- 1Institute for Applied Physics, Goethe University Frankfurt, Frankfurt, Germany
- 2INAF—Osservatorio Astrofisico D’Abruzzo, Teramo, Italy
- 3INFN—Sezione di Perugia, Perugia, Italy
- 4Los Alamos National Laboratory, Los Alamos, NM, United States
Neutron star mergers (NSMs) are one of the astrophysical sites for the occurrence of the rapid neutron capture process (r-process). After a merger, the ejected neutron-rich matter hosts the production of radioactive heavy nuclei located far from the stability valley. Their nuclear physics properties are key inputs for r-process nucleosynthesis calculations. Here, we focus on the importance of neutron-capture rates and perform a sensitivity study for typical outflows from NSMs. We identify the rates with the highest impact on the final r-process abundance pattern and the nuclear energy release, therefore determining the nucleosynthesis in NSMs. A list of major n-capture rates affecting individual isotopes and elements production is also provided.
1 Introduction
Elements heavier than iron are mainly synthesized, to a nearly equal proportion, through the slow neutron capture process (s-process) (Käppeler et al., 2011) and the rapid neutron capture process (r-process) (Cowan et al., 2021). One of the most challenging problems in current nuclear physics and astrophysics is identifying the astrophysical r-process sites. The production of r-process nuclei requires very high neutron densities (nn > 1020 cm−3) and temperatures (T > 109 K). Therefore, the most obvious candidates are explosive events involving either the collapse of single massive stars or the merger of compact remnants such as binary neutron star (BNS) or black hole (BH)-neutron star (BHNS) systems.
In compact binary merger (CBM) systems, energy is released through the emission of gravitational waves. As a consequence, the loss of energy gradually makes the binary orbit to shrink, and the inspiral of the two compact objects ends with their merger. Numerical simulations show that, during the last phase of the coalescence, the matter is dynamically ejected on timescales of milliseconds into two components: a cold and very neutron-rich (electron fraction Ye ∼ 0.1) tidal ejecta mainly distributed on the orbital plane (equatorial angles) and a more isotropic shock-heated ejecta originating from the contact interface between the two objects. The former can be observed both in BNS and BHNS systems and is constituted by material from the tidal disruption of the neutron star. The latter is typically associated with a BNS system. In this case, the dynamical ejecta, especially at high latitudes (polar angles), is subject to pair processes and neutrino irradiation from the central remnant, which increase Ye up to
Short gamma-ray burst and kilonova emission are typical electromagnetic signals associated with CBMs [see Metzger (2019), for a review]. In BNS mergers, the neutron-rich matter is ejected through various channels and, as it expands into space, hosts the production of r-process nuclei, whose radioactive decay heats the ejecta. The ensuing radiation eventually emerges from the optically-thick ejecta and powers an electromagnetic transient known as kilonova. The first phenomenon of this kind to be observed was the electromagnetic counterpart AT2017gfo (Arcavi et al., 2017; Coulter et al., 2017; Drout et al., 2017; Evans et al., 2017; Kasliwal et al., 2017; Nicholl et al., 2017; Pian et al., 2017; Smartt et al., 2017; Soares-Santos et al., 2017; Tanvir et al., 2017) of the gravitational wave detection GW170817 of a BNS merger (Abbott et al., 2017a,b). The fair agreement of the luminosity and bolometric light curve evolution of AT2017gfo to kilonova models considering the heating rate and opacity expected from freshly synthesized r-process elements (Kasen et al., 2017; Perego et al., 2017; Villar et al., 2017; Tanaka et al., 2017; Wollaeger et al., 2018; Watson et al., 2019; Gillanders et al., 2022), provided the first direct indication that the r-process elements are actually produced in neutron star mergers (NSMs).
During the r-process nucleosynthesis in NSM ejecta, the attained neutron fluxes are so high to cause unstable neutron-rich nuclei, located along the dripline, to be rapidly synthesized, possibly also populating the region in which nuclear fission is extremely efficient. The early r-process is characterized by (n, γ) ⇄ (γ, n) equilibrium, with neutron capture on heavy nuclei proceeding much faster than β-decay (Arcones and Martínez-Pinedo, 2011). During this early phase, the isotope abundances depend on the temperature, the neutron abundance, and the neutron separation energies. The latter is determined by nuclear masses, and so by the nuclear-mass model considered for these unstable heavy nuclei (Sprouse et al., 2020). At some point, however, the availability of free neutrons is drastically reduced and a freeze-out occurs. This happens when the neutron-to-seed ratio, namely the neutrons captured per seed nucleus, drops below unity. After freeze-out, the equilibrium is no longer maintained and competition between neutron captures, photodissociations, β-decay, and β-delayed neutron emission arises. The interplay between them shapes the final abundance distribution of heavy elements, and the nuclear heating rate powering the possible electromagnetic transients associated with the r-process astrophysical scenarios.
Within this framework, knowing the nuclear properties of neutron-rich unstable nuclei, such as nuclear masses, β-decay rates, neutron-capture rates, and fission fragment distributions [see, e.g., Mumpower et al. (2016), Kullmann et al. (2022)], is crucial for r-process nucleosynthesis calculations. Unfortunately, for most of these nuclei, they are poorly known, and we have to rely on theoretical models, whose predictions can largely vary, depending on the assumptions made (Horowitz et al., 2019). This is particularly true for neutron-capture rates, whose direct experimental measurements are currently unfeasible. The half-life limit for direct measurements using current facilities is typically a few years (Couture and Reifarth, 2007; Reifarth et al., 2018). Capture cross sections of isotopes with shorter half-lives can only be investigated indirectly (Reifarth et al., 2014) or have to be based on theoretical estimates.
In this respect, next-generation neutron facilities might make the production and the study of many exotic nuclei accessible, thus providing key nuclear information of interest for r-process studies (Reifarth and Litvinov, 2014; Reifarth et al., 2017).
As a matter of fact, the theoretical rates determined using the Hauser–Feshbach (HF) statistical model (Hauser and Feshbach, 1952) are very often the sole option. However, variations by over two to three orders of magnitudes are predicted, depending on the different choices for nuclear structure properties, optical potentials, level densities, and γ-ray strengths of nuclei located far from the beta stability valley [see, e.g., Arnould et al. (2007)].
Sensitivity studies aiming at determining the impact of nuclear physics uncertainties on abundance predictions for astrophysical model conditions suitable to produce the weak and the main r-process components were performed in the past [e.g., Surman et al. (2009); Mumpower et al. (2012); Surman et al. (2014); Mumpower et al. (2016)]. More recently, uncertainties arising from different adopted mass models, spontaneous fission rates, and fission fragment distribution on the radioactive heating and kilonova emissions by mass outflows from NSMs have been investigated as well (Zhu et al., 2021; Barnes et al., 2021).
Here, we focus on neutron-capture rates and explore the impact of their variation for a range of initial astrophysical conditions typical of NSM ejecta. In particular, we study r-process nucleosynthesis in material that is either dynamically ejected during the merger of BNS [see also Mumpower et al. (2016), and references therein] or after the merger in the form of wind outflows over longer timescales. For the set of astrophysical trajectories considered, we perform a sensitivity study by changing single n-capture rates. We identify the nuclides that mostly affect the r-process abundance pattern and produce the larger variations in the total energy released by the decay of r-process elements. Finally, the inventory of isotopes and elements with the highest sensitivity to single n-capture rate changes is presented.
2 Methods
We make use of the freely available nuclear network code SkyNet (Lippuner and Roberts, 2017), which includes more than 7,000 isotopes up to Cn. We adopt the same setup used in Perego et al. (2022), except for the strong and weak nuclear reactions, for which we employ the latest default version of the JINA REACLIB library (from 06/20/2021).
