- Swedish Institute of Space Physics, Uppsala, Sweden
We argue that many studies in space physics would benefit from putting a detailed investigation into a wider perspective. Three examples of theoretical and observational studies are given. We argue that space physics should aim to be less of an isolated branch of science. Rather, by putting the scientific space results into a wider perspective these results will become more interesting and important than ever. We argue that diversity in a team often is favourable for work on complicated problems and helps to present the results in a wider perspective.
1 Introduction
To be useful now and in the future, space research must be performed in the greatest possible detail, and must be presented in the widest possible content. This is obvious advice for many areas of science. But space can in the short run be more spectacular than other areas of research. Thus, it is important to realize that results from your own narrow area of research in the long run should not be presented only in a detailed and limited way. A limited presentation is not enough to be useful to your fellow scientists, to society, and is not enough to attract attention and funding.
Space physics can remain as a separate science in terms of some special techniques, including launchers, spacecraft technology and scientific instruments for extreme conditions. Space science should aim to be less of an isolated academic research topic (and so should several other topics now treated as individual subjects). Space results should be presented in a way that make them as useful as possible for other basic science disciplines such as astrophysics, astrobiology and laboratory plasma physics and for applied sciences such as space weather, spacecraft interaction with the surrounding environment and thermonuclear fusion. This can then lead to applications in areas we have not even thought of today.
As a start, space research should aim at results with a wide impact within this area of research. Keeping this wider perspective during each study makes the ongoing research more interesting, and the results will be more useful to a wider community. Below I give examples of three studies where I performed detailed science, and tried to present the results in a wide perspective.
2 Examples of Studies With a Perspective
To qualify as a study putting results into perspective, an investigation should include new results and new understanding, should put things together in a new way, and should still be interesting 10 years after publication. The last requirement is somewhat arbitrary but has the advantage that it can be tested by checking if other scientists are using a relevant publication as a reference after several years. Rather than attempting a stricter definition of studies with a perspective I give three examples of investigations I considered nice at the time, and which I am still proud of.
2.1 Dispersion Surfaces
The first example concerns the theory of plasma waves in a non-relativistic, collisionless, homogeneous and magnetized plasma. This is a good first approximation for the study of many plasmas in space physics and astrophysics. In collisionless plasmas energy is not transferred between charged particles via Coloumb collsions. Rather, the charged particles interact via electromagnetic waves. Often there is a need to identify which wave mode is observed. The relevant equations are complicated but can be found in textbooks, e.g., Chen (2016). Books and many research papers also give plots illustrating special cases such as a limited range of frequencies and wave-vectors k only parallel or perpendicular to the background magnetic field. As a student, my supervisor developed a computer code to study instabilities of waves in a homogeneous, anisotropic and magnetized plasma, WHAMP (Rönnnmark, 1982; Rönnmark, 1983), while I did a lot of testing and debugging. As part of my studies, I wanted to sort out which (not heavily damped) waves exist for a certain combination of plasma parameters.
Dispersion surfaces, plots of frequency as a function of wave vector for all directions of k (Oakes et al., 1979), are a useful way to display the numerical results. A systematic presentation of dispersion surfaces (André, 1985) was part of my thesis. Figure 1 shows an example of surfaces, covering frequencies up to three times the proton gyrofrequency. Wave modes labeled with capital letters are often treated as individual and isolated approximations. Here it is clear that they are smoothly connected as k is gradually changed. These surfaces have turned out to be very useful and are included in overviews and books (e.g., Benz (2002); Koskinen (2011)) and recent PhD theses (e.g., Allison (2019)) The point is not so much an individual wave mode represented by a small part of a surface and well described by an analytical approximation in a textbook. The point is more the overall picture of all possible wave modes, showing which wave modes should be considered. Putting the numerical solutions together in this way gives a new and useful perspective.
