, A. C., Stone, E. C., Richardson, J. D., Heikkila, B. C., Lal, N., and Kota, J. (2021). Astrophysical J. 906, 126. Issue 2. doi:10.3847/1538-4357/abc5c0
Decker, R. B., Krimigis, S. M., Roelof, E. C., Hill, M. E., Armstrong, T. P., Gloeckler, G., et al. (2008). Mediation of the solar wind termination shock by non-thermal ions. Nature 454 (7200), 67–70. doi:10.1038/nature07030
Decker, R. B., Krimigis, S. M., Roelof, E. C., Hill, M. E., Armstrong, T. P., Gloeckler, G., et al. (2005). Voyager 1 in the foreshock, termination shock, and heliosheath. Science 309 (5743), 2020–2024. doi:10.1126/science.1117569
Decker, R. B., Krimigis, S. M., Roelof, E. C., and Hill, M. E. (2012). No meridional plasma flow in the heliosheath transition region. Nature 489 (7414), 124–127. doi:10.1038/nature11441
Dialynas, K., Galli, A., Dayeh, M. A., Cummings, A. C., Decker, R. B., Fuselier, S. A., et al. (2020). Combined -10 eV to -344 MeV particle spectra and pressures in the heliosheath along the voyager 2 trajectory. Astrophysical J. Lett. 905, L24. id.L24. doi:10.3847/2041-8213/abcaaa
Dialynas, K., Krimigis, S. M., Decker, R. B., and Hill, M. E. (2021). Ions Measured by Voyager 1 Outside the Heliopause to ∼28 au and Implications Thereof. Astrophysical J. 917 (1), 42. doi:10.3847/1538-4357/ac071e
Dialynas, K., Krimigis, S. M., Decker, R. B., Hill, M., Mitchell, D. G., Hsieh, K. C., et al. (2022). The structure of the global heliosphere as seen by in-situ ions from the voyagers and remotely sensed ENAs from Cassini. Space Sci. Rev. 218 (4), 21. article id.21. doi:10.1007/s11214-022-00889-0
Drake, J. F., Swisdak, M., Opher, M., and Richardson, J. D. (2017). The Formation of magnetic depletions and flux annihilation due to reconnection in the heliosheath. Astrophysical J. 837 (2), 159. article id. 159. doi:10.3847/1538-4357/aa6304
Fisk, L. A., and Gloeckler, G. (2022). Global structure and dominant particle acceleration mechanism of the heliosheath: Definitive conclusions. Astrophysical J. 927 (1), 73. doi:10.3847/1538-4357/ac4d2f
Fisk, L. A., and Gloeckler, G. (2014). On whether or notvoyager 1has crossed the heliopause. Astrophysical J. 789 (1), 41. article id. 41. doi:10.1088/0004-637X/789/1/41
Fisk, L. A., and Gloeckler, G. (2016). The Fisk and Gloeckler model for the nose region of the heliosheath: Another model for Ed Stone to test. J. Phys. Conf. Ser. 767 (1), 012008. article id. doi:10.1088/1742-6596/767/1/012008
Florinski, V., Zank, G. P., and Pogorelov, N. V. (2005). Heliopause stability in the presence of neutral atoms: Rayleigh-taylor dispersion analysis and axisymmetric MHD simulations. J. Geophys. Res. Space Phys. 110, A07104. Issue A7CiteID A07104. doi:10.1029/2004JA010879
Fuselier, S. A., and Cairns, I. H. (2013). Astrophysical J. 771 (2), 10. article id. 83. doi:10.1088/0004-637X/771/2/83
Fuselier, S. A., and Cairns, I. H. (2017). Reconnection at the heliopause: Predictions for voyager 2. J. Phys. Conf. Ser. 900, 012007. article id. doi:10.1088/1742-6596/900/1/012007
Fuselier, S. A., Galli, A., Richardson, J. D., Reisenfeld, D. B., Zirnstein, E. J., Heerikhuisen, J., et al. (2021). Energetic neutral atom fluxes from the heliosheath: Constraints from in situ measurements and models. Astrophysical J. Lett. 915 (2), L26. id.L26. doi:10.3847/2041-8213/ac0d5c