We have performed r-process calculations for a NSM scenario. In particular, five parameterized fluid trajectories are considered, representative of the conditions in initial electron fraction (Ye1), initial entropy (s), and expansion timescale (τ), within the material from the dynamical ejecta (both at polar and equatorial angles (Radice et al., 2018; Bernuzzi et al., 2020; Perego et al., 2022), the spiral-wave wind ejecta (Nedora et al., 2019, 2021), the neutrino-driven wind ejecta (Perego et al., 2014; Martin et al., 2015), and the viscosity-driven wind ejecta (Fernández and Metzger, 2013; Just et al., 2015; Wu et al., 2016). Specifically, we adopted the following (Ye, s [kB baryon−1], τ [ms]) combinations:
• (0.05, 8, and 10) for the dynamical ejecta at equatorial latitudes
• (0.35, 30, and10) for the dynamical ejecta at polar latitudes
• (0.30, 20, and 10) for the spiral-wave wind ejecta
• (0.35, 15, and 30) for the ν-driven disk wind ejecta
• (0.25, 20, and 80) for the viscous ejecta
In the following, we will refer to these models as DynEq, DynPo, SpiWW, DisWN, and DisWV, respectively. All the trajectories are initialized in nuclear statistical equilibrium (NSE) conditions at T0 = 6 GK. The initial density is accordingly determined from solving for NSE at the given Ye and s. The subsequent evolution is then set to follow an initially exponentially decreasing profile with time (ρ ∝ e−t/τ), smoothly switching to a homologous expansion (ρ ∝ t−3) at t = 3τ (Lippuner and Roberts, 2015; Perego et al., 2022). The temperature is evolved accounting for the heating from nuclear reactions [e.g., Freiburghaus et al. (1999)]. To obtain the final abundances, the nuclear reaction network is evolved up to 10 Myr. Figure 1 shows the final abundance pattern obtained for the chosen astrophysical trajectories. Their comparison with the solar r-process residuals shows that the ensemble of trajectories is able to approximately reproduce all the data range up to the heaviest nuclei. Specifically, the DynEq ejecta produces a full r-process pattern, inclusive of the second and third r-process peak elements, whose relative abundances are close to solar ones. Actinides are significantly produced as well. DynPo, SpiWW, and DisWN ejecta do instead lead to a weak r-process, being only the light r-process elements to be synthesized. The DisWV case extends up to the second r-process peak but does not produce lanthanides. These simulations served as a baseline for the sensitivity study. The latter was performed by first varying a single neutron-capture rate and then recomputing a new simulation, whose predictions for the final abundance patterns were compared with the baseline ones. (n, γ) rates were individually changed by either multiplying or dividing by a constant factor. Since current theoretical compilations of neutron-capture rates are discrepant by two to three orders of magnitudes, a representative factor of 100 was considered [see Mumpower et al. (2016), and references therein]. The sensitivity study calculations were restricted to those rates whose target nuclei reach an abundance of at least Y = 10–10 at any time step of the baseline simulation and have a half-life larger than 1 s, according to the evaluated data of NUBASE 2020 (Kondev et al., 2021). We further restrict the selection on those isotopes with a charge number Z ≥ 20, since the lighter nuclei are scarcely synthesized, except hydrogen and helium, whose production is not influenced by neutron-capture rates (Perego et al., 2022). This procedure allows us to focus on those rates that may have the greatest impact on the final r-process abundance pattern and have a good chance to be measured by future experiments and, at the same time, avoid performing a massive number of simulations. The number of changed neutron-capture rates varies from
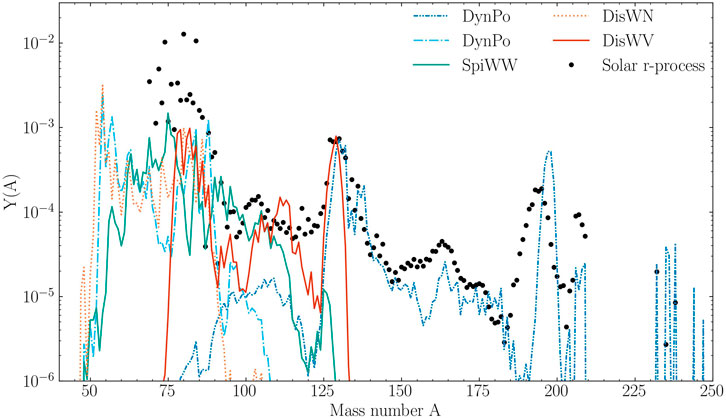
FIGURE 1. Final abundances Y(A) versus mass number A for the five parameterized baseline astrophysical trajectories representative of the different NSM ejecta channels described in the text: matter dynamically ejected during the merger at equatorial (DynEq) and polar (DynPo) angles (blue and cyan lines, respectively), and winds expelled after the merger due to the propagation of spiral arms in the NS remnant (SpiWW; green line), neutrino irradiation (DisWN; orange line), and viscous processes (DisWV; red line). The scaled solar r-residuals, obtained by multiplying the solar system abundances of Lodders (2021) by the r-fractions from Prantzos et al. (2020), are shown for comparison.
3 Results and discussion
In Figures 2, 3, we show the results of the study for all the considered outflows from NSM. The bands represent the variance in the final abundances from changing individual (n, γ) rates of a factor of 100. The importance of neutron captures is clearly demonstrated as they produce significant variations, up to one order of magnitude, in the overall abundance pattern. For each trajectory, n-capture rates become important at the freeze-out, when β-decays take over and the r-process path moves toward stability (Surman et al., 1997). This happens later in time in the more neutron-rich ejecta due to the higher-attained neutron-to-seed ratio. During this phase, the final r-process abundance pattern is affected by both an early-freeze-out photodissociation effect and a late-freeze-out neutron capture effect (Surman et al., 2009). If the temperatures are sufficiently high, the photodissociation of abundant nuclei, populated through neutron capture, can still be efficient and the r-process path can move back to lower mass numbers, so making available further neutrons to be captured and modifying the nucleosynthesis path. When instead, as the temperature is decreasing, the r-process path shifts toward stability, late-time neutron captures are effective and compete with β-decays, possibly altering the neutron density and the β-decay pathways of the most abundant nuclei, especially of the closed-shell nuclei.
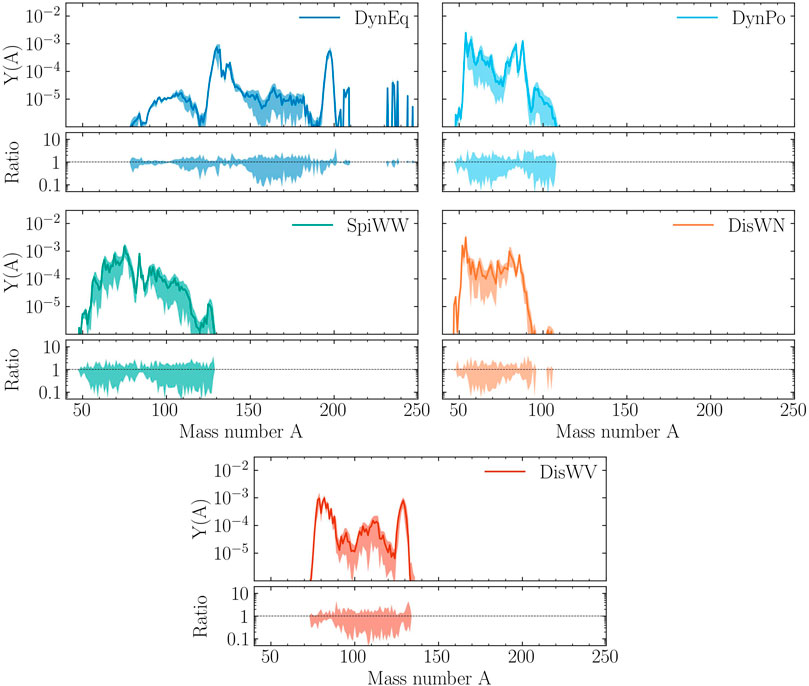
FIGURE 2. Upper panels: Variance in the isotopic abundance patterns (shaded bands), corresponding to the sensitivity studies for the different chosen trajectories. Lower panels: Ratio of final abundances with respect to the baseline. Color code as shown in Figure 1.