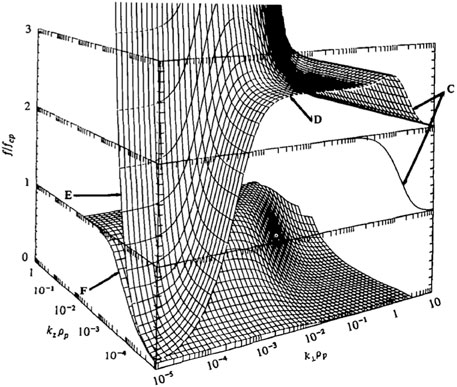
FIGURE 1. Dispersion surfaces, wave frequency shown as a function of wavevector components. Here f and fcp are the wave frequency and the proton gyrofrequency, k⊥ and k∥ are the wavevector components perpendicular and parallel to the background magnetic field and ρp is the proton gyroradius. Wave modes include: C) Ion Bernstein waves (also called Ion Cyclotron Harmonic, ICH, waves), D) Lower hybrid waves, E) Right circularly polarized whistler waves (fast magnetosonic or compressional Alfvén waves), F) Left circularly polarized shear Alfvén waves (electromagnetic ion cyclotron, EMIC, mode). From André (1985), where details of the plasma model are given.
2.2 Ion Energization
The second example concerns the energization of ions leaving the ionosphere. A large fraction of the plasma in the magnetosphere originate in the ionosphere, with initial energies of less than one electron volt. Ions such as H+ and O+ leaving the auroral regions can then be energized to several keV. In addition to upward acceleration of positive ions parallel to the geomagnetic field by the potential drops accelerating electrons downward to cause auroras, ions are often energized in the perpendicular direction. When the ions have left the lower ionosphere dominated by collisions, this heating can be caused by various electromagnetic waves, ultimately powered by energy from the solar wind. These ions may then move adiabatically up the field lines of the inhomogeneous terrestrial magnetic field and form so-called conics in ion velocity space. There are many observations by sounding rockets and satellites of ion conics and associated waves from altitudes of a few hundred km out to several Earth radii. Possible perpendicular energization mechanisms range from nearly static electric field structures, waves below and around the ion gyrofrequency, and waves near the lower hybrid frequency. Many studies have tried to identify the important mechanism. It was clear that more than one process may be acting, but at least for specific regions in space the search was usually for the (only) mechanism.
Having the opportunity to look at a lot of data from the Swedish Freja satellite launched 1992 to investigate the auroral region, I realized that another question would be more interesting. What is the relative importance of different mechanisms (wave modes)? Together with my PhD student Patrik Norqvist and other colleagues, we performed a statistical study using Freja observations close to the apogee of 1700 km, including more than 200 events of simultaneously observed ion conics and waves (André et al., 1998). We found that O+ energization was mainly caused by broadband waves, where the frequencies around the gyrofrequency were relevant for resonant heating. Sometimes waves around half the proton gyryfrequency (EMIC emissions) or waves around the lower hybrid frequency were more important. We used test particle calculations to verify that the observed wave amplitudes were high enough to explain the observed ion energies. We also considered and disregarded other possible mechanisms of perpendicular ion energization. We found that many previous studies were correct in that they had identified a plausible mechanism for a specific event. Our study included many events and showed that broadband waves overall cause most of the perpendicular ion energization in the auroral region, while other waves sometimes can be important. Considering several wave heating mechanisms in one single study gave a new and useful perspective.
2.3 Outflow of Ionospheric Low-Energy Ions
The third example concerns ions leaving the ionosphere without being much energized. Plasma in the magnetosphere can originate in either the solar wind or the ionosphere, and during decades it gradually became clear that the ionosphere can be an important source also at high altitudes (Chappell et al., 1980; Chappell, 2015). A problem in large regions of the magnetopshere is that a spacecraft in sunlight in a low-density plasma will be positively charged to tens of volts due to the emission of photoelectrons. Hence, positive ions from the ionosphere with eV energies will not reach the spacecraft. These ions often have a drift energy that is higher than the velocity distributions thermal energy. The upward flowing ions will be scattered by a large electrostatic structure around the charged satellite. This will cause an enhanced ion wake behind the spacecraft. For similar electron and ion temperatures the low mass electrons have much higher thermal velocities and immediately fill this wake, causing a region behind the spacecraft with an excess of electrons. The result is a local electric field caused by the combination of drifting low-energy ionospheric ions and the charged spacecraft. As PI with main responsibility for the Electric Field and Wave instruments on the four ESA Cluster satellites launched 2000, I first considered this local field a problem. The EFW instrument includes two pairs of probes on wire booms in the spin plane of each satellite. Each pair has a probe-to-probe separation of 88 m and the electric field is obtained from the potential difference between the probes.