Fuselier, S. A., Petrinec, S. M., Bobra, M. G., and Cairns, I. H. (2020). Reconnection at the heliopause: Comparing the voyager 1 and 2 heliopause crossings. J. Phys. Conf. Ser. 1620 (1), 012004. article id. doi:10.1088/1742-6596/1620/1/012004
Fuselier, S. A., Petrinec, S. M., and Trattner, K. J. (2000). Stability of the high-latitude reconnection site for steady northward IMF. Geophys. Res. Lett. 27 (4), 473–476. doi:10.1029/1999GL003706
Giacalone, J., Fahr, H., Fichtner, H., Florinski, V., Heber, B., Hill, M. E., et al. (2022). Anomalous cosmic rays and heliospheric energetic particles. Space Sci. Rev. 218 (4), 22. article id.22. doi:10.1007/s11214-022-00890-7
Gloeckler, G., and Fisk, L. A. (2015). Astrophysical J. Lett. 806 (2), 5. article id. L27. doi:10.1088/2041-8205/806/2/L27
Gloeckler, G., Geiss, J., Roelof, E. C., Fisk, L. A., Ipavich, F. M., Ogilvie, K. W., et al. (1994). Acceleration of interstellar pickup ions in the disturbed solar wind observed on Ulysses. J. Geophys. Res. 99, 17637–17644. doi:10.1029/94JA01509
Gosling, J. T., Thomsen, M. F., Bame, S. J., Onsager, T. G., and Russell, C. T. (1990). The electron edge of low latitude boundary layer during accelerated flow events. Geophys. Res. Lett. 17 (11), 1833–1836. doi:10.1029/GL017i011p01833
Grygorczuk, J., Czechowski, A., and Grzedzielski, S. (2014). Astrophysical J. Lett. 789 (2), L43. article id. L43. doi:10.1088/2041-8205/789/2/L43
Gurnett, D. A., Kurth, W. S., Burlaga, L. F., and Ness, N. F. (2013). In situ observations of interstellar plasma with voyager 1. Science 341 (6153), 1489–1492. doi:10.1126/science.1241681
Gurnett, D. A., and Kurth, W. S. (2019). Plasma densities near and beyond the heliopause from the Voyager 1 and 2 plasma wave instruments. Nat. Astron. 3, 1024–1028. doi:10.1038/s41550-019-0918-5
Hesse, M., and Cassak, P. A. (2019). Magnetic reconnection in the space sciences: Past, present, and future. J. Geophys. Res. 125. doi:10.1029/2018JA025935
Holzer, T. E. (1972). Interaction of the solar wind with the neutral component of the interstellar gas. J. Geophys. Res. 77 (28), 5407–5431. doi:10.1029/JA077i028p05407
Isenberg, P. A., Forbes, T. G., and Möbius, E. (2015). Draping of the interstellar magnetic field over the heliopause: A passive field model. Astrophysical J. 805 (2), 153. article id. 153. doi:10.1088/0004-637X/805/2/153
Izmodenov, V. V., and Alexashov, D. B. (2015). Three-dimensional kinetic-mhd model of the global heliosphere with the heliopause-surface fitting. Astrophysical J. Suppl. Ser. 220 (2), 32. article id. 32. doi:10.1088/0067-0049/220/2/32
Izmodenov, V. V., Malama, Y. G., Ruderman, M. S., Chalov, S. V., Alexashov, D. B., Katushkina, O. A., et al. (2009). Kinetic-gasdynamic modeling of the heliospheric interface. Space Sci. Rev. 146 (1-4), 329–351. doi:10.1007/s11214-009-9528-3
Jokipii, J. R., and Giacalone, J. (1998). The theory of anomalous cosmic rays. Space Sci. Rev. 83, 123–136. doi:10.1023/a:1005077629875
Kivelson, M. G., and Russell, C. T. (1995). Introduction to space physics. Cambridge, United Kingdom: Cambridge University Press. doi:10.1017/9781139878296