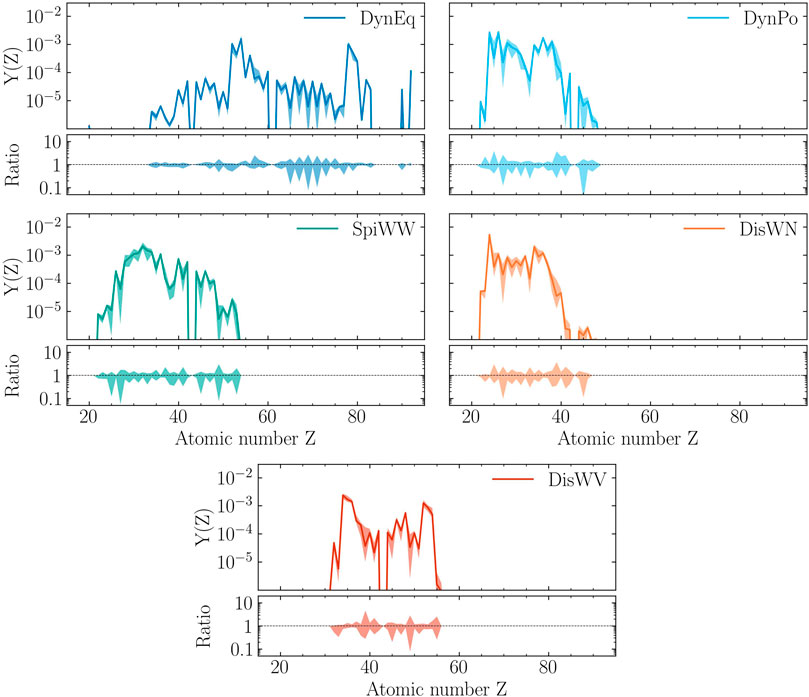
FIGURE 3. Same as shown in Figure 2 but for elemental abundances.
In turn, these effects also influence the radioactive energy generation rate per unit mass, ϵ(t), from the various decay channels: β-decay, α-decay, and spontaneous fission of heavy nuclei (Li and Paczyński, 1998; Metzger et al., 2010; Zhu et al., 2018; Wu et al., 2019; Hotokezaka and Nakar, 2020).
Figure 4 shows the fluctuations induced by changing the neutron-capture rates for the various trajectories in the heating rate ϵ(t). The latter is well represented by a power law as a function of the time, even if the actual shape depends on the ejecta composition and therefore on the initial Ye (Lippuner and Roberts, 2015; Wanajo, 2018). In general, noteworthy variations are found for all the considered case studies and in particular for the high-Ye ejecta ones. DynPo, SpiWW, and DisWN models do, in fact, produce a considerable amount of nuclei only in the limited range 50 ≲ A ≲ 90, where very few isotopes have half-lives of 10–100 days that may produce marked features in bolometric kilonova lightcurves (Wu et al., 2019).
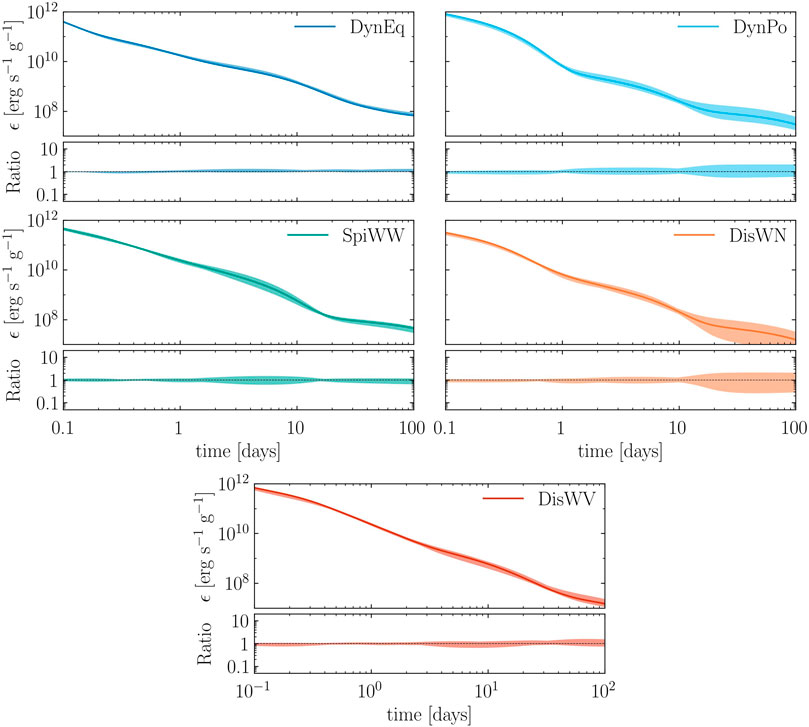
FIGURE 4. Upper panels: Variance in the nuclear heating rate (shaded bands), corresponding to the sensitivity studies for the different chosen trajectories, in the time window 0.1–100 days. Lower panels: Ratio of the heating rate with respect to the baseline. Color code as shown in Figure 1.
In the sensitivity study, for each new simulation, the impact of changing an individual neutron-capture rate on the final abundance pattern is quantified with a sensitivity measure, FA, that is suited to estimate the global changes arising both from large local variations and small variations along the abundance pattern (Surman et al., 2009; Mumpower et al., 2016); it is defined as follows:
where X(A) = A ⋅ Y(A) are final mass fractions of the simulation with the rate varied, and Xb(A) are the final mass fractions for the baseline abundance pattern.
Given the fact that the r-process is associated with explosive events, it is not possible to have the evidence of its in situ nucleosynthesis with observations of stellar spectra, as instead is the case of the s-process (Käppeler et al., 2011). As a consequence, one usually looks at the solar system abundances of heavy nuclei to get information about the typical r-process abundance pattern. In particular, the r-process contribution to the solar system neutron-capture abundances is determined by subtracting the s-process contribution [e.g., Prantzos et al. (2020)]. The residual r-process pattern provides an extremely useful benchmark for comparing abundance predictions of r-process simulations at an isotopic level (see Figure 2). However, a growing number of stellar abundance observations of very metal-poor stars showed that the solar r-process pattern is not universal and that a star-to-star scatter for elemental abundance distributions is present [e.g., Sneden et al. (2008); Cowan et al. (2021), and references therein]. The results of our study shown in Figure 3 demonstrate that changes in neutron-capture rates have an impact on elemental abundances as well. In order to quantify such uncertainties, a second sensitivity measure is adopted, FZ, which is appropriate to describe local changes to individual elements by single neutron-capture rate variations, and it is defined as follows:
Analogously, to describe local changes to individual isotopes, we define FZ,A as follows:
To describe the variations in the nuclear decay heating rate ϵ(t) at a time t, we introduce another sensitivity measure, Dt, which is defined as follows:
The final sensitivity measures FA, FZ, FZ,A, and Dt are computed as an average between the values obtained considering the case where a single neutron-capture rate is increased and decreased by a factor of 100.