Once we understood that the ion wake is the cause of the local electric field, Anders Eriksson and his PhD student Erik Engwall started to develop a method to use this local field to estimate the flux of outflowing ions (Engwall et al., 2006a; Engwall et al., 2006b; Engwall et al., 2009b). The trick is to use also the Electron Drift Instrument on each spacecraft, measuring the drift of artificially emitted electrons as they gyrate back to the spacecraft under the influence of the geophysical magnetic field measured by the FluxGate Magnetometer FGM. These electrons with an energy of about a keV have large gyroradii and are mot much affected by the local wake. Drifting low-energy ions can then be inferred by detecting a wake electric field, obtained as a large enough difference between the quasi-static electric fields obtained by the EFW (total electric field) and EDI (geophysical electric field) instruments. The direction of the wake gives the direction of the ion outflow. Since the perpendicular E × B drift velocity is known (from EDI and FGM) the parallel velocity can be inferred (Engwall et al., 2009a; André et al., 2015). The density can be obtained by calibrating observations of the spacecraft potential, in practise obtained as the potential difference between the EFW probes (nearly at the plasma potential) and the spacecraft (Lybekk et al., 2012; Haaland et al., 2017). The ion flux can then be obtained from the drift velocity and the density. The first important perspective in this example is to seriously try to understand observations which at first seem peculiar or just wrong. In this case two instruments observing the same parameter, the electric field, gave different results. Rather than deciding that at least one observation must be wrong, understanding of the situation shows that both are correct, one showing the local field around the charged spacecraft (EFW) and the other the geophysical field present in large regions (EDI). This wider perspective then gives the possibility to estimate a parameter thought not to be possible to measure, the flux of ionospheric low-energy ions.
The Cluster wake method to estimate the flux of outflowing ionospheric ions has then been used to improve the map of plasma in the magnetosphere. Together with Chris Cully I made a study resulting in the overview of low-energy ions in Figure 2 (André and Cully, 2012). The night-side polar lobe results are from the Engwall et al. (2009a) investigation using the wake method. For the dayside we used this method together with other estimates. Sometimes ions with low thermal velocity have a large enough E × B drift to be detected by an onboard ion instrument also on a charged spacecraft. We also compared total density obtained from wave observations with particle instrument observations, to estimate how much of the ion population was low-energy and hidden from direct detection. This overall picture has been confirmed by a larger statistical study using 10 years of data and the wake method in the polar lobes (André et al., 2015). Also, this method has been validated in different ways such as using a similar method in the solar wind and comparing with data from particle instruments at lower altitude where spacecraft charging is less of a problem (André et al., 2021b,a). Comparing with a review of many observations, the overview in Figure 2 is still useful (Toledo-Redondo et al., 2021). Previously, many of the low-energy ions of ionospheric origin could not be detected. Putting observations by several instruments and methods together in an overview such as the one in Figure 2 gives a new and useful perspective on the magnetosphere.
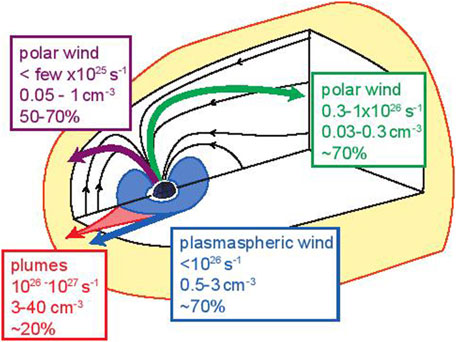
FIGURE 2. Overview of ion outflow from Earth. Regions dominated by low-energy (eV) plasma of ionospheric origin are indicated. Order of magnitude outflow rates, densities and percentage of time low-energy ions dominate the density are given. From André and Cully (2012).
For all three examples above, some theory or data have been added to previous knowledge. But the most important part is the new overall picture. How different wave modes with different names are related, concluding that several waves can energize ions in the auroral region but one type is typically more important, and realizing that low energy ions of ionospheric origin are not just sporadically detected at high altitudes but are a very common component of the magnetospheric plasma.
3 The Scientist With a Perspective
The successful space scientist is intelligent and ambitious, in some wide meaning of these words. But also the surrounding family, society and culture have significant impact on who becomes a great scientist (Gladwell, 2009). Extrovert entrepreneurs are often sought after to lead science projects, while it is clear that introvert and quiet people can be equally good scientists and leaders (Cain, 2013). For complicated (science) problems that takes time to solve, it seems that a group of people with mixed skills and backgrounds is often successful. Different skills can include, for example, expertise in instrument design, data analysis and numerical simulations. Members of the group can differ, for example, concerning gender, ethnic background and age. Diversity in a team is not without problems but I think the positive aspects dominate (Carter and Phillips, 2017; Stangor, 2017; Peters, 2021). This is one reason to achieve roughly equal numbers of female and male scientists at all academic ranks (Coe et al., 2019; Popp et al., 2019). A diverse team is more likely to keep a wider perspective.