Kleimann, J., Dialynas, K., Fraternale, F., Galli, A., Heerikhuisen, J., Izmodenov, V., et al. (2022). The structure of the large-scale heliosphere as seen by current models. Space Sci. Rev. 218 (4), 36. article id.36. doi:10.1007/s11214-022-00902-6
Kornbleuth, M., Opher, M., Baliukin, I., Gkioulidou, M., Richardson, J. D., Zank, G. P., et al. (2021). The development of a split-tail heliosphere and the role of non-ideal processes: A comparison of the BU and moscow models. Astrophysical J. 923179 (2), 179. doi:10.3847/1538-4357/ac2fa6
Korolkov, S., Izmodenov, V., and Alexashov, D. (2020). Numerical modeling of the convective Kelvin-Helmholtz instabilities of astropauses. J. Phys. Conf. Ser. 1640 (1), 012012. article id. doi:10.1088/1742-6596/1640/1/012012
Krimigis, S. M., Decker, R. B., Roelof, E. C., Hill, M. E., Armstrong, T. P., Gloeckler, G., et al. (2013). Search for the exit: Voyager 1 at heliosphere’s border with the galaxy. Science 341 (6142), 144–147. doi:10.1126/science.1235721
Krimigis, S. M., Decker, R. B., Roelof, E. C., Hill, M. E., Bostrom, C. O., Dialynas, K., et al. (2019). Energetic charged particle measurements from Voyager 2 at the heliopause and beyond. Nat. Astron. 3, 997–1006. doi:10.1038/s41550-019-0927-4
Kurth, W. S., and Gurnett, D. A. (2020). Observations of a radial density gradient in the very local interstellar medium by voyager 2. Astrophysical J. Lett. 900, L1. Number 1. doi:10.3847/2041-8213/abae58
Lavraud, B., Fedorov, A., Budnik, E., Thomsen, M. F., Grigoriev, A., Cargill, P. J., et al. (2005). High-altitude cusp flow dependence on IMF orientation: A 3-year cluster statistical study. J. Geophys. Res. Space Phys. 110, A02209. Issue A2CiteID A02209. doi:10.1029/2004JA010804
Lavraud, B., Jacquey, C., Achilli, T., Fuselier, S. A., Grigorenko, E., Phan, T. D., et al. (2018). Concomitant double ion and electron populations in the Earth's magnetopause boundary layers from double reconnection with lobe and closed field lines. J. Geophys. Res. Space Phys. 123, 5407–5419. doi:10.1029/2017JA025152
Lavraud, B., Thomsen, M. F., Lefebvre, B., Schwartz, S. J., Seki, K., Phan, T. D., et al. (2006). Evidence for newly closed magnetosheath field lines at the dayside magnetopause under northward IMF. J. Geophys. Res. Space Phys. 111. Issue A5CiteID A05211. doi:10.1029/2005JA011266
McComas, D., Funsten, H. O., Fuselier, S. A., Lewis, W. S., Mobius, E., and Schwadron, N. A. (2011). IBEX observations of heliospheric energetic neutral atoms: Current understanding and future directions. Geophys. Res. Lett. 38. doi:10.1029/2011GL048763
McComas, D. J., Allegrini, F., Bochsler, P., Bzowski, M., Christian, E. R., Crew, G. B., et al. (2009). Global observations of the interstellar interaction from the interstellar boundary explorer (IBEX). Science 326, 959–962. doi:10.1126/science.1180906
McComas, D., and Schwadron, N. (2014). Astrophysical J. Lett. 795 (1), L17. article id. L17. doi:10.1088/2041-8205/795/1/L17
McNutt, R. L., Wimmer-Schweingruber, R. F., Gruntman, M., Krimigis, S. M., Roelof, E. C., Brandt, P. C., et al. (2022). Interstellar probe – destination: Universe! Acta Astronaut. 196, 13–28. doi:10.1016/j.actaastro.2022.04.001