Figure 5 and Table 1 show the nuclei with the greatest sensitivity FA obtained in the full set of studies. Regardless of the different astrophysical trajectories considered, the nuclei having the greatest influence on the r-process in NSM scenarios are those located across the neutron closed-shell regions [see also Mumpower et al. (2016), and references therein]. In particular, depending on the considered shell, we can individuate different subsets. In the N = 50 region, neutron-rich isotopes of copper, zinc, gallium, selenium, and bromine show the highest impact. Cadmium, indium, tin, and tungsten isotopes are the most important in N = 82, 126 regions. When considering trajectories with high electron fraction (Ye = 0.35), also neutron-rich isotopes in the vicinity of N = 28 zone, of elements such as calcium, titanium, vanadium, and chromium, affect r-process abundance distribution.
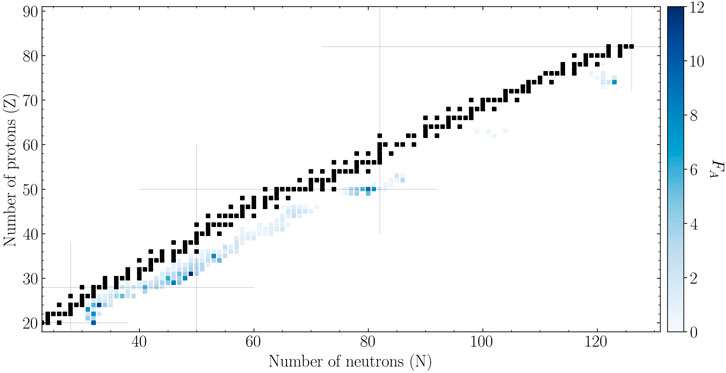
FIGURE 5. Combined results of all neutron-capture rate sensitivity studies performed. The shading represents the sensitivity measure FA. Only nuclei with sensitivity values FA greater than 0.5 in at least one of considered astrophysical trajectories are shaded. Black squares denote stable nuclei.
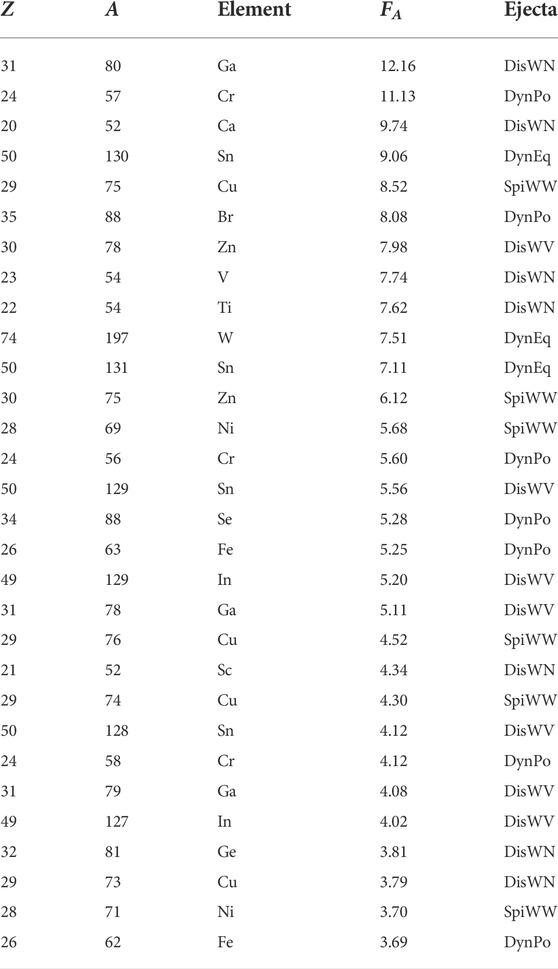
TABLE 1. Top 30 nuclei with maximum neutron-capture rate sensitivity measure FA for the five NSM ejecta trajectories considered in this study. For each specific nucleus, the relative FA value is the largest one obtained in the full set of sensitivity studies and refers to the particular type of ejecta listed.
We note that these nuclei exhibit large effects on the r-process nucleosynthesis in NSMs, as the five sets of astrophysical trajectories considered for the studies are representative of a NSM scenario. In principle, other nuclei may be important as well in other r-process scenarios. Nonetheless, determination of these n-capture cross sections should be of top priority in measurement campaigns at current and future exotic RIB facilities, given that NSMs are acknowledged to be an r-process site, even if other sources might be needed to explain the r-process enrichment in the Universe [e.g., Côté et al. (2019); Skúladóttir et al. (2019); Van der Swaelmen et al. (2022)].
In Table 2, the nuclei with the greatest sensitivity Dt at different considered times are listed. In particular, each Di refers to a time equal to t = 10i days. So, for example, D0 is the sensitivity to a specific neutron-capture rate for the radioactive heating rate at 1 day. It is straightforward noticing that the effect of varying the neutron-capture rates on the nuclear decay heating rate is somewhat milder, being within a factor of
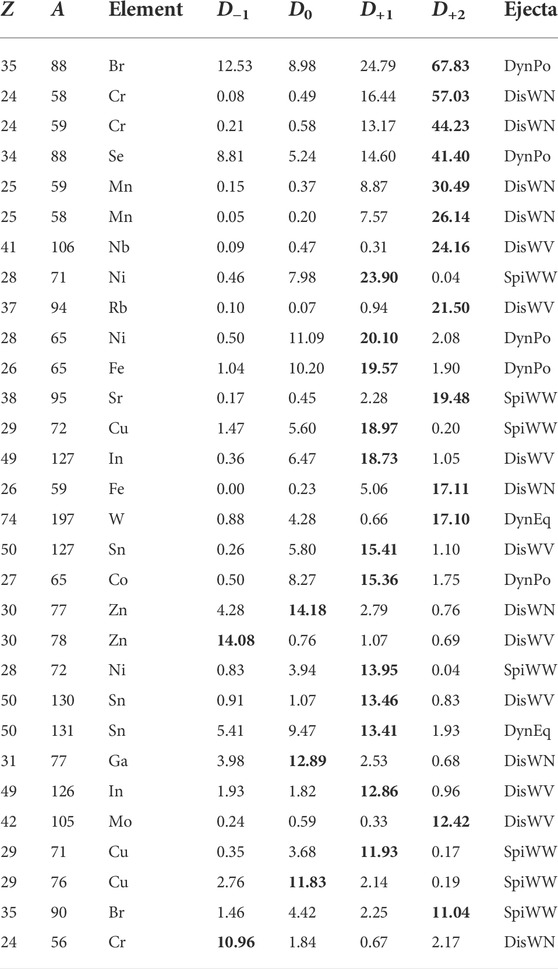
TABLE 2. Top 30 nuclei with maximum nuclear heating rate sensitivity measure Dt (see text for details). The maximum value attained at any time t = 0.1, 1, 10, 100 days is set in boldface. For each specific nucleus, the relative Dt value is the largest one obtained in the full set of sensitivity studies and refers to the particular type of ejecta listed.
We note that nuclei with larger Dt measures are located just on the right of N = 28 and N = 50 regions of the nuclear chart (see Figure 6).
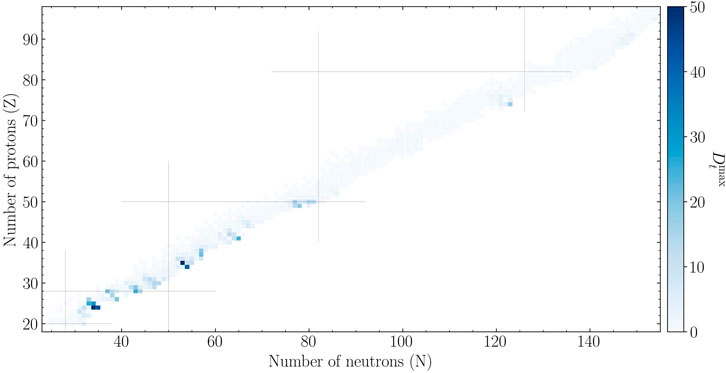
FIGURE 6. Nuclear heating rate sensitivity across the nuclear chart. For each nucleus, the shading represents the maximum sensitivity measure Dt attained at any time t = 0.1, 1, 10, 100 days.
