- 1Department of Earth, Environmental, and Planetary Sciences, Brown University, Providence, RI, United States
- 2In Orbit Aerospace, Torrance, CA, United States
- 3Sapienza University of Rome, Rome, Italy
- 4Department of Mechanical Engineering, Stanford University, Stanford, CA, United States
- 5Department of Computer Science, University of Oxford, Oxford, United Kingdom
- 6Chair of Space Systems, Technische Universität Dresden, Dresden, Germany
- 7Department of Mechanical Engineering, University of California, Berkeley, Berkeley, CA, United States
- 8Delft University of Technology, Delft, Netherlands
- 9Benchmark Space Systems, Burlington, VT, United States
- 10Department of Aerospace Engineering, Tohoku University, Sendai, Japan
- 11Lunar and Planetary Laboratory, University of Arizona, Tucson, AZ, United States
- 12Brevitē LLC, New York, NY, United States
Enceladus, with its subsurface ocean, is amongst the top priority targets in the search for life beyond Earth. Following on discoveries from the Cassini mission that Enceladus possesses a global subsurface ocean containing salt and organic compounds, there are many unconstrained properties of the ocean and ice shell that must be investigated to further assess the habitability of Enceladus and begin the search for biosignatures on Enceladus. In this paper, we present a concept study for a New Frontiers class multi-lander and orbiter mission to Enceladus that investigates if there is or ever was a habitable environment on Enceladus. The mission architecture includes an orbiter for detailed chemical analysis of material erupted from Enceladus’ plumes and four impact landers for geophysical measurements. As part of our mission concept study, we explore key trades for orbital and surface science, as well as assess the scientific potential and hazards of candidate landing sites on Enceladus. The novelty of our mission architecture and consideration of both orbital and surface science elements makes this work directly relevant to a broad range of potential future mission architectures under consideration, such as those identified in the 2023–2032 Planetary Science and Astrobiology Decadal Survey.
Introduction
Motivation and context
Enceladus is a small (∼250 km radius) yet geologically active world with exciting opportunities to assess habitability beyond Earth (Porco et al., 2006; Parkinson et al., 2008). Enceladus is a primary candidate in the search for extraterrestrial habitable environments because it contains the hallmarks of habitability for life as we know it: liquid water, common chemical building blocks (CHNOPS) for life, and available energy (e.g., chemical energy gradients; Cockell et al., 2016; Domagal-Goldman et al., 2016; Hand et al., 2020; Hendrix et al., 2019). Following on the Voyager 1 and 2 flybys, Cassini revealed that Enceladus possesses a global subsurface ocean under an ice shell that is 20–40 km thick on average (Postberg et al., 2009; Postberg et al., 2011; Beuthe et al., 2016; Thomas et al., 2016; Čadek et al., 2016), a remarkably young surface age (Porco et al., 2006), and cryovolcanic plumes that eject ocean material onto Enceladus’ surface and Saturn’s E ring (Porco et al., 2006; Spahn et al., 2006; Kempf et al., 2010; Porco et al., 2014). Ice grains erupted from Enceladus’ south polar plumes (both in the plumes at Enceladus and in Saturn’s E ring) analyzed by Cassini’s Ion and Neutral Mass Spectrometer (INMS) indicate the subsurface ocean contains salt, ammonia, and organics; this evidence suggests the ocean is in direct contact with the rocky interior of Enceladus (Waite et al., 2006; Postberg et al., 2008; Postberg et al., 2009; Hsu et al., 2015; Sekine et al., 2015; Postberg et al., 2018; Glein and Waite, 2020; Affholder et al., 2021). The plumes access Enceladus’ ocean through an ice shell <5 km thick from more than a hundred individual jets along four long (∼130 km) fractures known as the Tiger Stripes (Helfenstein and Porco, 2015; Čadek et al., 2016).
The Tiger Stripes have narrow openings (measured to be only ∼10 m across in some areas), but the subsurface geometry of the fractures’ connection to the ocean is still debated (Postberg et al., 2009; Goguen et al., 2013; Porco et al., 2014; Helfenstein and Porco, 2015; Ingersoll and Nakajima, 2016; Postberg et al., 2018). The area around the Tiger Stripes, the South Polar Terrain (SPT), is the youngest surface area on Enceladus (some areas <4 Ma old; Porco et al., 2006). It is not known whether particles falling from the plumes form an unconsolidated regolith or are thermally processed and/or sintered to form a solid ice layer (Porco et al., 2006; Kempf et al., 2010; Konstantinidis et al., 2015). More broadly, the generally ropy plains of the SPT are heavily fractured, may contain rapidly outgassing subsurface pockets of CO2, and are scattered with large ice blocks (∼10–100 m across) concentrated near the Tiger Stripes (Porco et al., 2006; Martens et al., 2015; Matson et al., 2018).
Beyond the SPT, surfaces tend to be older and more heavily cratered, though not lacking in extensional features (Kirchoff and Schenk, 2009; Hargitai and Kereszturi, 2015). Some parallel ridges and troughs in the northern hemisphere of Enceladus, such as Samarkand Sulci, may represent past active regions that may once have been similar to the present-day SPT (Porco et al., 2006; Crow-Willard and Pappalardo, 2015). A series of broad topographic basins, including the SPT basin, are found across the surface of Enceladus and may be related to past true polar wander of Enceladus’ ice shell over a heat source (Tajeddine et al., 2017). Enceladus’ cryovolcanic activity is modulated by its tidal cycle and may cease over longer timescales when fractures like the Tiger Stripes cannot penetrate to the ocean (e.g., Porco et al., 2014; Rudolph et al., 2022). At present, Enceladus’ ocean is not thermodynamically stable and should freeze over tens to hundreds of millions of years (e.g., Roberts and Nimmo, 2008; Rudolph et al., 2022). Thus, Enceladus may experience only transient habitable conditions. It is not known how long habitable conditions must persist for life to emerge (e.g., Cockell et al., 2016).
Here, we present a concept study for a New Frontiers class multi-lander and orbiter mission concept to Enceladus to investigate if there is or ever was a habitable environment on Enceladus. This mission concept study was developed during the 2019 Caltech Space Challenge, a 1-week program that brings together two teams of engineering and science undergraduate and graduate students to create a pre-Phase A concept study (Rabinovitch et al., 2014); the second team’s mission concept study can be found in Deutsch et al. (this issue). The 2019 Caltech Space Challenge charged participants to design a New Frontiers-class mission concept to assess whether Enceladus provides the conditions necessary (or sufficient) to sustain biotic or pre-biotic chemistry. Additional constraints provided by the challenge organizers were that the mission must make use of multiple small surface components, launch on a SLS-type vehicle, and arrive at Enceladus between 2036 and 2042. The culmination of the 2019 Caltech Space Challenge included the presentation of a final report, fact sheet, and public talk to a review panel (Caltech Space Challenge, 2019).
Though this mission concept study was carried out in advance of the 2023–2032 Planetary Science and Astrobiology Decadal Survey (NASEM, 2022), the SILENUS (Spectrometer Investigating the Livability of Enceladus with a Network Using Seismometers; Figure 1) mission concept is highly relevant to addressing the question of dynamic habitability outlined in the 2023–2032 Decadal Survey: “Where in the Solar System do potentially habitable environments exist, what processes led to their formation, and how do planetary environments and habitable conditions co-evolve over time?” The 2023–2032 Decadal Survey (NASEM, 2022) prioritized an Enceladus Orbilander concept as the second highest priority new Flagship mission for the decade owing to Enceladus’ favorability as a destination to address fundamental questions in habitability and life detection. Additionally, Enceladus is included in the list of targets in the Decadal Survey for New Frontiers 5, 6, and 7, which span the next decade of New Frontiers science.
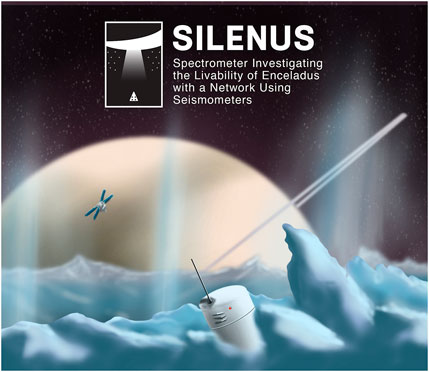
FIGURE 1. Logo for the SILENUS mission concept and artist sketch of a penetrator on the surface of Enceladus collecting seismic data as the orbiter soars overhead.
SILENUS in the context of mission concepts for Enceladus
Many mission concepts have been developed to study Enceladus, e.g., ELF (Lunine et al., 2015; Lunine et al., 2016), Enceladus Orbilander (MacKenzie et al., 2021), EnEx (Konstantinidis et al., 2015), Etna (Deutsch et al., this issue), JET (Lunine et al., 2016), THEO (MacKenzie et al., 2016), and TIGER (Spiers et al., 2021). Our mission concept is most similar to the Small Lander Large Orbiter explored as an alternative to the Flagship-class Enceladus Orbilander (MacKenzie et al., 2021). We note that another key difference between the SILENUS mission concept and the Enceladus Orbilander concept (MacKenzie et al., 2021) is that the Enceladus Orbilander is a life detection mission, whereas SILENUS aims to further characterize the habitability of Enceladus following on the discoveries made with Cassini.
A primary highlight of our architecture includes multiple penetrators to reduce the science risk of surface elements. Like the TandEM mission concept (Coustenis et al., 2009), SILENUS utilizes penetrators as opposed to traditional landers owing to the uncertainties of the surface terrain and strength on Enceladus, and our penetrators are equipped with seismometers. Seismometers have been explored for landed components of other Enceladus mission concepts (Coustenis et al., 2009; Deutsch et al., this issue; MacKenzie et al., 2021; Vance et al., 2021), but our mission concept study is among the first to incorporate a seismic network. Additionally, though other mission concepts propose to operate on the edge of south polar darkness (e.g., Spiers et al., 2021), SILENUS presents a case for science operations that continue during a time period when the South Polar Terrain (SPT) is in complete darkness. More broadly, SILENUS is applicable to future mission concept studies not only in its science definition, exploration of trades, and orbit design, but also in its detailed characterization of landing sites based on scientific value, surface hazards, and previous characterization.
