- 1UK Center for Astrobiology, University of Edinburgh, Edinburgh, United Kingdom
- 2University of Florida, Plant Pathology Department, Space Life Sciences Lab, Exploration Park, Merritt Island, FL, United States
- 3Laboratory for Astrophysics, Leiden Observatory, Leiden University, Leiden, Netherlands
- 4Life Support and Physical Sciences Instrumentation Section, European Space Agency, Nordwijk, Netherlands
- 5Space Policy Institute, George Washington University, Washington, DC, United States
- 6German Aerospace Center (DLR), Institute of Aerospace Medicine, Radiation Biology Department, Research Group Astrobiology, Köln, Germany
- 7Centre de Biophysique Moléculaire, Centre National de la Recherche Scientifique (CNRS), Orléans, France
- 8Medical University Graz, Diagnostic and Research Institute of Hygiene, Microbiology and Environmental Medicine, Graz, Austria
- 9Centro de Astrobiología (CSIC-INTA), Madrid, Spain
- 10CBMSO, Madrid, Spain
- 11Matis Ohf, Microbiology Group, Department of Research and Innovation, Faculty of Food Science and Nutrition, University of Iceland, Reykjavik, Iceland
- 12European Science Foundation (ESF), Strasbourg, France
The identification of reliable biomarkers, such as amino acids, is key for the search of extraterrestrial life. A large number of microorganisms metabolize, synthesize, take up and excrete amino acids as part of the amino acid metabolism during aerobic and/or anaerobic respiration or in fermentation. In this work, we investigated whether the anaerobic microbial metabolism of amino acids could leave a secondary biosignature indicating biological activity in the environment around the cells. The observed fingerprints would reflect the physiological capabilities of the specific microbial community under investigation. The metabolic processing of an amino acid mixture by two distinct anaerobic microbial communities collected from Islinger Mühlbach (ISM) and Sippenauer Moor (SM), Germany was examined. The amino acid mixture contained L-alanine, β-alanine, L-aspartic acid, DL-proline, L-leucine, L-valine, glycine, L-phenylalanine and L-isoleucine. In parallel, an amino acid spiked medium without microorganisms was used as a control to determine abiotic changes over time. Liquid chromatography mass spectrometry (LC-MS) was used to track amino acid changes over time. When comparing to the control samples that did not show significant changes of amino acids concentrations over time, we found that glycine was almost completely depleted from both microbial samples to less than 3% after the first two weeks- This results indicates a preferential use of this simple amino acid by these microbial communities. Although glycine degradation can be caused by abiotic processes, these results show that its preferential depletion in an environment would be consistent with the presence of life. We found changes in most other amino acids that varied between amino acids and communities, suggesting complex dynamics with no clear universal pattern that might be used as a signature of life. However, marked increases in amino acids, caused by cellular synthesis and release into the extracellular environment (e.g., alanine), were observed and could be considered a signature of metabolic activity. We conclude, that substantial anomalous enhancements of some amino acids against the expected abiotic background concentration may be an agnostic signature of the presence of biological processes.
Introduction
The search for signatures of extraterrestrial life, extinct or extant, is a key goal for the research field of astrobiology. One way to search for life is to seek the remains or products of biological processes (Hays et al., 2017). Examples include the fossil remains of single cells or communities (e.g., stromatolites; Grotzinger and Rothman, 1996), biologically processed minerals (Westall et al., 2011), stable isotope patterns unique for biological systems (House et al., 2000; Shen et al., 2001), gases that can be produced by biological processes (Seager et al., 2012), or complex biogenic organic molecules, such as carbohydrates, lipids, nucleic acids, amino acids, and proteins (Kvenvolden, 1973; Bada and McDonald, 1996; Deamer at al., 2002; Creamer et al., 2017).
Amino acids have previously been considered as a biosignature (Parnell et al., 2007; Hand et al., 2017; Georgiou, 2018) that may be preserved under certain conditions on planetary bodies for billions of years (Aerts et al., 2014). Amino acids are ubiquitous in life as main components of cells and, apart from glycine, which is not chiral, biological systems on Earth almost exclusively use the L enantiomeric form. However, some D-amino acids can be found in the cell membranes of bacteria (Kaiser and Benner, 2008; Lam et al., 2009). Evidence has been provided that indicates enantiomeric excess of certain amino acids in meteorites (Busemann et al., 2006; Glavin et al., 2010), but at ∼9% or less. Consequently, the ratio of the enantiomers of amino acids was proposed as a signature of life, with terrestrial life showing an enantiomeric excess of L-amino acids (Avnir, 2021). Although only twenty core amino acids are found in Earth organisms (proteogenic amino acids), there are several hundred known non-proteogenic amino acids (Gutiérrez-Preciado et al., 2010; Fichtner et al., 2017; Souza-Correa et al., 2019). Therefore, the mere presence of amino acids in an extraterrestrial sample does not indicate a signature of life and cannot be used as a biomarker itself (Parnell et al., 2007; Bada and McDonald, 2996). Nevertheless, the abundance of certain amino acids in a sample might provide clues on the presence of microorganisms. While abiotic processing of amino acids is driven by thermodynamic processes, in biological systems their relative abundance is the result of metabolic activity in any given organism or a community (Davila and McKay, 2014). In addition, life uses only a selection of amino acids, while extraterrestrial carbonaceous matter contains, as noted above, a much broader variety.