Depending on the production site, special isotopes or elements can be of interest in nuclear astrophysics, e.g., because they can be measured in solar system material or detected in stellar spectra. In this regard, it is useful also to evaluate which of them are mostly affected by neutron-capture rate variations and the rates responsible for that. The most sensitive elements to n-capture rate variations are listed in Table 3. The maximum local sensitivity,
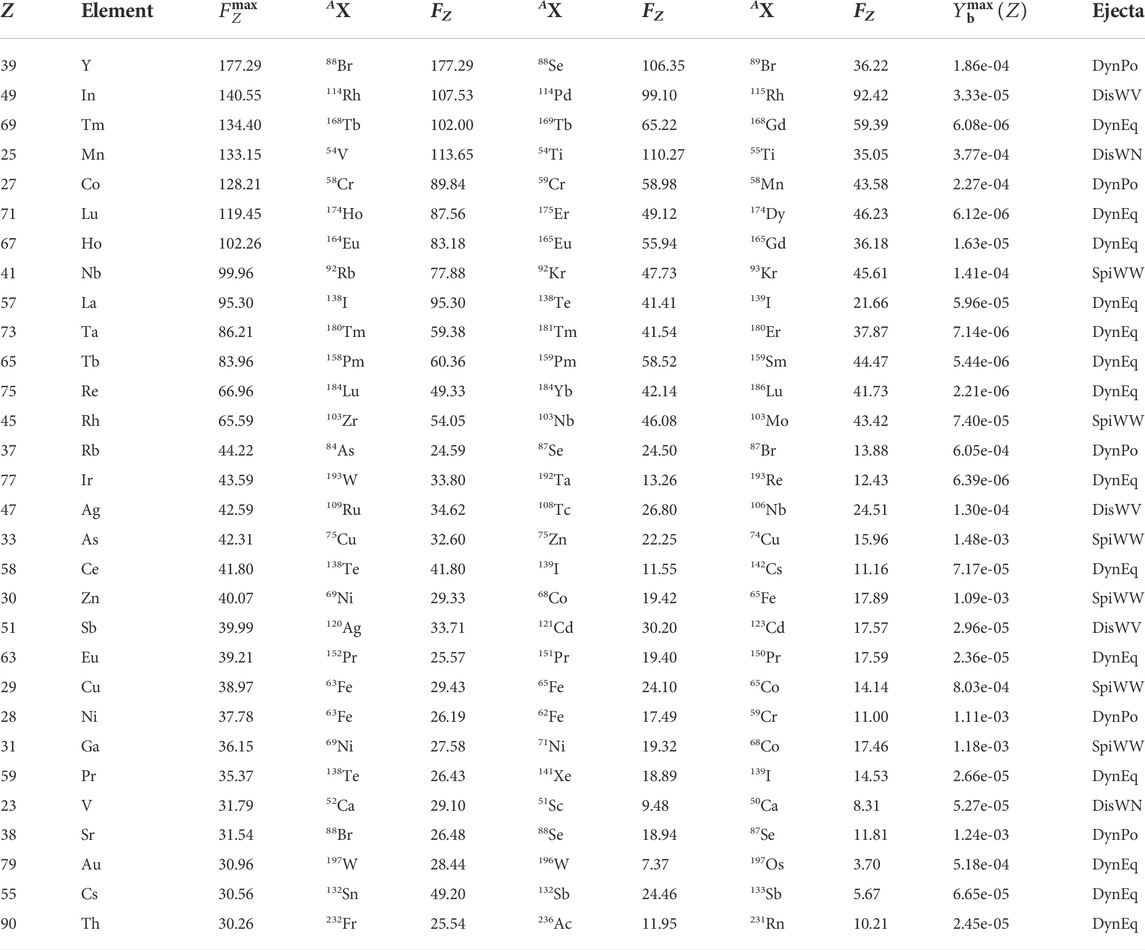
TABLE 3. 30 most sensitive elements with the relative maximum local sensitivity
where Ymax(Z) is the abundance at the top of the uncertainty band for atomic number Z, and Ymin(Z) is the one at the bottom (see Figure 3). The top three rates that have the biggest effects on individual elements are indicated as well. Similarly, the isotopes whose abundance is most affected by the changes in the neutron-capture rates are shown in Table 4. Their maximum local sensitivity,
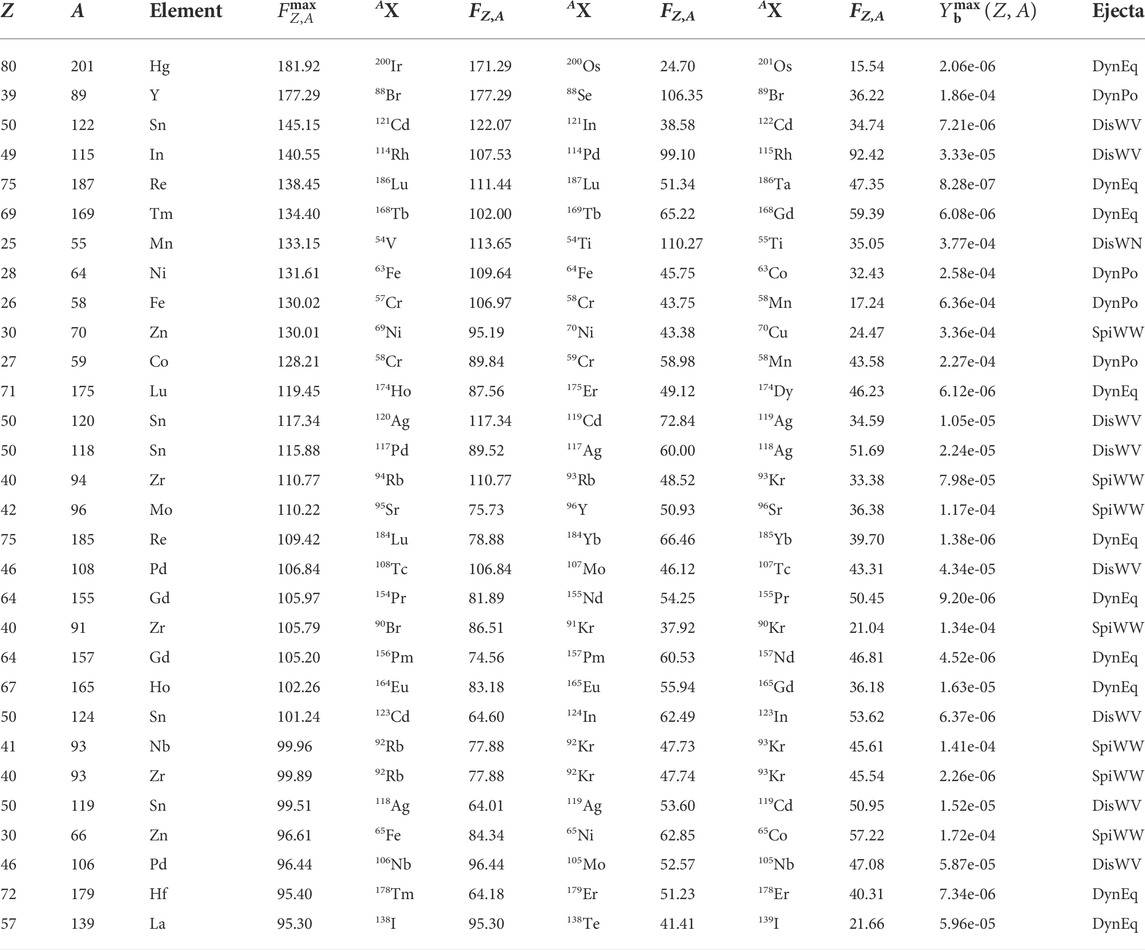
TABLE 4. 30 most sensitive isotopes with the relative maximum local sensitivity
4 Conclusions
The impact of neutron-capture rate uncertainties on the r-process in neutron star mergers has been systematically studied for five different astrophysical trajectories representative of various ejecta channels from NSMs. We investigated the sensitivity of the relative r-process abundance yields, focusing on the capture rates of relatively long-lived nuclei. In accordance with prior studies (Surman et al., 2009; Mumpower et al., 2012, 2016), we found that the most significant n-capture rates are those involving nuclei located in the vicinity of neutron-closed shells N = 50, 82, 126, while for high-Ye ejecta, isotopes in the N = 28 region considerably influence the final abundance pattern as well.