4 Conclusion
The work on a complicated problem usually becomes more interesting if a wider perspective can be kept during the work. The result becomes more useful to fellow scientists and to society when it is presented in a wider perspective. Diversity in the team can help to keep this wider perspective.
Data Availability Statement
The original contributions presented in the study are included in the article/supplementary material, further inquiries can be directed to the corresponding author.
Author Contributions
The author confirms being the sole contributor of this work and has approved it for publication.
Funding
MA was supported by the Swedish National Space Agency contract SNSA 2020-00058.
Conflict of Interest
The author declares that the research was conducted in the absence of any commercial or financial relationships that could be construed as a potential conflict of interest.
Publisher’s Note
All claims expressed in this article are solely those of the authors and do not necessarily represent those of their affiliated organizations, or those of the publisher, the editors and the reviewers. Any product that may be evaluated in this article, or claim that may be made by its manufacturer, is not guaranteed or endorsed by the publisher.
Acknowledgments
Useful discussions concerning the main ideas of this article with Susanne Höfner are acknowledged. Helpful discussions concerning references and related questions with Gabriella Stenberg Wieser and Kristina Andréen are acknowledged.
References
Allison, H. J. (2019). Understanding How Low Energy Electrons Control the Variability of the Earth’s Electron Radiation Belts (UK: University of Cambridge). Ph.D. thesis.
André, M., and Cully, C. M. (2012). Low-energy Ions: A Previously Hidden Solar System Particle Population. Geophys. Res. Lett. 39, a–n. doi:10.1029/2011GL050242
André, M., Eriksson, A. I., Khotyaintsev, Y. V., and Toledo‐Redondo, S. (2021a). The Spacecraft Wake: Interference with Electric Field Observations and a Possibility to Detect Cold Ions. JGR Space Phys. 126, e29493. doi:10.1029/2021JA029493
André, M., Li, K., and Eriksson, A. I. (2015). Outflow of Low-Energy Ions and the Solar Cycle. J. Geophys. Res. Space Phys. 120, 1072–1085. doi:10.1002/2014JA020714
André, M., Norqvist, P., Andersson, L., Eliasson, L., Eriksson, A. I., Blomberg, L., et al. (1998). Ion Energization Mechanisms at 1700 Km in the Auroral Region. J. Geophys. Res. 103, 4199–4222. doi:10.1029/97JA00855
André, M., Toledo-Redondo, S., and Yau, A. W. (2021b). “Cold Ionospheric Ions in the Magnetosphere,” in Magnetospheres in the Solar System, Geophysical Monograph 259. Editors R. Maggiolo, N. André, H. Hasegawa, and D. T. Welling (Washington, DC: John Wiley & Sons), 219–228. doi:10.1002/978111950751210.1002/9781119815624.ch15
Benz, A. (2002). Plasma Astrophysics, Kinetic Processes in Solar and Stellar Coronae. second edition, 279. New York: Kluwer Academic Publishers. doi:10.1007/978-0-306-47719-5
Cain, S. (2013). Quiet: The Power of Introverts in a World that Can’t Stop Talking. New York: Random House. isbn: 978-0-307-35215-6.