One method to investigate the presence of life, distinct from looking for the amino acids in life itself (e.g., within a microbial cell), might be to study the effects of microbiota on the abundance of amino acids expected to be present in an environment, and distinguish these patterns from abiotic sources, such as those delivered by meteoritic material (Summons et al., 2008).
In many extraterrestrial environments, including Mars, we would expect amino acids in addition to other organic compounds to be available to any putative biota. They are delivered to a planetary surface in carbonaceous chondrite meteorites (Cronin and Pizzarello 1983; Ehrenfreund et al., 2001a; Martins et al., 2007a; Cleaves et al., 2008; Elsila et al., 2016).
For example, a wide range of amino acids has been detected in carbonaceous chondrites. In the Murchison meteorite, amino acids are found at 10–60 μg g−1 meteorite (Sephton, 2002). The amino acids in carbonaceous meteorites are part of the soluble fraction that make up ∼30% of organic carbon in these materials. The frequency of the prebiotic synthesis of amino acids and their abundances follow thermodynamic principles with the chemically simple compounds being most abundant (Miller, 1993; Pizzarello, 2006; Pizzarello and Shock, 2010; Glavin et al., 2012).
A large number of microorganisms can use amino acids as electron donors for anaerobic respiration or in fermentation (Nixon et al., 2012). In this process, they degrade the amino acid molecules. Microorganisms are unlikely to degrade amino acids at the same rate compared to degradation by abiotic processes. Rather, they will degrade the molecules according to their metabolic pathways, the accessibility of certain amino acids, the availability of other metabolizable organic compounds, and other organism-specific effects. Thus, we could hypothesize that, in the process of degrading abiotic amino acids, microorganisms would leave a biosignature by the preferential degradation of certain amino acids in the environment around them. This biosignature might be superimposed on the biosignature of the amino acids in the organism itself and that were synthesized by the organism, but it would be a distinct and additional biosignature reflecting non-random biological destruction of the abiotic amino acid pool. One attraction of such a biosignature is that, if cells alter the amino acid concentration in the environment around them, then, particularly in low biomass environments, that signature might be much more pervasive and easier to detect than the amino acid signature of the cells themselves, which could be highly localized and poorly preserved. Furthermore, although this signature would assume the presence of amino acid-using life, the decrease (or increase) in any amino acids away from the expected background abiotic concentration could be an agnostic signature of metabolic processes.
In this study, we tested this hypothesis by investigating the metabolic usage of seven amino acids previously detected in both terrestrial environments and Martian meteorites by two distinct anaerobic microbial communities from Martian analog environments. We used two distinct communities to determine if there were common patterns of degradation of certain amino acids that could potentially suggest a universal signature of amino acid degradation by life. The almost complete degradation of glycine was common to both communities. For other amino acids, we observed different patterns of degradation with increased extracellular concentrations of some amino acids. We discuss the implications of these findings for life detection.
Materials and Methods
Sampling Site
The samples investigated were collected in the frame of the MASE (Mars Analogs for Space Exploration) project, a 4-year collaborative research project supported by the European Commission Seventh Framework Contract. The aim of the project was to characterize Mars analogue environments on Earth with regard to habitability and the search for potential biosignatures of extraterrestrial environments (Cockell et al., 2017). Samples analyzed herein were collected from two sulfidic springs close to Regensburg, Germany, where many sulfide-containing springs emanate from Mesozoic karst formations. The two springs, in the Sippenauer Moor (SM) and Islinger Mühlbach (ISM) areas (48.8685° N; 11.9563° E and 48.9857° N; 12.1272° E, respectively) are characterized by a main sulfidic spring, emanating into a streamlet where whitish mats of sulfide-oxidizing bacteria cover the submerged surfaces. The sites SM and ISM are independent and not connected in the deep subsurface. Detailed analyses of the site microbiomes are already available (Moissl et al., 2002; Rudolph et al., 2004; Probst et al., 2014). All samples were taken under anoxic conditions (for a detailed description see Cockell et al., 2017) and an anoxic atmosphere was maintained throughout.