Science definition
The primary mission of SILENUS is assessing and contextualizing the habitability of Enceladus. Rather than searching directly for signs of extant or extinct life, SILENUS would characterize physical and chemical environments on Enceladus to better understand whether life as we know it could emerge and survive there. This enables SILENUS to complete key science objectives in preparation for future life detection missions and, though not part of the mission requirements, make opportunistic measurements that could reveal evidence of extant or extinct life depending on its presence and abundance. Importantly, a habitability-focused mission provides the necessary context for either a detection or non-detection of life on Enceladus. If life is ever detected on Enceladus, it is important to study the markers of habitability associated with this life; on the other hand, if Enceladus is devoid of signs of extant or extinct life, then a detailed characterization of Enceladus’ habitability can transform our understanding of what is truly required for a world to support life (McKay et al., 2014; Neveu et al., 2018; Hendrix et al., 2019; Hand et al., 2020). We note that there is a lack of consensus as to whether the existing evidence provides a solid foundation for the habitability of Enceladus, or if there is further evidence needed to support this classification (e.g., Glein et al., 2015; Hendrix et al., 2019; Glein and Waite, 2020; Cable et al., 2021).
The main question guiding science definition for SILENUS, “Is or was there a habitable environment on Enceladus?”, is divided into three primary science objectives that frame the science traceability matrix (STM; Table 1):
Science Objective A. Characterize the organic chemistry of the plume ejecta.
Science Objective B. Characterize the inorganic chemistry of the plume ejecta.
Science Objective C. Determine the age, structure, and exchange pathways of habitable environments.
Though SILENUS is focused on assessing past and present habitability, the measurements outlined in the STM (Table 1) enable rich opportunities for synergistic science concerned with the geology and geophysics of Enceladus. In particular, the penetrators equipped with seismometers and context cameras offer opportunities, including to measure the level of seismicity on Enceladus, relationships between the seismicity and tidal cycle, material properties of the ice shell, and constraints on core shape and structure (Vance et al., 2018; Neumann and Kruse, 2019; Ermakov et al., 2021; Marusiak et al., 2021; Kisvárdai et al., 2022; Rovira-Navarro et al., 2022). Additionally, new imagery from the orbiter’s navigational camera and penetrators’ context imagers of Enceladus’ surface would enable higher resolution geologic mapping and opportunities to study the characteristics of the near-surface ice.
Landing site characterization
To achieve the penetrators’ science objectives, a consideration of landing sites is required. Unlike landing site consideration for a traditional lander (e.g., MacKenzie et al., 2021), the penetrators are more resilient to uncertainties in surface strength, but there are several important hazards and science potential to take into account when selecting landing sites.
In order to achieve the desired seismic measurements (Table 1), we propose to deploy three penetrators within the SPT and a fourth penetrator in the northern hemisphere of Enceladus. It is important to consider not only the network geometry, but also the surface conditions, as a high degree of mechanical coupling between the penetrator and surface is ideal for making seismic measurements. Additionally, as the penetrators are equipped with context cameras, there is greater science potential for sites with exposed stratigraphy and/or closest to the plumes (where the most material is falling onto the surface, likely preserving thicker regolith layers; Filacchione et al., 2016; Kempf et al., 2010). Beyond the Tiger Stripes, previously identified fractures (e.g., Samarkand Sulci) in the northern hemisphere are ideal targets for probing Enceladus’ potential past habitability.
However, there are numerous hazards, particularly in the SPT, that pose a risk to the penetrators:
Tiger Stripes and fractures: For both science operations and planetary protection, the Tiger Stripes crevasses are a significant hazard to be avoided. The interior of the Tiger Stripes descend into steep-sided valley floors (which could occlude communication between penetrators and the orbiter) with poorly characterized terrain; the conduit sources of the jets are likely only meters to tens of meters across (Goguen et al., 2013; Helfenstein and Porco, 2015). Additionally, the highest surface temperatures on Enceladus are found within the Tiger Stripes region (Goguen et al., 2013; Helfenstein and Porco, 2015; Filacchione et al., 2016). Numerous smaller fractures crosscut the Tiger Stripes and surrounding funiscular plains, as well as some regions outside the SPT (Porco et al., 2014; Helfenstein and Porco, 2015); these pose similar potential communication challenges for the penetrators.
Ice blocks: There are many irregular shaped boulders or ice blocks (∼10–100 m across) littered throughout the SPT. More than 100,000 of these blocks have been mapped and, though they are broadly most concentrated nearest the Tiger Stripes, the concentrations can vary drastically over small spatial scales (Martens et al., 2015). The ice blocks could potentially deflect the penetrator as it falls or partially block communication with the orbiter.
Pinnacles: Best characterized near Baghdad Sulcus, tall (∼100 m) pinnacles of ice sit atop ridges, with ∼100 m spacing between the pinnacles observed in Baghdad Sulcus (Helfenstein and Porco, 2015). The pinnacles could potentially deflect the penetrator. Similar features may also be present outside the SPT near Samarkand Sulci (Giese et al., 2017).
Subsurface CO2 pockets: Surface and near-surface pockets of solid and gaseous CO2 are another potential hazard found within the SPT (Matson et al., 2018). These pockets can outgas episodically, potentially dislodging a penetrator that lands nearby, and could also block communications between the penetrator and orbiter if a penetrator fell into one of these pockets.
For the point design we study herein, we select four specific landing locations along with backup sites (Figure 2). We select sites at such locations where we can make the best measurements to achieve the science objectives (Table 1), with the lowest hazard level from the combined list of potential hazards described above, and where we have the highest quality of existing imagery to characterize the site. In particular, the sites in the SPT were selected because they are within the best imaged areas of the SPT which have also been mapped as having relatively low concentrations of ice blocks. The specific hazards contributing most to the risk at each landing site are varied to minimize total risk and increase the likelihood of full returns on science objectives. The site near Samarkand Sulci was selected because it was the largest area of low hazard flat (at available resolution) terrain which was able to satisfy the requirements (specifically a seismometer separation of 100°) for the science objectives.
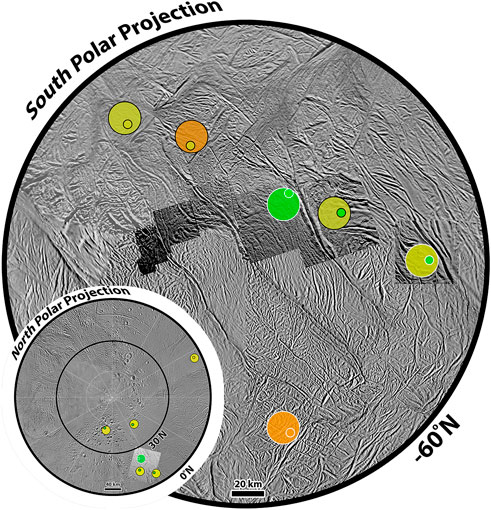
FIGURE 2. Ellipses represent selected landing sites (white outlines) and backups (black outlines) in Enceladus’ northern hemisphere (inset) and SPT categorized broadly by hazard levels (green- low, yellow- moderate, orange- high). Selected high resolution imagery is overlaid.
Science instrument payload
The proposed mission to Enceladus utilizes multiple instrument platforms to fulfill the science objectives (Table 1). While the mission architecture will be discussed in greater detail in subsequent sections, some overarching mission challenges are taken into consideration for the selection of the instrumentation:
Mission arrival between 2036 and 2042. The SPT region enters a period of ∼10 years of darkness at latitudes ≥70° during this time (e.g., Spiers et al., 2021). The majority of the potential launch windows considered will require landing in-situ instruments in darkness.
Unstable Polar Orbits. Orbital access to the SPT is not stable due to the perturbations of the Saturnian system. Polar orbits, which have a high science value, require larger ΔV for station keeping.
Unknown Terrain. Terrain mapping completed by Cassini only provides at best 10 m accuracy in the highest resolution coverage areas. Without an additional mission preceding SILENUS, detailed mapping could only be done upon arrival to Enceladus.
Extreme Environments. The mission design must survive several extreme environments. The large distance of Saturn from the Sun means solar power and heating are reduced; surface temperatures of Enceladus are of the order of 30–50 K (Filacchione et al., 2016), with areas near the plumes reaching up to 200 K (Abramov and Spencer, 2009; Goguen et al., 2013). Surface features at the SPT near the crevasses have significant ice boulders and variability in terrain (Martens et al., 2015), making landing dangerous.
Cost Limitations. Maintaining a budget below the $1B cost cap for the New Frontiers-class mission concept with the complex systems needed to operate in this environment will limit many options that can be considered.
Instrument trade
The first iteration of a design for this multi-platform concept begins with a qualitative, high-level trade study. This analysis focuses on the aforementioned design challenges along with initial estimations of risk, science merit, feasibility, and cost. The outcome is captured in the Key Trade Matrix (Table 2), which describes all prepared options and the amount of trade space available for each of the mission concept elements. We find that in-situ measurements are best addressed with a lander or penetrator concept and the ideal system architecture that we selected for SILENUS is a combined solution of an orbiter with penetrators.
Preferred instrumentation
To narrow the instrumentation search and guarantee an optimal mission architecture of valuable science return, the considered instruments in the Key Trade Matrix (Table 2) are mapped to the Science Traceability Matrix (STM) objectives (Table 1). The comparison is presented in Table 3, where a color code represents the science return of each instrument with respect to the associated science objective defined in Table 1.
Additional factors in preferred instrument selection are trades in the broader mission architecture. In particular, instruments have different potential to address science objectives depending on their allocation between the orbiter and penetrators. We explored three broad mission architecture cases (Table 4) based on the science returns for instruments identified in Table 3. Taking into account science return and risk allocation (i.e., the highest priority and majority of science objectives can be achieved by the lowest risk components of the mission architecture), we proceed with the instrument selection for the case of an orbital science-focused architecture.