Mostafavi, P., Burlaga, L. F., Cairns, I. H., Fuselier, S. A., Fraternale, F., Gurnett, D. A., et al. (2022). Shocks in the very local interstellar medium. Space Sci. Rev. 218 (4), 27. article id.27. doi:10.1007/s11214-022-00893-4
Mostafavi, P., Zank, G. P., and Webb, G. M. (2017). Structure of energetic particle mediated shocks revisited. Astrophysical J. 841 (1), 4. article id. 4. doi:10.3847/1538-4357/aa6f10
Mostafavi, P., Zank, G. P., and Webb, G. M. (2018). The mediation of collisionless oblique magnetized shocks by energetic particles. Astrophysical J. 868 (2), 120. article id. 120. doi:10.3847/1538-4357/aaeb91
Øieroset, M., Phan, T. D., Angelopoulos, V., Eastwood, J. P., McFadden, J., Larson, D., et al. (2008). THEMIS multi-spacecraft observations of magnetosheath plasma penetration deep into the dayside low-latitude magnetosphere for northward and strong ByIMF. Geophys. Res. Lett. 35, L17S11. doi:10.1029/2008GL033661
Opher, M., and Drake, J. F. (2013). On the rotation of the magnetic field across the heliopause. Astrophysical J. Lett. 778 (2), L26. article id. L26. doi:10.1088/2041-8205/778/2/l26
Opher, M., Drake, J. F., Swisdak, M., Schoeffler, K. M., Richardson, J. D., Decker, R. B., et al. (2011). Is the magnetic field in the heliosheath laminar or A turbulent sea of bubbles? Astrophysical J. 734 (1), 10. article id. 71. doi:10.1088/0004-637X/734/1/71
Opher, M., Drake, J. F., Swisdak, M., Zieger, B., and Toth, G. (2017). The twist of the draped interstellar magnetic field ahead of the heliopause: A magnetic reconnection driven rotational discontinuity. Astrophysical J. Lett. 839 (1), L12. article id. L12. doi:10.3847/2041-8213/aa692f
Opher, M., Drake, J. F., Zank, G., Powell, E., Shelley, W., Kornbleuth, M., et al. (2021). A turbulent heliosheath driven by the Rayleigh–taylor instability. Astrophysical J. 922 (2), 181. doi:10.3847/1538-4357/ac2d2e
Pierrard, V., Botek, E., and Darrouzet, F. (2021). Improving predictions of the 3D dynamic model of the plasmasphere. Front. Astronomy Space Sci. 8, 69. doi:10.3389/fspas.2021.681401
Richardson, J. D., Belcher, J. W., Garcia-Galindo, P., and Burlaga, L. F. (2019). Voyager 2 plasma observations of the heliopause and interstellar medium. Nat. Astron. 3, 1019–1023. doi:10.1038/s41550-019-0929-2
Richardson, J. D., Burlaga, L. F., Elliott, H., Kurth, W. S., Liu, Y. D., and von Steiger, R. (2022). Observations of the outer heliosphere, heliosheath, and interstellar medium. Space Sci. Rev. 218 (4), 35. article id.35. doi:10.1007/s11214-022-00899-y
Richardson, J. D., Cummings, A. C., Burlaga, L. F., Giacalone, J., Opher, M., and Stone, E. C. (2021). Using magnetic flux conservation to determine heliosheath speeds. Astrophysical J. Lett., 919 (2), 6, doi:10.3847/2041-8213/ac27b1
Richardson, J. D., Kasper, J. C., Wang, C., Belcher, J. W., and Lazarus, A. J. (2008). Cool heliosheath plasma and deceleration of the upstream solar wind at the termination shock. Nature 454 (7200), 63–66. doi:10.1038/nature07024
Ruderman, M. S., and Fahr, H. J. (1993). The effect of magnetic fields on the macroscopic instability of the heliopause. 1. Parallel interstellar magnetic fields. Astronomy Astrophysics 275, 635.