Carter, A. B., and Phillips, K. W. (2017). The Double-Edged Sword of Diversity: Toward a Dual Pathway Model. Soc. Personal. Psychol. Compass 11, e12313. doi:10.1111/spc3.12313
Chappell, C. R., Baugher, C. R., and Horwitz, J. L. (1980). New Advances in Thermal Plasma Research. Rev. Geophys. 18, 853–861. doi:10.1029/RG018i004p00853
Chappell, C. R. (2015). The Role of the Ionosphere in Providing Plasma to the Terrestrial Magnetosphere - an Historical Overview. Space Sci. Rev. 192, 5–25. doi:10.1007/s11214-015-0168-5
Chen, F. F. (2016). Introduction to Plasma Physics and Controlled Fusion. New York: Springer. doi:10.1007/978-3-319-22309-4
Coe, I. R., Wiley, R., and Bekker, L.-G. (2019). Organisational Best Practices towards Gender Equality in Science and Medicine. Lancet 393, 587–593. doi:10.1016/S0140-6736(18)33188-X
Engwall, E., Eriksson, A. I., André, M., Dandouras, I., Paschmann, G., Quinn, J., et al. (2006a). Low-energy (Order 10 eV) Ion Flow in the Magnetotail Lobes Inferred from Spacecraft Wake Observations. Geophys. Res. Lett. 33, L06110. doi:10.1029/2005GL025179
Engwall, E., Eriksson, A. I., Cully, C. M., André, M., Puhl-Quinn, P. A., Vaith, H., et al. (2009a). Survey of Cold Ionospheric Outflows in the Magnetotail. Ann. Geophys. 27, 3185–3201. doi:10.5194/angeo-27-3185-2009
Engwall, E., Eriksson, A. I., Cully, C. M., André, M., Torbert, R., and Vaith, H. (2009b). Earth’s Ionospheric Outflow Dominated by Hidden Cold Plasma. Nat. Geosci. 2, 24–27. doi:10.1038/ngeo387
Engwall, E., Eriksson, A. I., and Forest, J. (2006b). Wake Formation behind Positively Charged Spacecraft in Flowing Tenuous Plasmas. Phys. Plasmas 13, 062904062904. doi:10.1063/1.2199207
Gladwell, M. (2009). Outliers: The Story of Success. New York: Penguin Books. isbn: 978-0-141-04302-9.
Haaland, S., Lybekk, B., Maes, L., Laundal, K., Pedersen, A., Tenfjord, P., et al. (2017). North-south Asymmetries in Cold Plasma Density in the Magnetotail Lobes: Cluster Observations. J. Geophys. Res.Space Phys. 122, 136–149. doi:10.1002/2016JA023404
Koskinen, H. E. J. (2011). Physics of Space Storms: From the Solar Surface to the Earth. New York: Springer. doi:10.1007/978-3-642-00319-6
Lybekk, B., Pedersen, A., Haaland, S., Svenes, K., Fazakerley, A. N., Masson, A., et al. (2012). Solar Cycle Variations of the Cluster Spacecraft Potential and its Use for Electron Density Estimations. J. Geophys. Res. (Space Phys. 117, A01217. doi:10.1029/2011JA016969
Oakes, M. E., Michie, R. B., Tsui, K. H., and Copeland, J. E. (1979). Cold Plasma Dispersion Surfaces. J. Plasma Phys. 21, 205–224. doi:10.1017/S0022377800021784
Peters, U. (2021). Hidden Figures: Epistemic Costs and Benefits of Detecting (Invisible) Diversity in Science. Eur. J. Philosophy Sci. 11, 33. doi:10.1007/s13194-021-00349-6
Popp, A. L., Lutz, S. R., Khatami, S., van Emmerik, T. H. M., and Knoben, W. J. M. (2019). A Global Survey on the Perceptions and Impacts of Gender Inequality in the Earth and Space Sciences. Earth Space Sci. 6, 1460–1468. doi:10.1029/2019EA000706
Rönnmark, K. (1983). Research Note: Computation of the Dielectric Tensor of a Maxwellian Plasma. Plasma Phys. 25, 699–701. doi:10.1088/0032-1028/25/6/007
Rönnnmark, K. (1982). WHAMP - Waves in Homogeneous, Anisotropic, Multicomponent Plasmas, 179. Kiruna: Kiruna Geophysical Institute. Tech. Rep.
Stangor, C. (2017). Group Processes. Oxford: Oxford University Press. doi:10.1093/acrefore/9780190236557.013.255
Keywords: diversity, dispersion surfaces, ion energization, ionospheric outflow, low-energy ions, spacecraft charging
Citation: André M (2022) Space Physics: The Need for a Wider Perspective. Front. Astron. Space Sci. 9:937742. doi: 10.3389/fspas.2022.937742
Received: 06 May 2022; Accepted: 27 May 2022;
Published: 17 June 2022.
Edited by:
Jorge Luis Chau, Leibniz Institute of Atmospheric Physics (LG), GermanyReviewed by:
Huishan Fu, Beihang University, ChinaCopyright © 2022 André. This is an open-access article distributed under the terms of the Creative Commons Attribution License (CC BY). The use, distribution or reproduction in other forums is permitted, provided the original author(s) and the copyright owner(s) are credited and that the original publication in this journal is cited, in accordance with accepted academic practice. No use, distribution or reproduction is permitted which does not comply with these terms.
*Correspondence: Mats André, bWF0cy5hbmRyZUBpcmZ1LnNl