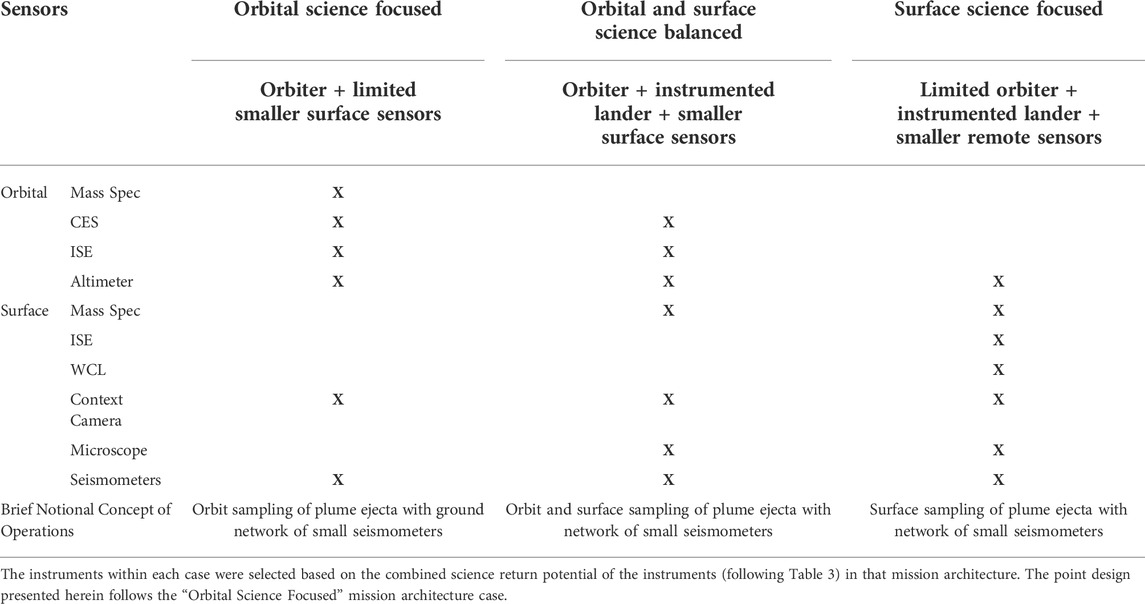
TABLE 4. A comparison of three instrument allocations considered for three cases of broader mission architecture.
The return matrix illustrates that the preferred set of instruments are seismometers, ion selective electrodes (ISE), capillary electrophoresis (CES), a wet chemistry lab (WCL), a mass spectrometer, and a laser altimeter. We note that the altimeter and ground penetrating radar offer similar contributions to science objectives, but an additional trade-off taking into account key mission concept parameters (mass, power, cost) found that an altimeter is preferable to ground penetrating radar (GPR) for the SILENUS mission concept.
For the penetrators, seismometers and context cameras were selected for the point design over alternatives such as drills and microscopic imagers (Tables 2, 3). This prioritization is motivated by the high level of science return likely to be achieved with the seismometers and context cameras as well as the challenges of using other instruments under the New Frontiers cost cap and/or the uncertainties in surface conditions at Enceladus.
Mission design
Launch trajectory and orbit design
The main maneuvers performed throughout the mission, along with a standard margin of 30%, leads to a final total consumption of ∼4900 m/s ΔV. This budget includes launch, a series of planetary gravity assists to shape the interplanetary trajectory to Saturn followed by a deceleration for Saturn Orbit Insertion (SOI). At this point, a subsequent burn allows for Enceladus Orbit Insertion (EOI), and then we account for station keeping maneuvers around Enceladus to assure successful science phase operations, and allowing for the spacecraft’s S/C final disposal.
The definition of a reasonable Earth-Saturn transfer orbit is fundamental to our design and follows a trade-off between launch date, consumption, and expected flight time. The Space Launch System (SLS) under development at NASA is considered, but alternatives such as the Space-X Falcon Heavy are ultimately selected as preferable for our point design given its high capacity at lower costs and a partially reusable configuration.
The work of Palma (2016) analyzed different trajectories to Saturn in 2020–2030, while assuming a flight time of ∼10 years. We select a nominal trajectory with a proposed launch date in April 2028 and 9.8 years interplanetary flight. With a total ΔV budget of 1330 m/s for both the interplanetary cruise and SOI, we proceed in budgeting with a more conservative estimation of 1500 m/s ΔV, which also allows increased resiliency to changes in launch date.
We evaluate several approaches for Enceladus Orbit Insertion (EOI), including aerobraking maneuvers at Titan, but ultimately select a more classical approach that makes use of several gravity assists of Saturn’s moons. Though we consider different tour budgets to EOI, we find that a 3-year tour allows us to lower ΔV consumption up to ∼1200 m/s. This 3-year tour would include several flybys of Saturn’s moons and opens the possibility of studying other moons for synergistic science and further estimating ephemerides of the Saturnian system.
After the EOI, at least ten passes through the plumes are necessary in order to fulfill the science requirements (Table 1). Ideally, the relative velocity between the spacecraft and plume particles should be less than 2 km/s (New et al., 2021). The velocity of the plume particles may be up to 1.2 km/s near where they exit the Tiger Stripes (Hansen et al., 2020), so the spacecraft must move slowly during these passes to avoid increasing its relative velocity. However, orbiting Enceladus is very challenging due to its low mass and proximity to Saturn, where a first approximation of its sphere of influence is around 490 km from Enceladus’ center of mass.
This Saturn-Enceladus close proximity requires consideration of a restricted 3-body problem (R3BP), where potential quasi-stable orbits belong to the Halo family. We select stable periodic Halo orbits close to Enceladus’ surface as reported in Massarweh and Cappuccio (2020), where a 5:14 resonance with a L1 orbital period of 11h46m is identified, thus leading to a minimum altitude over the mean radius (252.1 km) of ∼16.6 km (Figure 3). These orbits are not completely stable and, without intervention, could cause the spacecraft to impact the surface or fly away in only a few days due to higher harmonics effects. A ∼12-h period allows passing through the plumes twice per day, while maneuvers to control the spacecraft trajectory are needed to lower the risk of impact. It follows that a conservative 10 m/s correction per day over 10 days enables extra passes through the plumes (e.g., 20 extra passes). Overall, we allocate a total of 100 m/s for station keeping during the Halo orbit phase. During this low altitude campaign the spacecraft releases the penetrators to reach their target positions on the surface of Enceladus.
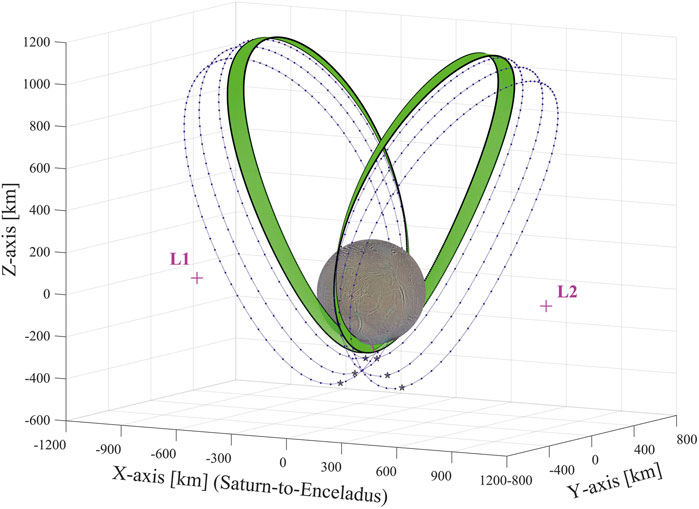
FIGURE 3. Some members of the periodic Halo orbit family (at Saturn Enceladus L1/L2 Lagrange points) near Enceladus are graphically shown in blue, along with their periapsis (gray stars). The range of zero-stable periodic orbits is highlighted in green color, following the linear stability results in Massarweh and Cappuccio (2020).
After this phase, a motion to a stable orbit at mid-inclination is needed to finish data collection from the penetrators (previously released to Enceladus’ surface) and to complete the science orbital phase. We select an orbit with an 8:35 resonance at mid-inclination (∼60°) and with semi-major axis of ∼500 km from the family of stable (quasi-periodic) orbits identified by Russel and Martin (2009); see Figure 4 and Table 2 therein). We ultimately estimate that the ΔV to move from the Halo orbit to the stable orbit would be less than 250 m/s.
Due to planetary protection constraints, the spacecraft must be deorbited away from Enceladus. Three different approaches are possible: 1) a plunge into Saturn, 2) crashing on another moon, or 3) an escape trajectory from the Saturn system. The first and third approaches do not contaminate a moon environment, but need a ΔV of several km/s. Hence, Tethys has been selected for a controlled crash due to its proximity and no relevant planetary protection limitations at the time of writing; the transfer orbit to impact on Tethys requires a ΔV of about 700 m/s.
Orbiter design
The orbiter design (Figure 4) builds on the heritage of past successful missions. A key design feature of SILENUS is the choice of basing the power system on solar panels, as have been selected for outer planets missions such as Juno and Europa Clipper, as well as explored in previous mission concept studies for Enceladus (e.g., Lunine et al., 2015; Lunine et al., 2016; MacKenzie et al., 2016). Thermal blankets consisting of amber multilayer insulation (MLI) cover the orbiter exterior as insulation to the extreme thermal fluctuations and to protect from small particles. The exterior mounting structure supports the 4-m high gain antenna and four deployable solar arrays. During the initial cruise, the high-gain antenna may point towards the Sun so its main reflector could serve as a sunshade and the low-gain antenna may be used for communications with Earth (Taylor et al., 2002). A surface-mounted dipole antenna would be used for communication between the penetrators and the orbiter. A surface-mounted camera for engineering and outreach would be included on one of the faces of the orbiter. The interior would house the onboard computer, propulsion fuel tanks, and payload instruments. The four penetrators are also mounted within the orbiter housing.