Schwadron, N. A., Bzowski, M., Crew, G. B., Gruntman, M., Fahr, H., Fichtner, H., et al. (2009). Comparison of interstellar boundary explorer observations with 3D global heliospheric models. Science 326 (5955), 966–968. doi:10.1126/science.1180986
Schwadron, N. A., and McComas, D. J. (2013). Astrophysical J. Lett. 778 (2), 5. article id. L33. doi:10.1088/2041-8205/778/2/L33
Schwadron, N. A., and McComas, D. J. (2006). Particle acceleration at a blunt termination shock. AIP Conf. Proc. 858, 165–170. doi:10.1063/1.2359322
Stone, E. C., Cummings, A. C., Heikkila, B. C., and Lal, N. (2019). Cosmic ray measurements from Voyager 2 as it crossed into interstellar space. Nat. Astron. 3, 1013–1018. doi:10.1038/s41550-019-0928-3
Stone, E. C., Cummings, A. C., McDonald, F. B., Heikkila, B. C., Lal, N., and Webber, W. R. (2008). An asymmetric solar wind termination shock. Nature 454 (7200), 71–74. doi:10.1038/nature07022
Stone, E. C., Cummings, A. C., McDonald, F. B., Heikkila, B. C., Lal, N., and Webber, W. R. (2005). Voyager 1 explores the termination shock region and the heliosheath beyond. Science 309 (5743), 2017–2020. doi:10.1126/science.1117684
Stone, E. C., Cummings, A. C., McDonald, F. B., Heikkila, B. C., Lal, N., and Webber, W. R. (2013). Voyager 1 observes low-energy galactic cosmic rays in a region depleted of heliospheric ions. Science 341 (6142), 150–153. doi:10.1126/science.1236408
Strauss, R. D., Potgieter, M. S., Ferreira, S. E. S., and Hill, M. E. (2010). Modelling anomalous cosmic ray oxygen in the heliosheath. Astronomy Astrophysics 522, 8, doi:10.1051/0004-6361/201014528
Strumik, M., Grzedzielski, S., Czechowski, A., Macek, W. M., and Ratkiewicz, R. (2014). Advective transport of interstellar plasma into the heliosphere across the reconnecting heliopause. Astrophysical J. 782 (1), 5. article id. L7. doi:10.1088/2041-8205/782/1/L7
Swisdak, M., Drake, J. F., and Opher, M. (2013). A porous, layered heliopause. Astrophysical J. Lett. 774 (1), 5. article id. L8. doi:10.1088/2041-8205/774/1/L8
Swisdak, M., Drake, J. F., Opher, M., and Bibi, A. (2010). Astrophysical J. 710 (2), 1769–1775. doi:10.1088/0004-637x/710/2/1769
Webber, W. R., and McDonald, F. B. (2013). Recent Voyager 1 data indicate that on 25 August 2012 at a distance of 121.7 AU from the Sun, sudden and unprecedented intensity changes were observed in anomalous and galactic cosmic rays. Geophys. Res. Lett. 40 (9), 1665–1668. doi:10.1002/grl.50383
Zank, G. P. (2015). Faltering steps into the galaxy: The boundary regions of the heliosphere. Annu. Rev. Astronomy Astrophysics 53, 449–500. doi:10.1146/annurev-astro-082214-122254
Keywords: heliosphere, heliopause, heliosheath, solar wind, interstellar medium, magnetic reconnection, instrumentation
Citation: Lavraud B, Opher M, Dialynas K, Turner DL, Eriksson S, Provornikova E, Kornbleuth MZ, Mostafavi P, Fedorov A, Richardson JD, Fuselier SA, Drake J, Swisdak M, Eubanks M, Chen TY, Kucharek H, Kollmann P, Blanc M, André N, Génot V, Wimmer-Schweingruber RF, Barabash S, Brandt P and McNutt R (2023) What is the heliopause? Importance of magnetic reconnection and measurement requirements. Front. Astron. Space Sci. 10:1060618. doi: 10.3389/fspas.2023.1060618
Received: 03 October 2022; Accepted: 13 January 2023;
Published: 08 February 2023.
Edited by:
John C. Dorelli, National Aeronautics and Space Administration, United StatesReviewed by:
Mahboubeh Asgari-Targhi, Harvard University, United StatesCopyright © 2023 Lavraud, Opher, Dialynas, Turner, Eriksson, Provornikova, Kornbleuth, Mostafavi, Fedorov, Richardson, Fuselier, Drake, Swisdak, Eubanks, Chen, Kucharek, Kollmann, Blanc, André, Génot, Wimmer-Schweingruber, Barabash, Brandt and McNutt. This is an open-access article distributed under the terms of the Creative Commons Attribution License (CC BY). The use, distribution or reproduction in other forums is permitted, provided the original author(s) and the copyright owner(s) are credited and that the original publication in this journal is cited, in accordance with accepted academic practice. No use, distribution or reproduction is permitted which does not comply with these terms.
*Correspondence: B. Lavraud, YmVub2l0LmxhdnJhdWRAdS1ib3JkZWF1eC5mcg==
Disclaimer: All claims expressed in this article are solely those of the authors and do not necessarily represent those of their affiliated organizations, or those of the publisher, the editors and the reviewers. Any product that may be evaluated in this article or claim that may be made by its manufacturer is not guaranteed or endorsed by the publisher.
Research integrity at Frontiers
Learn more about the work of our research integrity team to safeguard the quality of each article we publish.