Rate variations also affects the nuclear heating rate at timescales relevant for the kilonova emission, especially at late times (t ≳ 10 days) and for high-Ye trajectories, where a few nuclei with a comparable β-decay lifetimes contribute the most to the heating.
Finally, the most sensitive isotopes and elements to n-capture rate changes were determined and listed, along with the rates that largely influence their production. The results presented in this study can be used as guidance to prioritize future experimental campaigns for the determination of neutron-capture rate reaction of interest for the r-process occurring in NSMs.
Data availability statement
The raw data supporting the conclusions of this article will be made available by the authors, without undue reservation.
Author contributions
DV and RR conceptualized the study. DV performed the simulations and carried out the analysis. DV took care of the original draft preparation. All authors contributed to the review and editing of the manuscript and approved the submitted version.
Funding
AC was supported by the U.S. Department of Energy through the Los Alamos National Laboratory, operated by Triad National Security, LLC, for the National Nuclear Security Administration of the U.S. Department of Energy (Contract No. 89233218CNA000001). DV was supported by the German-Israeli Foundation (GIF No. I-1500-303.7/2019).
Acknowledgments
DV thanks A. Perego, S. Giuliani, and L. Chiesa for fruitful discussions concerning the modeling of the r-process nucleosynthesis in NSMs.
Conflict of interest
The authors declare that the research was conducted in the absence of any commercial or financial relationships that could be construed as a potential conflict of interest.
Publisher’s note
All claims expressed in this article are solely those of the authors and do not necessarily represent those of their affiliated organizations, or those of the publisher, the editors, and the reviewers. Any product that may be evaluated in this article, or claim that may be made by its manufacturer, is not guaranteed or endorsed by the publisher.
Footnotes
1The electron fraction is defined as the ratio between net density of electrons and the total baryon number density.
References
Abbott, B. P., Abbott, R., Abbott, T. D., Acernese, F., Ackley, K., Adams, C., et al. (2017a). GW170817: Observation of gravitational waves from a binary neutron star inspiral. Phys. Rev. Lett. 119, 161101. doi:10.1103/PhysRevLett.119.161101
Abbott, B. P., Abbott, R., Abbott, T. D., Acernese, F., Ackley, K., Adams, C., et al. (2017b). Multi-messenger observations of a binary neutron star merger. Astrophys. J. 848, L12. doi:10.3847/2041-8213/aa91c9
Arcavi, I., Hosseinzadeh, G., Howell, D. A., McCully, C., Poznanski, D., Kasen, D., et al. (2017). Optical emission from a kilonova following a gravitational-wave-detected neutron-star merger. Nature 551, 64–66. doi:10.1038/nature24291
Arcones, A., and Martínez-Pinedo, G. (2011). Dynamicalr-process studies within the neutrino-driven wind scenario and its sensitivity to the nuclear physics input. Phys. Rev. C 83, 045809. doi:10.1103/PhysRevC.83.045809
Arnould, M., Goriely, S., and Takahashi, K. (2007). The r-process of stellar nucleosynthesis: Astrophysics and nuclear physics achievements and mysteries. Phys. Rep. 450, 97–213. doi:10.1016/j.physrep.2007.06.002
Barnes, J., Zhu, Y. L., Lund, K. A., Sprouse, T. M., Vassh, N., McLaughlin, G. C., et al. (2021). Kilonovae across the nuclear physics landscape: The impact of nuclear physics uncertainties on r-process-powered emission. Astrophys. J. 918, 44. doi:10.3847/1538-4357/ac0aec
Bernuzzi, S., Breschi, M., Daszuta, B., Endrizzi, A., Logoteta, D., Nedora, V., et al. (2020). Accretion-induced prompt black hole formation in asymmetric neutron star mergers, dynamical ejecta, and kilonova signals. Mon. Not. R. Astron. Soc. 497, 1488–1507. doi:10.1093/mnras/staa1860
Ciolfi, R., and Kalinani, J. V. (2020). Magnetically driven baryon winds from binary neutron star merger remnants and the blue kilonova of 2017 august. Astrophysical J. Lett. 900, L35. doi:10.3847/2041-8213/abb240
Côté, B., Eichler, M., Arcones, A., Hansen, C. J., Simonetti, P., Frebel, A., et al. (2019). Neutron star mergers might not Be the only source of r-process elements in the milky way. Astrophys. J. 875, 106. doi:10.3847/1538-4357/ab10db
Coulter, D. A., Foley, R. J., Kilpatrick, C. D., Drout, M. R., Piro, A. L., Shappee, B. J., et al. (2017). Swope Supernova Survey 2017a (SSS17a), the optical counterpart to a gravitational wave source. Science 358, 1556–1558. doi:10.1126/science.aap9811
Couture, A., and Reifarth, R. (2007). Direct measurements of neutron capture on radioactive isotopes. Atomic Data Nucl. Data Tables 93, 807–830. doi:10.1016/j.adt.2007.06.003
Cowan, J. J., Sneden, C., Lawler, J. E., Aprahamian, A., Wiescher, M., Langanke, K., et al. (2021). Origin of the heaviest elements: The rapid neutron-capture process. Rev. Mod. Phys. 93, 015002. doi:10.1103/RevModPhys.93.015002
Drout, M. R., Piro, A. L., Shappee, B. J., Kilpatrick, C. D., Simon, J. D., Contreras, C., et al. (2017). Light curves of the neutron star merger GW170817/SSS17a: Implications for r-process nucleosynthesis. Science 358, 1570–1574. doi:10.1126/science.aaq0049
Evans, P. A., Cenko, S. B., Kennea, J. A., Emery, S. W. K., Kuin, N. P. M., Korobkin, O., et al. (2017). Swift and NuSTAR observations of GW170817: Detection of a blue kilonova. Science 358, 1565–1570. doi:10.1126/science.aap9580
Fernández, R., and Metzger, B. D. (2013). Delayed outflows from black hole accretion tori following neutron star binary coalescence. Mon. Not. R. Astron. Soc. 435, 502–517. doi:10.1093/mnras/stt1312
Freiburghaus, C., Rosswog, S., and Thielemann, F. K. (1999). [CLC] [ITAL]r[/ITAL] [/CLC]-Process in neutron star mergers. Astrophys. J. 525, L121–L124. doi:10.1086/312343
Fujibayashi, S., Kiuchi, K., Nishimura, N., Sekiguchi, Y., and Shibata, M. (2018). Mass ejection from the remnant of a binary neutron star merger: Viscous-radiation hydrodynamics study. Astrophys. J. 860, 64. doi:10.3847/1538-4357/aabafd
Gillanders, J. H., Smartt, S. J., Sim, S. A., Bauswein, A., and Goriely, S. (2022). Modelling the spectra of the kilonova AT2017gfo - I. The photospheric epochs. Mon. Not. R. Astron. Soc. 515, 631–651. doi:10.1093/mnras/stac1258
Hauser, W., and Feshbach, H. (1952). The inelastic scattering of neutrons. Phys. Rev. 87, 366–373. doi:10.1103/PhysRev.87.366