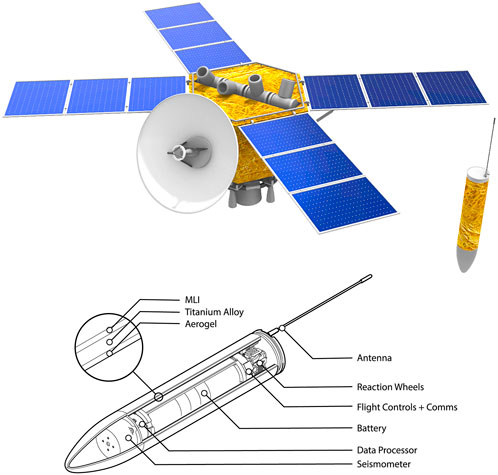
FIGURE 4. Upper: schematic CAD model of orbiter spacecraft and a penetrator. Lower: cross sectional view of penetrator.
Orbiter propulsion
We consider a trade-off between chemical, electrical, and nuclear propulsion to select the optimal propulsion technology for the orbiter. Although the specific impulse of a nuclear engine is twice that of one chemical propulsion engine, and more payload can be carried, nuclear thermal propulsion (NTP) has been excluded a priori due to its low technology readiness level (TRL) and the logistical challenges of utilizing this technology. Electric propulsion is in a phase of strong development, and great improvements are expected in the coming years. However, only a few interplanetary missions have used electrical propulsion (e.g., Dawn and BepiColombo). Electrical propulsion would therefore constitute a significant engineering challenge for the mission concept, adding complexity and risk. Chemical propulsion was chosen because it is a reliable, low-risk, and cheaper propulsion technology.
Due to the nature of the Enceladus science orbit under the influence of Saturn, active attitude control is necessary to satisfy the pointing requirements of the orbiter payload. This can be achieved through a combination of 16 cold-gas thrusters to achieve three-axis stabilization. The orbiter uses a series of star trackers, inertial measurement units, and sun sensors for attitude determination. Sun sensors are placed on all panels of the spacecraft, which allows the orbiter to determine the position of the solar vector and orient its solar arrays accordingly.
Two isolated propulsion systems were chosen for the orbiter:
(1) For the interplanetary trajectory to Enceladus, Enceladus’ orbit, performance and decommissioning, a bipropellant system based on Nitrogen Tetroxide (NTO)/Monomethylhydrazine (MMH) is chosen. The propulsion system is a main engine for propulsive maneuvers and a redundant main engine, both protected from micrometeoroid particles by an articulating cover. This type of engine is selected due to its reliable history of operations, high TRL, and high specific impulse.
(2) A monopropellant system is used for attitude control and small (<0.3 m/s) trajectory control maneuvers. The propellant chosen for the thruster is hydrazine (N2H4). Though toxic, hydrazine is well characterized when it combusts and easily detectable. Additionally, it could be used to clean and sterilize components. Sixteen redundant 1 N thrusters were selected, which have a strong flight heritage, having already flown onboard several space missions.
Orbiter power
Solar arrays
Powering the orbiter on a New Frontiers-class budget is a significant challenge, precluding the use of radioisotope thermoelectric generators (RTGs) for this mission concept. For this reason, we explore the viability of solar panels. At Saturn (9.5 AU from the Sun), the total solar irradiance is about 1.1% of the total solar irradiance at Earth.
We propose to use state of the art SolAero ZTJ Space Cell solar arrays (NASA JPL, 2017). The ZTJ cells are the baseline for the Europa Clipper mission, which would have flown by the time the SILENUS mission concept would propose to launch. SolAero has heritage in powering satellites and spacecraft for NASA and JPL, including the MAVEN, MMS, GPM, NuSTAR, SMAP, and LADEE missions.
The panels are sized based on peak power (all instruments operating and transponder at full power) of 288 W. A 30% margin is included, for a total power requirement of 370 W. Using the ZTJ cell specifications, the deployed solar array area required is estimated to be 134 m2 with a mass of 122 kg. This is a feasible size, and we expect these values to improve in future years with additional technology development. This calculation assumes that the solar panels are always on-angle. Furthermore, for pricing the solar panels we use historical data from JPL, and for prior analysis used a rough estimate of $1 M/kWh.
Note that solar panels need to be thermally controlled for peak efficiency and may need electrical heaters to compensate. Additional calculations must also account for solar panel decay due to radiation, temperature, and other degradation factors. Degradation and thermal power for the solar arrays are beyond the scope of this analysis, but a more detailed engineering analysis would be essential to future development of this mission concept.
Batteries
The primary batteries are sized to supply power when the orbiter is in eclipse or off-sun. The orbiter will be in darkness for at least 12 h every 33 h, and we estimate sizing the energy storage to 4 × 55 Ah Li-ion batteries.
A more detailed trade study for the secondary batteries is needed, which we propose to be conducted during Phase A of this mission concept. Currently, the Europa Lander concept has demonstrated Li-CFX chemistry batteries, which meet 500 Wh/kg power density within the 20-day mission.
Orbiter onboard data handling
The storage requirements are driven by the orbiter’s Enceladus flybys and the penetrator experiments. The onboard data handling and processing is extended by the use of low-powered field-programmable gate arrays (FPGAs) and space radiation-hardened integrated circuits for storage. The on-board computer (OBC) would be responsible for storing spacecraft data and payload instrumentation data. Additional functions of the OBC will include complex I/O management, fault tolerance and detection management, interfacing the ground to space link (telemetry and teleoperations), and interfacing on-board buses, data networks, and user interfaces for control and monitoring purposes.
The total data to downlink from the orbiter to Earth during every orbit around Enceladus is 202 kbits (Supplementary Table S1). The OBC will require a power of 45 W to process the data from the instruments and perform all other spacecraft operation tasks. Additionally, the FPGA must be radiation tolerant to moderate total ionizing doses of around 100 krad (Spencer and Niebur, 2010). Using the aforementioned sizing and information from other deep space missions, the derived cost for the data handling system is estimated at $300,000 (CPUStack, 2012).
Communications
The communication requirements for SILENUS include communication between the orbiter and Earth as well as communication between each of the penetrators and the orbiters (e.g., to uplink data collected from the seismometers). There is time specifically allocated in mission operations (see Section 4.4) for data to be transferred from the penetrators to the orbiter.
The selected communication architecture is radio communication with Deep Space Network (DSN) stations on Earth. The spacecraft would be commanded throughout the mission, providing link reliability via either a low-gain antenna or high-gain antenna (HGA) when Earth-pointed. The HGA is capable of communicating within the radio spectrum 2–35 GHz (S to Ka-band).
The telemetry communications of the mission concept are designed around the capability of X-band for command uplinks, engineering telemetry, and data downlinks. The onboard design would consist of a high-gain 4 m HGA, a 1 cm low-gain X-band antenna, and two X-band and two UHF/VHF band transponders. Our telecommunications downlink assessment assumed a 4 m HGA at X-band (single polarization), 50-W radiated power, and a DSN receiving array of two 70 m dishes.
The estimated downlink data rate is ∼40 kbps (before accounting for overhead or compression) for a duration of 6 h per day while in the stable orbit around Enceladus. The telecommunication design assumes that the orbiter would cease science operations during downlinks and point the body-fixed HGA within 0.05° of the ground station to transmit the stored data. During the science Halo-orbit, 6 h would be spent on a communication link with Earth. There would be no data exchange with the penetrators during Earth communication; the penetrators store all collected data on board.
Each of the penetrator systems can uplink data to the orbiter at a rate of 9.6 kbps using the UHF bandwidth while the orbiter is in the stable orbit over 5 days via two frequency bands. A relatively low-power antenna and transceiver are sufficient for the communication needs of the penetrator. A UHF/VHF band dipole antenna will be deployed after successful landing. The penetrator would use a commercial off-the-shelf (COTS) system. One way communication from the penetrator to the orbiter is sufficient for our purposes, although it is possible to support two-way communication with this configuration.
Penetrator design
We select a swarm-like lander concept to maximize science return within the New Frontiers budget cap. The orbiter would deploy four penetrators, each equipped with a seismometer and two context imagers, at predetermined landing sites. The seismometer requires a strong mechanical coupling with the substrate, which could be a challenge due to the highly uncertain terrain and surface properties. The penetrators land within a 20 km radius of target locations spread throughout the SPT and in the northern hemisphere (Figure 2) and operate for a minimum of 2 weeks for data collection.
The four penetrators are identical and their modularity simplifies manufacturing logistics. To comply with other design constraints, the penetrators have a bullet-shaped outer shell. The bullet-shaped structure will withstand the impact, even allowing for the highly uncertain characteristics of the surface. We estimate the mass of each penetrator to be 14 kg with volume <12 U.
The preferred total system architecture is a ballistically ejected penetrator with orientation control during descent and a radio relay using the orbiter. The penetrators would be deployed from the orbiter with no further trajectory control after deployment. Assuming the orbit velocity of 100 m/s and spacecraft mass of 25 kg, the impact energy is estimated at 0.14 MJ, so we select titanium alloy Ti-6Al-4V with a thickness of 5 mm for the outer shell of the penetrators. The metal structure complies with the impact conditions and facilitates the transfer of seismic signals from the surface to the seismometer housed within. Another engineering consideration is the temperature gradient between the penetrator material and the surface on impact, which could lead to brittle failure.
The proposed internal spacecraft temperature is 248 K (−25°C), which is a stark contrast to the lows of 33 K on the surface of Enceladus (Filacchione et al., 2016). While MLI systems insulate radiative energy, the direct contact and massive thermal gradient between the penetrator interior and Enceladus’ surface require special considerations. Conduction with the surface would quickly deplete the battery power and likely cause ice sublimation and thus penetrator sinking. We propose using a 1 mm thick layer of aerogel to insulate the interior of the penetrator. Aerogel is a unique material known for its very low density, and high thermal insulation (thermal conductivity of 0.03 W/mK), which has been used on the Sojourner Mars rover and Stardust mission (e.g., Aegerter et al., 2011). Heat flow paths and thermocouples will be used to control and monitor any thermal fluctuations.
Additional features, such as crumple zones, could absorb additional impact stresses and ensure safe operation at the surface, protecting both the instruments and aerogel insulation.
Attitude determination and control
We chose an orbiter ballistic ejection for the penetrator deorbit method, as a thrusted deorbit burn is unfeasibly complex for this mission concept. The penetrators will require active attitude determination and control following the ejection from the orbiter. Utilizing the orbit path of the orbiter and gravitational potential energy, the penetrators will have a controlled fall within a general landing area as specified by the science mission requirements prior to launch (Figure 2). The reaction wheels will stabilize the penetrators based on the measurements of the inertial measurement unit (IMU), which will be calibrated to the orbiter prior to launch, under the assumption that the orbiter is oriented parallel to the surface. The penetrator’s final landing area will be identified through the use of the laser altimeter and known location of the orbiter as it passes over the surface of Enceladus.
Other solutions we considered but did not ultimately select include an uncontrolled landing and orienting the seismometer to the ground after landing by using a gimbal/gyroscope-flywheel system. To circumvent the complexity of a controlled, multi-layer shell, we instead use cubesat reaction wheels. By ensuring a specific landing orientation, the penetrator will also be in the position allowing the antenna to communicate with the orbiter.
Penetrator power
Each penetrator requires a maximum power of 11 W, divided evenly between science instruments and heaters. A trade study among current rechargeable battery architectures has shown that Lithium-Ion (Li-Ion) batteries prevail over more advanced technologies such as Lithium-Sulfur (Li-S) batteries. While Li-S offers a better specific energy, the energy density is less than that of the Li-Ion counterpart. We assume the depth of discharge of these systems to be 70% and, for 2 weeks of power, battery mass is 6 kg and volume 9U.
Another important factor to consider for power supply is the performance decrease of the batteries, which is not included in the sizing estimates of the battery system. More advanced battery concepts, such as Li-CFx batteries (NASA, Technology Review: Jet Propulsion Lab, 2018) can cut the battery mass in half, due to their advanced specific energies, but are still size constrained. The choice of current battery systems is due to the cost consideration of the battery development. An alternative to batteries are radioisotope thermoelectric generators (RTGs), which are a more stable energy-source over long-duration missions but are much more expensive than battery systems. Additionally, radioisotopes raise concerns with the planetary protection of Enceladus. Augmenting the power input with solar panels is not viable for this specific mission concept, since the SPT region will be in complete darkness during the specified time period.
Onboard imaging suite
A context camera and photon source will be used to confirm penetrator release, landing, and penetration of the surface. Additional usages of the engineering camera include public outreach and science (e.g., stratigraphy imaging). Due to the illumination conditions at SILENUS’ landing time and location, a light source is required to illuminate the areas of interest (Table 1). We explore two photon source options. The Curiosity Mars rover’s camera MAHLI uses LED illumination. Currently, no LED technology is rated for Enceladus’ low surface temperatures, and adequate thermal insulation would need to be incorporated. As an alternative, we also consider the Surface Science Lamp (SSL), which was used onboard the Huygens lander at Titan.
Penetrator onboard data handling
The penetrators are required to store context images and seismic data. The penetrators will have a mission lifetime of 3 weeks. For this duration, the seismometers will be continuously measuring for 2 weeks, followed by a week allocated for data uplink. During the data collection period, the seismometers generate 12,000 bit/s, resulting in a total data generation of approximately 1.8 Gb over 2 weeks.
The required science context imagery at the point of impact totals 15 images at 21 MB each, resulting in a total image data generation of ∼315 MB. Due to the lack of visibility that the orbiter experiences while in the science orbit flying through the plumes, the penetrators will be required to have the capability to store the entirety of the context imagery and 2 weeks of seismometer measurements. Therefore, the onboard computer will need to be able to store at least 2.11 GB. The chosen equipment for our point design to satisfy the subsystem’s needs is the GOMSpace NanoMind A3200, which provides 512 KB built-in flash, 128 MB NOR flash, and 32 MB of SDRAM. This is a COTS component from GOMSpace, utilized for cube, nano, and microsat missions. The component would need to be radiation-hardened and protected to endure deep space.
Mission operations
The mission concept begins with a gravity assist Earth-Venus-Earth-Earth (EVEE) maneuver to reach Saturn, totaling 10 years (Figure 5A). Once the Saturn Orbital Insertion (SOI) is completed, the orbiter would begin a spiral down phase for ∼2.5 years to enter a polar Halo orbit around Enceladus. After Enceladus Orbital Insertion (EOI), plume sampling and altimeter mapping begins during the low pass of the polar Halo over SPT at altitudes below 20 km. Primary orbiter science continues until the minimum plume sampling and mapping requirements (Table 1) are achieved for a duration of 10 days. Once plume sampling and mapping requirements are met (nominally after 8 days), the orbiter sequentially deploys three penetrators in the SPT region over several passes. Then, the orbiter exits the polar Halo and enters a stable quasi-periodic orbit around Enceladus, deploying the final seismometer in the new periodic orbit away from the SPT (Figure 2). Penetrators begin science data collection immediately. Seismic data would be gathered from the surface, stored, and transmitted to the orbiter after 2 weeks. Data from the penetrators would be gathered by the orbiter until the seismic data collection window is complete (Figure 5B). The data will be cached and transferred from the orbiter via the Deep Space Network (DSN) to Earth, and then to the Spacecraft Operations Center (SOC). From the SOC, all science data will be processed and remote commands can be sent. The orbiter would continue mapping operations in the new orbit for 1 year. Once all data is acquired from the penetrator, the mission would be deemed complete (Figure 5A) with the option to extend mapping operations or secondary science. Finally, the spacecraft would perform a controlled crash on Tethys, satisfying planetary protection requirements (see Section 5).
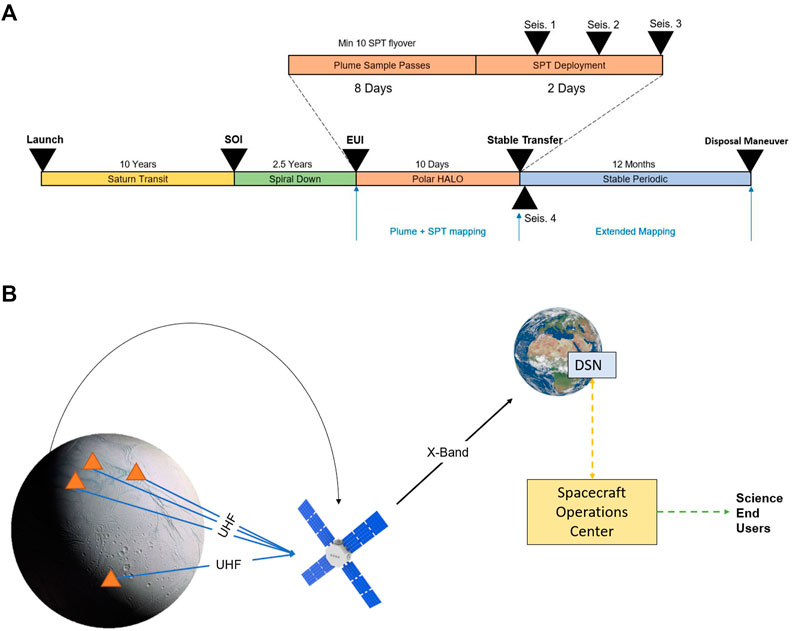
FIGURE 5. (A) Mission concept of operations timeline. (B) Communications flow, where triangles represent penetrators.
Ground operations
SILENUS would use NASA’s DSN, where coordination and operational management is accomplished through the Deep Space Operations Center (DSOC) located at the Jet Propulsion Laboratory (JPL).
The telecommunications system on-board will be operating on two bands (X and Ka) such that the uplink frequency and gain is supported by two types of DSN antennae. X-band is primarily utilized for spacecraft operations and navigation. For this, we need to employ a 70-m dish and currently there are three locations with IDs (DSS 14, DSS 43, DSS 63) that satisfy the gain requirement. The reception on the ground will be less frequent than between the orbiter and penetrators. This would be carried on the Ka-band with a similar transmitting antenna gain, which requires a 34 m Beam Waveguide (BWG) antenna on ground. Once again, we have multiple options in all three locations for this band in downlink, however only one DSS 25 in Goldstone, California has uplink frequency capability in Ka-band.
Cost budget and mission cost analysis
The mission concept is designed as a pre-Phase A concept study under the New Frontiers class, which aims to conduct high-science-return investigations while containing total mission cost and development time. We improve the performance of our mission concept through the use of validated new technologies and control of design, development, and operation costs while maintaining a strong commitment to flight safety. Excluding the cost of the launch vehicle and costs accumulated in Phases E-F (Operations and Sustainment, Closeout), our mission concept is budgeted for $1 billion Fiscal Year 16 (FY16) dollars (Table 5).
For this budget, the inputs for phases A to D (Concept and Technology Development, Preliminary Design and Technology Completion, Final Design and Fabrication, and System Assembly, Integration & Test, Launch & Checkout) are calculated for a Outer Planet Multi-FE type mission with 30% reserves. Using guidelines and metrics for assessing space system cost estimates (Supplementary Table S2), we note the dry weight of the orbiter to be 890 kg. This ensures that we accurately scale flight system costs by weight and mission type. The mission concept classification is Science/Surveillance with key characteristics of scientific observation, large spacecraft, multiple payloads, low heritage, and long life.
Engineering budgets
In addition to cost, we scrutinize other critical budgets to assess feasibility of this mission concept. The mass, power, and link budget tables can be found in the supplements (Table 6 and Supplementary Tables S3–S5), but we highlight the rationale and most significant contributors here.