Horowitz, C. J., Arcones, A., Côté, B., Dillmann, I., Nazarewicz, W., Roederer, I. U., et al. (2019). r-process nucleosynthesis: connecting rare-isotope beam facilities with the cosmos. J. Phys. G. Nucl. Part. Phys. 46, 083001. doi:10.1088/1361-6471/ab0849
Hotokezaka, K., and Nakar, E. (2020). Radioactive heating rate of r-process elements and macronova light curve. Astrophys. J. 891, 152. doi:10.3847/1538-4357/ab6a98
Just, O., Bauswein, A., Ardevol Pulpillo, R., Goriely, S., and Janka, H. T. (2015). Comprehensive nucleosynthesis analysis for ejecta of compact binary mergers. Mon. Not. R. Astron. Soc. 448, 541–567. doi:10.1093/mnras/stv009
Käppeler, F., Gallino, R., Bisterzo, S., and Aoki, W. (2011). Thesprocess: Nuclear physics, stellar models, and observations. Rev. Mod. Phys. 83, 157–193. doi:10.1103/RevModPhys.83.157
Kasen, D., Metzger, B., Barnes, J., Quataert, E., and Ramirez-Ruiz, E. (2017). Origin of the heavy elements in binary neutron-star mergers from a gravitational-wave event. Nature 551, 80–84. doi:10.1038/nature24453
Kasliwal, M. M., Nakar, E., Singer, L. P., Kaplan, D. L., Cook, D. O., Van Sistine, A., et al. (2017). Illuminating gravitational waves: A concordant picture of photons from a neutron star merger. Science 358, 1559–1565. doi:10.1126/science.aap9455
Kondev, F. G., Wang, M., Huang, W. J., Naimi, S., and Audi, G. (2021). The NUBASE2020 evaluation of nuclear physics properties. Chin. Phys. C 45, 030001. doi:10.1088/1674-1137/abddae
Kullmann, I., Goriely, S., Just, O., Bauswein, A., and Janka, H. T. (2022). Extensive study of nuclear uncertainties and their impact on the r-process nucleosynthesis in neutron star mergers. arXiv e-prints. arXiv:2207.07421. doi:10.48550/arXiv.2207.07421
Li, L. X., and Paczyński, B. (1998). Transient events from neutron star mergers. Astrophys. J. 507, L59–L62. doi:10.1086/311680
Lippuner, J., Fernández, R., Roberts, L. F., Foucart, F., Kasen, D., Metzger, B. D., et al. (2017). Signatures of hypermassive neutron star lifetimes on r-process nucleosynthesis in the disc ejecta from neutron star mergers. Mon. Not. R. Astron. Soc. 472, 904–918. doi:10.1093/mnras/stx1987
Lippuner, J., and Roberts, L. F. (2015). r-Process lanthanide production and heating rates in kilonovae. Astrophys. J. 815, 82. doi:10.1088/0004-637X/815/2/82
Lippuner, J., and Roberts, L. F. (2017). SkyNet: A modular nuclear reaction network library. Astrophys. J. Suppl. Ser. 233, 18. doi:10.3847/1538-4365/aa94cb
Lodders, K. (2021). Relative atomic solar system Abundances, mass fractions, and atomic masses of the elements and their isotopes, composition of the solar photosphere, and compositions of the major chondritic meteorite groups. Space Sci. Rev. 217, 44. doi:10.1007/s11214-021-00825-8
Martin, D., Perego, A., Arcones, A., Thielemann, F. K., Korobkin, O., and Rosswog, S. (2015). Neutrino-driven winds in the aftermath of a neutron star merger: Nucleosynthesis and electromagnetic transients. Astrophys. J. 813, 2. doi:10.1088/0004-637X/813/1/2
Metzger, B. D., Martínez-Pinedo, G., Darbha, S., Quataert, E., Arcones, A., Kasen, D., et al. (2010). Electromagnetic counterparts of compact object mergers powered by the radioactive decay of r-process nuclei. Mon. Not. R. Astron. Soc. 406, 2650–2662. doi:10.1111/j.1365-2966.2010.16864.x
Metzger, B. D., Thompson, T. A., and Quataert, E. (2018). A magnetar origin for the kilonova ejecta in GW170817. Astrophys. J. 856, 101. doi:10.3847/1538-4357/aab095
Mumpower, M. R., McLaughlin, G. C., and Surman, R. (2012). Influence of neutron capture rates in the rare Earth region on ther-process abundance pattern. Phys. Rev. C 86, 035803. doi:10.1103/PhysRevC.86.035803
Mumpower, M. R., Surman, R., McLaughlin, G. C., and Aprahamian, A. (2016). The impact of individual nuclear properties onr-process nucleosynthesis. Prog. Part. Nucl. Phys. 86, 86–126. doi:10.1016/j.ppnp.2015.09.001
Nedora, V., Bernuzzi, S., Radice, D., Daszuta, B., Endrizzi, A., Perego, A., et al. (2021). Numerical relativity simulations of the neutron star merger GW170817: Long-term remnant evolutions, winds, remnant disks, and nucleosynthesis. Astrophys. J. 906, 98. doi:10.3847/1538-4357/abc9be
Nedora, V., Bernuzzi, S., Radice, D., Perego, A., Endrizzi, A., and Ortiz, N. (2019). Spiral-wave wind for the blue kilonova. Astrophys. J. 886, L30. doi:10.3847/2041-8213/ab5794
Nicholl, M., Berger, E., Kasen, D., Metzger, B. D., Elias, J., Briceño, C., et al. (2017). The electromagnetic counterpart of the binary neutron star merger LIGO/virgo GW170817. III. Optical and UV spectra of a blue kilonova from fast polar ejecta. Astrophys. J. 848, L18. doi:10.3847/2041-8213/aa9029
Perego, A., Radice, D., and Bernuzzi, S. (2017). AT 2017gfo: An anisotropic and three-component kilonova counterpart of GW170817. Astrophys. J. 850, L37. doi:10.3847/2041-8213/aa9ab9
Perego, A., Rosswog, S., Cabezón, R. M., Korobkin, O., Käppeli, R., Arcones, A., et al. (2014). Neutrino-driven winds from neutron star merger remnants. Mon. Notices R. Astronomical Soc. 443, 3134–3156. doi:10.1093/mnras/stu1352
Perego, A., Vescovi, D., Fiore, A., Chiesa, L., Vogl, C., Benetti, S., et al. (2022). Production of very light elements and strontium in the early ejecta of neutron star mergers. Astrophys. J. 925, 22. doi:10.3847/1538-4357/ac3751
Pian, E., D’Avanzo, P., Benetti, S., Branchesi, M., Brocato, E., Campana, S., et al. (2017). Spectroscopic identification of r-process nucleosynthesis in a double neutron-star merger. Nature 551, 67–70. doi:10.1038/nature24298
Prantzos, N., Abia, C., Cristallo, S., Limongi, M., and Chieffi, A. (2020). Chemical evolution with rotating massive star yields II. A new assessment of the solar s- and r-process components. Mon. Not. R. Astron. Soc. 491, 1832–1850. doi:10.1093/mnras/stz3154
Radice, D., Bernuzzi, S., and Perego, A. (2020). The dynamics of binary neutron star mergers and GW170817. Annu. Rev. Nucl. Part. Sci. 70, 95–119. doi:10.1146/annurev-nucl-013120-114541
Radice, D., Perego, A., Hotokezaka, K., Fromm, S. A., Bernuzzi, S., and Roberts, L. F. (2018). Binary neutron star mergers: Mass ejection, electromagnetic counterparts, and nucleosynthesis. Astrophys. J. 869, 130. doi:10.3847/1538-4357/aaf054
Reifarth, R., Erbacher, P., Fiebiger, S., Göbel, K., Heftrich, T., Heil, M., et al. (2018). Neutron-induced cross sections - from raw data to astrophysical rates. Eur. Phys. J. Plus 133, 424. doi:10.1140/epjp/i2018-12295-3