The peak power requirements from major subsystems of the orbiter are listed in Supplementary Table S3. This informs the power budget, used to size the solar arrays, with the largest contributor being the communications system.
The link uplink and downlink budgets are found in Supplementary Tables S4, S5, respectively. This demonstrates the interaction between the orbiter around Enceladus and the Earth ground station. The communication architecture is developed from the Cassini heritage (Spencer and Niebur, 2010) and has sufficient telemetry margins to meet the proposed science objectives.
The preliminary mass budget was estimated through first order approximation (Table 6; Larson et al., 1999). The total mass percentage allocations (Table 6) allows us to size each subsystem, for instance, a communication (TTC) subsystem mass of around 120 kg. Such first-order estimation of the total dry-mass orbiter would be 929 kg; this value is then used to size the remaining (unknown) subsystems. For example, in the Cassini architecture, the orbiter’s final total mass is around 889 kg, thus referenced in our calculations.
Mission safety
There are several common practices and considerations needed for a successful interplanetary mission: radiation, electromagnetic interference (EMI), contamination control, and planetary protection. These factors are relevant for the orbiter spacecraft and the penetrators.
The spacecraft would be subjected to several levels and types of radiation throughout the mission. Radiation can impact the performance of electronic parts, causing failures, corrupting or even completely destroying data. Radiation can also degrade the quality of the solar panels, especially when passing through high-radiation areas. In order to mitigate radiation damage, the spacecraft can be designed with radiation-hardened electronic hardware. Similarly, a structural design can be selected to shield the critical electronics. Once in the Saturnian system, radiation from the solar wind is negligible and Enceladus is on the outskirts of the Saturnian radiation belt.
EMI generated by multiple electronic systems in the spacecraft can disrupt the operation and impact mission success. The EMI mitigation strategy is to carefully plan and test both individual components as well as the system. A complete mission safety plan would be completed during Phase A, with regular safety reviews conducted for all subsequent phases.
Certain subsystems have the potential to contaminate the spacecraft and therefore jeopardize the mission. Outgassing of materials, or deposits from thruster ejecta can impact and disrupt other spacecraft operations. Thruster plume analysis via computational fluid dynamics and thruster testing can characterize and mitigate the deposition of any propellant particles on the spacecraft. Careful material selection can minimize outgassing.
Planetary protection
Planetary protection includes preventing 1) the contamination of planetary bodies outside of Earth and 2) the contamination of Earth from exo-Earth materials (NASA Planetary Protection Center of Excellence, 2018). Planetary protection is salient when exploring moons with liquid water, especially when investigating the habitability of such moons.
Following Cassini’s discovery of Enceladus’s plumes, the Committee on Space Research (COSPAR) Planetary Protection Policy (COSPAR, 2021) was amended to add Enceladus to the list of target bodies for planetary protection classification Category III/IV, and NASA’s planetary protection policy (NPR 8020.12D, Planetary Protection Provisions for Robotic Extraterrestrial Mission) was also updated as such. Because the Enceladus system has been classified as a Category IV, missions to Enceladus require a crafted Planetary Protection Plan, a Pre-Launch Report, Post-Launch Report, End-of-Mission Report, and a Planetary Protection Implementation Plan. This plan details the use of clean rooms during assembly and test of spacecraft and microbial reduction measures, such as increased measures to reduce bioburden and prevent re-contamination by using bio-barriers. If the probability of impact is considered significant, an inventory of bulk constituent organics is required.
Furthermore, “the probability of inadvertent contamination of an ocean or other liquid water body” must be reduced to less than 1 × 10–4 per mission (NPR 8020.12D, Section 5). As the penetrators could directly contact the liquid body of water in a vent-conduit system, significantly more elaborate bioburden reduction processing would be necessary. A similar level of bioburden reduction processing would be necessary for the orbiter as well because penetrators would be in contact with the orbiter over a long period. To reduce contamination probability, it is worth considering more stringent sterilization, such as heat sterilization at 500°C for extended durations, to pyrolyze any organic material, decomposing all large organic molecules (Wilcox et al., 2017).
At the end of the mission, the orbiter would be destroyed to avoid any contact with Enceladus or other regions of interest (see Section 4.1.4). The penetrators will be sealed shut and sterilized appropriately since they would remain on the surface of Enceladus following the end of the mission.
Discussion of risks and trades
A key factor motivating the high level design of this mission concept is the strategic allocation of risk. Here, we consider risks to both achievement of science goals and technical requirements for mission success. The selected mission architecture places the instruments with the highest priority science observations in the orbiter, which is lower risk. Surface measurements are accomplished by the higher risk penetrators. Risk analysis is conducted to assess how instrument loss would affect the science return of the proposed mission concept (Table 7). Instead of using the NASA risk management metric (NASA, 2017), we use a simplified risk assessment, with 0 representing high risk, 1 representing moderate risk, and 2 representing low to no risk. The weighting of the science objectives is according to the science definition in Section 2.
The mission and engineering risk score (Table 8) is calculated by multiplying the likelihood score (1–5) by the impact score (1–5). The likelihood factor was determined through discussions with engineering and science team members based on the complexity and frequency for which a scenario could occur during the mission. The impact factor is determined by assuming the scenario occurred and assessing the influence of the event on mission success if no intervention were possible. We additionally assess the technology readiness level (TRL) of all science instruments and engineering systems (Supplementary Table S6). Note that the penetrator systems have the lowest TRL and would require the most development and testing.
Conclusion
We have presented a New Frontiers-class mission concept for an orbiter with multiple surface penetrators to assess the past and present habitability of Enceladus. The mission concept outlined achieves science objectives critical for understanding the potential habitability of Enceladus via determining the inventory of organic carbon-bearing compounds and whether or not these molecules are biogenic, constraining the environmental conditions of the ocean, and studying current and past geological activity. In particular, we outlined three key science objectives: A. Characterize the organic chemistry of the plume ejecta, B. Characterize the inorganic chemistry of the plume ejecta, and C. Constrain the age, structure, and exchange pathways of habitable environments. Investigating these specific aspects of Enceladus targets the existing gaps in our knowledge of Enceladus’ habitability, including ocean pH and the longevity of these potentially habitable environments (e.g., Hendrix et al., 2019).
The investigations we propose with SILENUS would directly answer priority science questions outlined in both the 2013–2022 and 2023–2032 Planetary Science and Astrobiology Decadal Surveys (National Research Council, 2011; NASEM, 2022). SILENUS is highly informative for future mission concept development for Enceladus, which is listed as a priority destination for the upcoming decade at both the New Frontiers and Flagship level. In particular, the inclusion of multiple surface elements makes the SILENUS design more robust to the challenges presented by the uncertain and hazardous terrains present on Enceladus, and leaves the most crucial science requirements to be achieved by the lower risk orbiter. Additionally, SILENUS provides a case study for science operations that can continue during the years of darkness over the SPT (such as during 2039–2054). Other science and engineering trades (e.g., orbit design, instrumentation allocation between orbiter and penetrators) explored herein, as well as the considerations for landing site selection, have broad applicability for the planning of future missions to this exciting ocean world.
Data availability statement
The original contributions presented in the study are included in the article/Supplementary Material, further inquiries can be directed to the corresponding author.
Author contributions
EN: conceptualization, data curation, formal analysis, investigation, methodology, project administration, supervision, validation, visualization, writing (original draft), writing (review and editing). KB, PC, JD, KD, AG, ML, LM, TP, SS: conceptualization, data curation, formal analysis, investigation, methodology, validation, visualization, writing (original draft), writing (review and editing). MR-N: conceptualization, data curation, formal analysis, investigation, methodology, validation, writing (review and editing). DL: conceptualization, visualization, writing (review and editing).
Funding
The initial work for this manuscript was conducted at the 2019 Caltech Space Challenge, sponsored by the Aerospace Corporation, the Guggenheim Aeronautical Laboratory at Caltech, the Jet Propulsion Laboratory, Keck Institute for Space Studies, Lockheed Martin Corporation, the Moore-Hufstedler Fund for Student Life at Caltech, and the Northrop Grumman Corporation. The funding for publication fees was provided by the Caltech Space Challenge.
Acknowledgments
The authors acknowledge the contributions of the additional members of Team Voyager in the 2019 Caltech Space Challenge for their contributions to the development of the initial mission concept: Jared Fuchs, Rebecca Jolitz, Atila Meszaros, Daniel Naftalovich. The authors wish to express their gratitude to Fabien Royer and Simon Toedtli for organizing the 2019 Caltech Space Challenge. The authors also thank team mentors, including Damon Landau, Florian Kehl, Hayden Burgoyne, Ilana Gat, Jason Rabinovich, Kristina Hogstrom, Kristjan Stone, Nigel Angold, and Thibaud Talon, as well as guest speakers David Murrow, Jaakko Karras, Jean-Pierre de la Croix, Kalind Carpenter, Morgan Cable, Randii Wessen, Steve Matousek, Thomas Heinsheimer, and Tom Nordheim for their insights and expertise during the 2019 Caltech Space Challenge. The authors also wish to express gratitude to the participants of Team Explorer: Emiliano Castillo Specia, Yun-Hang Cho, Victoria Da Poian, Ariel Deutsch, Graciela González Peytaví, Andrea Guariello, Onalli Gunasekara, Lewis Jones, Mariya Krasteva, Thasshwin Mathanlal, Paolo Panicucci, Jennifer Pouplin, Laura Tenelanda, Chandrakanth Venigalla, Nicole Villanueva, and Sam Zaref. The authors thank the following corporate and institute sponsors of the 2019 Caltech Space Challenge: Lockheed Martin Corporation, Keck Institute for Space Studies, Northrop Grumman Corporation, Aerospace Corporation, Jet Propulsion Laboratory, Guggenheim Aeronautical Laboratory at Caltech, and the Moore-Hufstedler Fund for Student Life at Caltech.
Conflict of interest
Author KB is employed by In Orbit Aerospace. Author TP is employed by Benchmark Space Systems. Author DL is employed by Brevitē LLC. All authors declare no other competing interests.
The remaining authors declare that the research was conducted in the absence of any commercial or financial relationships that could be construed as a potential conflict of interest.
Publisher’s note
All claims expressed in this article are solely those of the authors and do not necessarily represent those of their affiliated organizations, or those of the publisher, the editors and the reviewers. Any product that may be evaluated in this article, or claim that may be made by its manufacturer, is not guaranteed or endorsed by the publisher.
Supplementary material
The Supplementary Material for this article can be found online at: https://www.frontiersin.org/articles/10.3389/fspas.2022.995941/full#supplementary-material
References
Abramov, O., and Spencer, J. R. (2009). Endogenic heat from Enceladus’ south polar fractures: New observations, and models of conductive surface heating. Icarus 199 (1), 189–196. doi:10.1016/j.icarus.2008.07.016
Aegerter, M., Leventis, N., and Koebel, M. (2011). in Aerogels handbook. Editors M. A. Aegerter, N. Leventis, and M. M. Koebel (New York, NY: SpringerSpringer New York). doi:10.1007/978-1-4419-7589-8
Affholder, A., Guyot, F., Sauterey, B., Ferrière, R., and Mazevet, S. (2021). Bayesian analysis of Enceladus’s plume data to assess methanogenesis. Nat. Astron. 5, 805–814. doi:10.1038/s41550-021-01372-6
Beuthe, M., Rivoldini, A., and Trinh, A. (2016). Enceladus’s and Dione’s floating ice shells supported by minimum stress isostasy. Geophys. Res. Lett. 43 (10), 10,088–110,096. doi:10.1002/2016GL070650
Cable, M. L., Porco, C., Glein, C. R., German, C. R., MacKenzie, S. M., Neveu, M., et al. (2021). The science case for a return to Enceladus. Planet. Sci. J. 2 (132). doi:10.3847/psj/abfb7a
Čadek, O., Tobie, G., Van Hoolst, T., Massé, M., Choblet, G., Lefèvre, A., et al. (2016). Enceladus’s internal ocean and ice shell constrained from Cassini gravity, shape, and libration data. Geophys. Res. Lett. 43, 5653–5660. doi:10.1002/2016GL068634
Caltech Space Challenge (2019). Caltech space challenge. Final Presentation Team Voyager.” YouTube, uploaded by Caltech Space Challenge https://youtu.be/xIUK31lAhE8.
Cockell, C. S., Bush, T., Bryce, C., Direito, S., Fox-Powell, M., Harrison, J. P., et al. (2016). Habitability: A review. Astrobiology 16, 89–117. doi:10.1089/ast.2015.1295
Committee on Space Research (2021). COSPAR policy on planetary protection. https://cosparhq.cnes.fr/scientific-structure/panels/panel-on-planetary-protection-ppp/.
Coustenis, A., Atreya, S. K., Balint, T., Brown, R. H., Dougherty, M. K., Ferri, F., et al. (2009). TandEM: Titan and Enceladus mission. Exp. Astron. (Dordr). 23 (3), 893–946. doi:10.1007/s10686-008-9103-z
CPUStack, (2012). The CPUs of spacecraft computers in space. https://www.cpushack.com/space-craft-cpu.html.
Crow-Willard, E. N., and Pappalardo, R. T. (2015). Structural mapping of Enceladus and implications for formation of tectonized regions. J. Geophys. Res. Planets 120, 928–950. doi:10.1002/2015JE004818
Deutsch, A. N., Panicucci, P., Tenelanda-Osorio, L. I., Da Poian, V., Cho, Y. H., Venigalla, C., et al. (this issue). The Etna mission concept to Enceladus: Assessing the habitability of an active ocean world. Submitted to Frontiers in Astronomy and Space Sciences.
Domagal-Goldman, S. D., Wright, K. E., Adamala, K., De La Rubia, L. A., Bond, J., Dartnell, L. R., et al. (2016). The astrobiology primer v2.0. Astrobiology 16 (8), 561–653. doi:10.1089/ast.2015.1460
Ermakov, A. I., Park, R. S., Roa, J., Castillo-Rogez, J. C., Keane, J. T., Nimmo, F., et al. (2021). A recipe for the geophysical exploration of Enceladus. Planet. Sci. J. 2 (4), 157. doi:10.3847/PSJ/ac06d2
Filacchione, G., D’Aversa, E., Capaccioni, F., Clark, R. N., Cruikshank, D. P., Ciarniello, M., et al. (2016). Saturn’s icy satellites investigated by Cassini-VIMS. IV. Daytime temperature maps. Icarus 271, 292–313. doi:10.1016/J.ICARUS.2016.02.019
Giese, B., Helfenstein, P., Hauber, E., Hussmann, H., and Wagner, R. (2017). An exceptionally high standing ridge on Enceladus. Eur. Planet. Sci. Congr. Abstr. 11.
Glein, C. R., Baross, J. A., and Waite, J. H. (2015). The pH of Enceladus’ ocean. Geochimica Cosmochimica Acta 162, 202–219. doi:10.1016/J.GCA.2015.04.017
Glein, C. R., and Waite, J. H. (2020). The carbonate geochemistry of Enceladus’ ocean. Geophys. Res. Lett. 47 (3). doi:10.1029/2019GL085885
Goguen, J. D., Buratti, B. J., Brown, R. H., Clark, R. N., Nicholson, P. D., Hedman, M. M., et al. (2013). The temperature and width of an active fissure on Enceladus measured with Cassini VIMS during the 14 April 2012 South Pole flyover. Icarus 226, 1128–1137. doi:10.1016/j.icarus.2013.07.012
Hand, K. P., Sotin, C., Hayes, A., and Coustenis, A. (2020). On the habitability and future exploration of ocean worlds. Space Sci. Rev. 216, 95. doi:10.1007/s11214-020-00713-7
Hansen, C. J., Esposito, L., Colwell, J., Hendrix, A., Portyankina, G., Stewart, A., et al. (2020). The composition and structure of Enceladus' plume from the complete set of Cassini UVIS occultation observations. Icarus 344, 113461. doi:10.1016/j.icarus.2019.113461
Hargitai, H., and Kereszturi, Á. (2015). in Encyclopedia of planetary landforms. Editors Henrik Hargitai, and Á. Kereszturi (New York, NY: Springer New York). doi:10.1007/978-1-4614-3134-3
Helfenstein, P., and Porco, C. C. (2015). Enceladus’ geysers: Relation to geological features. Astron. J. 150 (3), 96. doi:10.1088/0004-6256/150/3/96
Hendrix, A. R., Hurford, T. A., Barge, L. M., Bland, M. T., Bowman, J. S., Brinckerhoff, W., et al. (2019). The NASA roadmap to ocean worlds. Astrobiology 19 (1), 1–27. doi:10.1089/ast.2018.1955
Hsu, H. W., Postberg, F., Sekine, Y., Shibuya, T., Kempf, S., Horányi, M., et al. (2015). Ongoing hydrothermal activities within Enceladus. Nature 519 (7542), 207–210. doi:10.1038/nature14262
Ingersoll, A. P., and Nakajima, M. (2016). Controlled boiling on Enceladus. 2. Model of the liquid-filled cracks. Icarus 272, 319–326. doi:10.1016/j.icarus.2015.12.040
John, K. K., Wynne, J. J., Powell, K. E., MacKenzie, S. M., Caswell, T. E., Stavros, E. N., et al. (2016). THEO mission concept: Testing the habitability of Enceladus’s ocean. Lunar Planet. Sci. Conf. 47. abstract #1277.
Kempf, S., Beckmann, U., and Schmidt, J. (2010). How the Enceladus dust plume feeds Saturn’s E ring. Icarus 206 (2), 446–457. doi:10.1016/j.icarus.2009.09.016
Kirchoff, M. R., and Schenk, P. (2009). Crater modification and geologic activity in Enceladus’ heavily cratered plains: Evidence from the impact crater distribution. Icarus 202 (2), 656–668. doi:10.1016/j.icarus.2009.03.034
Kisvárdai, I., Pál, B. D., and Kereszturi, Á. (2022). Investigating pore compaction depth and the habitability potential of icy moons. Lunar Planet. Sci. Conf. 53, 2082.
Konstantinidis, K., Flores Martinez, C. L., Dachwald, B., Ohndorf, A., Dykta, P., Bowitz, P., et al. (2015). A lander mission to probe subglacial water on Saturn׳s moon Enceladus for life. Acta Astronaut. 106, 63–89. doi:10.1016/j.actaastro.2014.09.012
Larson, W. J., United, S. A. F. A., Wertz, J. R., and Microcosm, I. (1999). in Space mission analysis and design. Editors D. Kirkpatrick, and D. Klungle. 3rd ed. (El Segundo: Microcosm Press, Kluwer Academic Publishers).
Lunine, J. I., Tobie, G., Mitri, G., Tosi, F., and Coustenis, A. (2016). Future exploration of Enceladus and Saturn’s icy moons. Enceladus Icy Moons Saturn, 3023. doi:10.1002/2015GL063384
Lunine, J. I., Waite, J. H., Postberg, F., Spilker, L., and Clark, K. (2015). Enceladus life finder: The search for life in a habitable moon. Lunar Planet. Sci. Conf. 46, 1525.