Reifarth, R., Göbel, K., Heftrich, T., Weigand, M., Jurado, B., Käppeler, F., et al. (2017). Spallation-based neutron target for direct studies of neutron-induced reactions in inverse kinematics. Phys. Rev. Accel. Beams 20, 044701. doi:10.1103/physrevaccelbeams.20.044701
Reifarth, R., Lederer, C., and Käppeler, F. (2014). Neutron reactions in astrophysics. J. Phys. G. Nucl. Part. Phys. 41, 053101. doi:10.1088/0954-3899/41/5/053101
Reifarth, R., and Litvinov, Y. A. (2014). Measurements of neutron-induced reactions in inverse kinematics. Phys. Rev. St. Accel. Beams 17, 014701. doi:10.1103/physrevstab.17.014701
Shibata, M., Fujibayashi, S., and Sekiguchi, Y. (2021). Long-term evolution of a merger-remnant neutron star in general relativistic magnetohydrodynamics: Effect of magnetic winding. Phys. Rev. D. 103, 043022. doi:10.1103/PhysRevD.103.043022
Skúladóttir, Á., Hansen, C. J., Salvadori, S., and Choplin, A. (2019). Neutron-capture elements in dwarf galaxies. I. Chemical clocks and the short timescale of the r-process. Astron. Astrophys. 631, A171. doi:10.1051/0004-6361/201936125
Smartt, S. J., Chen, T. W., Jerkstrand, A., Coughlin, M., Kankare, E., Sim, S. A., et al. (2017). A kilonova as the electromagnetic counterpart to a gravitational-wave source. Nature 551, 75–79. doi:10.1038/nature24303
Sneden, C., Cowan, J. J., and Gallino, R. (2008). Neutron-capture elements in the early galaxy. Annu. Rev. Astron. Astrophys. 46, 241–288. doi:10.1146/annurev.astro.46.060407.145207
Soares-Santos, M., Holz, D. E., Annis, J., Chornock, R., Herner, K., Berger, E., et al. (2017). The electromagnetic counterpart of the binary neutron star merger LIGO/virgo GW170817. I. Discovery of the optical counterpart using the dark energy camera. Astrophys. J. 848, L16. doi:10.3847/2041-8213/aa9059
Sprouse, T. M., Navarro Perez, R., Surman, R., Mumpower, M. R., McLaughlin, G. C., and Schunck, N. (2020). Propagation of statistical uncertainties of Skyrme mass models to simulations of r-process nucleosynthesis. Phys. Rev. C 101, 055803. doi:10.1103/PhysRevC.101.055803
Surman, R., Beun, J., McLaughlin, G. C., and Hix, W. R. (2009). Neutron capture rates nearA=130that effect a global change to ther-process abundance distribution. Phys. Rev. C045809 79. doi:10.1103/PhysRevC.79.045809
Surman, R., Engel, J., Bennett, J. R., and Meyer, B. S. (1997). Source of the rare-earth element peak inr-process nucleosynthesis. Phys. Rev. Lett. 79, 1809–1812. doi:10.1103/PhysRevLett.79.1809
Surman, R., Mumpower, M., Sinclair, R., Jones, K. L., Hix, W. R., and McLaughlin, G. C. (2014). Sensitivity studies for the weak r process: Neutron capture rates. AIP Adv. 4, 041008. doi:10.1063/1.4867191
Tanaka, M., Utsumi, Y., Mazzali, P. A., Tominaga, N., Yoshida, M., Sekiguchi, Y., et al. (2017). Kilonova from post-merger ejecta as an optical and near-Infrared counterpart of GW170817. Publ. Astronomical Soc. Jpn. 69, 102. doi:10.1093/pasj/psx121
Tanvir, N. R., Levan, A. J., González-Fernández, C., Korobkin, O., Mandel, I., Rosswog, S., et al. (2017). The emergence of a lanthanide-rich kilonova following the merger of two neutron stars. Astrophys. J. 848, L27. doi:10.3847/2041-8213/aa90b6
Van der Swaelmen, M., Viscasillas Vázquez, C., Cescutti, G., Magrini, L., Cristallo, S., Vescovi, D., et al. (2022). The gaia-ESO survey: Placing constraints on the origin of r-process elements. arXiv e-prints. arXiv:2207.14747. doi:10.48550/arXiv.2207.14747
Villar, V. A., Guillochon, J., Berger, E., Metzger, B. D., Cowperthwaite, P. S., Nicholl, M., et al. (2017). The combined ultraviolet, optical, and near-infrared light curves of the kilonova associated with the binary neutron star merger GW170817: Unified data set, analytic models, and physical implications. Astrophys. J. 851, L21. doi:10.3847/2041-8213/aa9c84
Wanajo, S. (2018). Physical conditions for the r-process. I. Radioactive energy sources of kilonovae. Astrophys. J. 868, 65. doi:10.3847/1538-4357/aae0f2
Watson, D., Hansen, C. J., Selsing, J., Koch, A., Malesani, D. B., Andersen, A. C., et al. (2019). Identification of strontium in the merger of two neutron stars. nature 574, 497–500. doi:10.1038/s41586-019-1676-3
Wollaeger, R. T., Korobkin, O., Fontes, C. J., Rosswog, S. K., Even, W. P., Fryer, C. L., et al. (2018). Impact of ejecta morphology and composition on the electromagnetic signatures of neutron star mergers. Mon. Not. R. Astron. Soc. 478, 3298–3334. doi:10.1093/mnras/sty1018
Wu, M. R., Barnes, J., Martínez-Pinedo, G., and Metzger, B. D. (2019). Fingerprints of heavy-element nucleosynthesis in the late-time lightcurves of kilonovae. Phys. Rev. Lett. 122, 062701. doi:10.1103/PhysRevLett.122.062701
Wu, M. R., Fernández, R., Martínez-Pinedo, G., and Metzger, B. D. (2016). Production of the entire range of r-process nuclides by black hole accretion disc outflows from neutron star mergers. Mon. Not. R. Astron. Soc. 463, 2323–2334. doi:10.1093/mnras/stw2156
Zhu, Y., Wollaeger, R. T., Vassh, N., Surman, R., Sprouse, T. M., Mumpower, M. R., et al. (2018). Californium-254 and kilonova light curves. Astrophys. J. 863, L23. doi:10.3847/2041-8213/aad5de
Keywords: neutron stars, compact binary mergers, gravitational waves, multimessenger astrophysics, kilonovae, r-process, neutron - nuclear reactions
Citation: Vescovi D, Reifarth R, Cristallo S and Couture A (2022) Neutron-capture measurement candidates for the r-process in neutron star mergers. Front. Astron. Space Sci. 9:994980. doi: 10.3389/fspas.2022.994980
Received: 15 July 2022; Accepted: 08 September 2022;
Published: 07 October 2022.
Edited by:
Samuel Andrea Giuliani, Universidad Autónoma de Madrid, SpainReviewed by:
Athanasios Psaltis, Darmstadt University of Technology, GermanyIgor Panov, Kurchatov Institute, Russia
Copyright © 2022 Vescovi, Reifarth, Cristallo and Couture. This is an open-access article distributed under the terms of the Creative Commons Attribution License (CC BY). The use, distribution or reproduction in other forums is permitted, provided the original author(s) and the copyright owner(s) are credited and that the original publication in this journal is cited, in accordance with accepted academic practice. No use, distribution or reproduction is permitted which does not comply with these terms.
*Correspondence: Diego Vescovi, dmVzY292aUBpYXAudW5pLWZyYW5rZnVydC5kZQ==