MacKenzie, S. M., Caswell, T. E., Phillips-Lander, C. M., Stavros, E. N., Hofgartner, J. D., Sun, V. Z., et al. (2016). THEO concept mission: Testing the habitability of Enceladus’s ocean. Adv. Space Res. 58 (6), 1117–1137. doi:10.1016/j.asr.2016.05.037
MacKenzie, S. M., Neveu, M., Davila, A. F., Lunine, J. I., Craft, K. L., Cable, M. L., et al. (2021). The Enceladus orbilander mission concept : Balancing return and resources in the search for life. Planet. Sci. J. 2 (2), 77. doi:10.3847/PSJ/abe4da
Martens, H. R., Ingersoll, A. P., Ewald, S. P., Helfenstein, P., and Giese, B. (2015). Spatial distribution of ice blocks on Enceladus and implications for their origin and emplacement. Icarus 245, 162–176. doi:10.1016/J.ICARUS.2014.09.035
Marusiak, A. G., Vance, S., Panning, M. P., Běhounková, M., Byrne, P. K., Choblet, G., et al. (2021). Exploration of icy ocean worlds using geophysical approaches. Planet. Sci. J. 2, 150. doi:10.3847/PSJ/ac1272
Massarweh, L., and Cappuccio, P. (2020). On the restricted 3-body problem for the saturn-enceladus system: Mission geometry & orbit design for plume sampling missions. AIAA 2020-0467. Orlando, FL: AIAA Scitech 2020 Forum.
Matson, D. L., Davies, A. G., Johnson, T. V., Combe, J.-P., McCord, T. B., Radebaugh, J., et al. (2018). Enceladus’ near-surface CO2 gas pockets and surface frost deposits. Icarus 302, 18–26. doi:10.1016/J.ICARUS.2017.10.025
McKay, C. P., Anbar, A. D., Porco, C. C., and Tsou, P. (2014). Follow the plume: The habitability of Enceladus. Astrobiology 14 (4), 352–355. doi:10.1089/ast.2014.1158
National Academies of Sciences (2022). Origins, worlds, and life: A decadal strategy for planetary science and astrobiology 2023-2032. Washington, D.C.: National Academies Press. doi:10.17226/26522Engineering, and medicine
National Aeronautics and Space Administration (2022). NASA’s space launch system reference guide. Available at: https://www.nasa.gov/sites/default/files/atoms/files/sls_reference_guide_2022_v2_508.pdf.
National Aeronautics and Space Administration (2017). S3001: Guidelines for risk management. https://www.nasa.gov/sites/default/files/atoms/files/s3001_guidelines_for_risk_management_-_ver_g_-_10-25-2017.pdf.
National Research Council (2011). Vision and voyages for planetary science in the decade 2013-2022. Washington, D.C.: National Academies Press. doi:10.17226/13117
Neumann, W., and Kruse, A. (2019). Differentiation of Enceladus and retention of a porous core. Astrophys. J. 882 (1), 47. doi:10.3847/1538-4357/ab2fcf
Neveu, M., Hays, L. E., Voytek, M. A., New, M. H., and Schulte, M. D. (2018). The ladder of life detection. Astrobiology 18 (11), 1375–1402. doi:10.1089/ast.2017.1773
New, J. S., Kazemi, B., Spathis, V., Price, M. C., Mathies, R. A., and Butterworth, A. L. (2021). Quantitative evaluation of the feasibility of sampling the ice plumes at Enceladus for biomarkers of extraterrestrial life. Proc. Natl. Acad. Sci. U. S. A. 118, e2106197118. doi:10.1073/pnas.2106197118
Palma, D. F. F. (2016). Preliminary trajectory design of a mission to Enceladus, master of science degree in Aerospace engineering. Lisbon, Portugal: Instituto Tecnico Lisbon.
Parkinson, C. D., Liang, M.-C., Yung, Y. L., and Kirschivnk, J. L. (2008). Habitability of Enceladus: Planetary conditions for life. Orig. Life Evol. Biosph. 38, 355–369. doi:10.1007/s11084-008-9135-4
Porco, C. C., DiNino, D., and Nimmo, F. (2014). How the geysers, tidal stress, and thermal emission across the South polar terrain of Enceladus are related. Astron. J. 148 (3), 45. doi:10.1088/0004-6256/148/3/45
Porco, C. C., Helfenstein, P., Thomas, P. C., Ingersoll, A. P., Wisdom, J., West, R. D., et al. (2006). Cassini observes the active south pole of Enceladus. Science 311 (5766), 1393–1401. doi:10.1126/science.1123013
Postberg, F., Kempf, S., Hillier, J. K., Srama, R., Green, S. F., McBride, N., et al. (2008). The E-ring in the vicinity of Enceladus. Icarus 193 (2), 438–454. doi:10.1016/j.icarus.2007.09.001
Postberg, F., Kempf, S., Schmidt, J., Brilliantov, N., Beinsen, A., Abel, B., et al. (2009). Sodium salts in E-ring ice grains from an ocean below the surface of Enceladus. Nature 459 (7250), 1098–1101. doi:10.1038/nature08046
Postberg, F., Khawaja, N., Abel, B., Choblet, G., Glein, C. R., Gudipati, M. S., et al. (2018). Macromolecular organic compounds from the depths of Enceladus. Nature 558 (7711), 564–568. doi:10.1038/s41586-018-0246-4
Postberg, F., Schmidt, J., Hillier, J., Kempf, S., and Srama, R. (2011). A salt-water reservoir as the source of a compositionally stratified plume on Enceladus. Nature 474 (7353), 620–622. doi:10.1038/nature10175
Rabinovitch, J., Mihaly, J., Parziale, N., Mehrotra, P., Cymbalist, N., Burgoyne, H., et al. (2014). “The Caltech space challenge: Lessons learned and future plans,” in 65th international astronautical congress.
Roberts, J. H., and Nimmo, F. (2008). Tidal heating and the long-term stability of a subsurface ocean on Enceladus. Icarus 194, 675–689. doi:10.1016/j.icarus.2007.11.010
Rovira‐Navarro, M., Katz, R. F., Liao, Y., Wal, W., and Nimmo, F. (2022). The tides of Enceladus’ porous core. J. Geophys. Res. Planets 127, e2021JE007117–27. doi:10.1029/2021je007117
Rudolph, M. L., Manga, M., Walker, M., and Rhoden, A. R. (2022). Cooling crusts create concomitant cryovolcanic cracks. Geophys. Res. Lett. 49 (5), 1–11. doi:10.1029/2021gl094421
Russell, R. P., and Martin, L. (2009). On the design of an Enceladus science orbit. Acta Astronaut. 65, 27–39. doi:10.1016/j.actaastro.2009.01.021
Sekine, Y., Shibuya, T., Postberg, F., Hsu, H. W., Suzuki, K., Masaki, Y., et al. (2015). High-temperature water-rock interactions and hydrothermal environments in the chondrite-like core of Enceladus. Nat. Commun. 6, 8604. doi:10.1038/ncomms9604
Spahn, F., Schmidt, J., Albers, N., Hörning, M., Makuch, M., Seiss, M., et al. (2006). Cassini dust measurements at Enceladus and implications for the origin of the E ring. Science 311 (5766), 1416–1418. doi:10.1126/science.1121375
Spencer, J. R., and Niebur, C. (2010). Planetary Science Decadal Survey: Enceladus Orbiter Mission Concept Study. Washington, DC, United States: NASA.
Spiers, E. M., Weber, J. M., Venigalla, C., Annex, A. M., Chen, C. P., Lee, C., et al. (2021). Tiger: Concept study for a new Frontiers Enceladus habitability mission. Planet. Sci. J. 2 (5), 195. doi:10.3847/PSJ/ac19b7
Tajeddine, R., Soderlund, K. M., Thomas, P. C., Helfenstein, P., Hedman, M. M., Burns, J. A., et al. (2017). True polar wander of Enceladus from topographic data. Icarus 295, 46–60. doi:10.1016/j.icarus.2017.04.019
Taylor, J., Sakamoto, L., and Wong, C. (2002). Cassini orbiter/huygens probe telecommunications. DESCANSO Design and Performance Summary Series. Pasadena, CA: Jet Propulsion Laboratory.
Vance, S., Behounkova, M., Bills, B. G., Byrne, P., Cadek, O., Castillo-Rogez, J., et al. (2021). Distributed geophysical exploration of Enceladus and other ocean worlds. Bull. AAS 53 (4). doi:10.3847/25c2cfeb.a07234f4
Vance, S. D., Panning, M. P., Stähler, S., Cammarano, F., Bills, B. G., Tobie, G., et al. (2018). Geophysical investigations of habitability in ice-covered ocean worlds. JGR. Planets 123 (1), 180–205. doi:10.1002/2017JE005341
Waite, J. H., Combi, M. R., Ip, W. H., Cravens, T. E., McNutt, R. L., Kasprzak, W., et al. (2006). Cassini ion and neutral mass spectrometer: Enceladus plume composition and structure. Science 311 (5766), 1419–1422. doi:10.1126/science.1121290
Keywords: Enceladus, mission concept, habitability, planetary science, icy moons, astrobiology
Citation: Nathan E, Balachandran K, Cappuccio P, Di J, Doerksen K, Gloder A, Li M, Massarweh L, Peev T, Santra S, Rovira-Navarro M and Limonchik D (2022) A multi-lander New Frontiers mission concept study for Enceladus: SILENUS. Front. Astron. Space Sci. 9:995941. doi: 10.3389/fspas.2022.995941
Received: 16 July 2022; Accepted: 17 August 2022;
Published: 14 October 2022.
Edited by:
Francesca Altieri, Institute for Space Astrophysics and Planetology (INAF), ItalyReviewed by:
Akos Kereszturi, Hungarian Academy of Sciences (MTA), HungaryRicardo Amils, Autonomous University of Madrid, Spain
Copyright © 2022 Nathan, Balachandran, Cappuccio, Di, Doerksen, Gloder, Li, Massarweh, Peev, Santra, Rovira-Navarro and Limonchik. This is an open-access article distributed under the terms of the Creative Commons Attribution License (CC BY). The use, distribution or reproduction in other forums is permitted, provided the original author(s) and the copyright owner(s) are credited and that the original publication in this journal is cited, in accordance with accepted academic practice. No use, distribution or reproduction is permitted which does not comply with these terms.
*Correspondence: Erica Nathan, ZXJpY2FfbmF0aGFuQGJyb3duLmVkdQ==