Cultivation
Cultivation was performed under anoxic conditions. Samples from SM and ISM were inoculated in anoxic MASE medium II and supplemented with a mixture of proteogenic and non-proteogenic amino acids. MASE II medium contains per liter: NH4Cl 0.1 g, NaHCO3 0.2 g, KH2PO4 0.06 g, MgSO4 × 7 H2O 0.2 g, CaCl2 × 2 H2O 0.1 g, FeCl2 0.023 g, selenite-tungstate solution 4 ml, 10 × Wolfe’s minerals 1 ml, 10 × Wolfe’s vitamins 1 ml, cysteine-HCl 0.5 g (Cockell et al., 2017). The medium was portioned (20 ml each) into 120 ml serum bottles and gassed with 1 bar N2/CO2 (80/20 vol/vol) and autoclaved. Prior to inoculation, the medium was supplemented with a filter-sterilized amino acid broth. Amino acids such as alanine, aspartic acid, glutamic acid, glycine, leucine, serine, and valine are common in both biological samples and for example, carbonaceous chondrite meteorite (Cronin and Pizzarello, 1983; Shimoyama et al., 1985; Martins et al., 2007b; Martins et al., 2007c; Pizzarello and Holmes, 2009; Cobb and Pudritz, 2014). Based on these data, the following mixture of amino acids was added to the medium: glycine, L-alanine, β-alanine, L-aspartic acid, DL-proline, L-leucine, L-valine, L-phenylalanine and L-isoleucine (Table 1). The amino acid broth used in this study included some proteinogenic amino acids that are most likely not found in meteorites due to their complexity in synthesis. These are amino acids with a relative high molecular weight of >100 Da, such as phenylalanine. The final concentration of each added amino acid was 10 mM, and the pH was adjusted to 7.0. One millilitre of the environmental sample was added to 20 ml of medium and incubated at 30°C. A negative control (NC), i.e., no microbial sample added, was set up to determine abiotic changes of amino acids.
After defined time points, 1.5 ml of inoculated medium was withdrawn from the inoculum using a syringe and needle under aseptic conditions. The first sample was taken immediately after inoculation (T0), followed by samples after 7, 14, 28, 56, and 90 days of incubation.
The sample was sterilized using a 0.22 µm filter (polyethersulfone membrane, Fisher Scientific) to remove microbial cells and transferred into an Eppendorf tube and frozen at −80°C until further analysis.
Amino Acid Extraction
Amino acid extraction was performed using a simplified procedure described in Aerts et al. (2020) based on the protocol outlined in full detail in Botta and Bada. (2002). Therefore, the extraction protocol is described in the following only briefly. For sterilization, all glassware, including the columns with glass wool for amino acid extraction, were double wrapped in aluminum foil and placed into a furnace at 500°C for a minimum of 3 h.
Two-hundred μl of each microbe-filtered solution was pipetted into a sterile Eppendorf tube and dried in a centrifugal vacuum concentrator system overnight (CentriVap Centrifugal concentrator/ColdTrap system, LabConco) to remove the liquid media. Then, 3–4 ml of a resin suspension (100 ml ULC/MS grade water with 24 mg of AG®50W-X8 Resin, Analytical grade 100–200 mesh, Bio-Rad) was added to glass columns half-filled with glass wool used for the subsequent amino acid extraction. A sequential washing with basic-neutral-acid-basic solutions was made to activate the resin active sites. The basic solution was a 2 M sodium hydroxide solution (8 g of NaOH in 100 ml of ULC/MS pure grade water), and the acid solution was 1.5 M HCl (12.5 ml of 36% HCl in 87.5 ml of ULC/MS grade water). ULC/MS grade water was used for neutralisation after basic and acid washing, until a pH of about 7 was reached (detected by pH-indicator paper). After the sequential washing procedure, 1.5 ml of ULC/MS pure grade water was added to each dried sample. The sample was vortexTed at 2,800 rpm for 30 s and subsequently added to the column. Note, that this first elution was not collected for further analysis and was disposed. Five mL of a 2 M ammonia solution (ULC/MS grade water added to 15.3 ml of 25% ammonia for a final volume of 100 ml) was added subsequently to the columns to remove the amino acids bound to the resin; the eluent was collected using sterile glass tubes. The ammonia within the eluents was removed overnight using the CentriVap/Coldtrap system. The dried samples were either stored in a fridge or freezer or directly reconstituted in 100 µl ULC/MS grade water, representing amino acid sample solutions. For the LC-MS measurements, the sample solution concentration was too high (saturated the detector systems) and 1:50 dilutions were prepared using ULC/MS grade water for accurate analysis.
LC-MS Measurements
The system used for amino acid analysis is described in Aerts et al. (2020). Measurements were performed using an Agilent 1260 LC-MS system equipped with an ultraviolet (UV) and fluorescence (FL) detector system, an autosampler module where the amino acid derivatization is performed, and a 500-MS ion trap mass spectrometer with electrospray ionisation. The column used for analysis was a 150 × 3 mm 2.6 µm phenyl-hexyl stationary phase from Phenomenex, which was maintained at 25°C. The LC was operated in a binary gradient of mobile phases A (10 mM Ammonium Acetate in ULC/MS water) and B (ULC/MS grade Methanol). The MS was operated in positive mode with optimised conditions for each individual amino acid. Amino acids were derivatised using a method based on Nimura and Kinoshita (1986) which was then automated in order to increase the robustness of the method. This automation was achieved by programming the autosampler module (Agilent G1329B) of the HPLC to mix the various reagents. The approach used was as follows: the amino acid sample was mixed in a 1:3 ratio with 0.1 M sodium borate, this mixture was then mixed in 2:1 ratio with methanolic N-Acetyl-l-cysteine (NAC)/o-phthaldialdehyde (OPA) (8 mg OPA and 10 mg NAC in 1 ml methanol), this mixture was incubated for 2 min after which 2.5 μl was injected into the system. In a typical measurement run, amino acid samples from one time point (including negative control) were analysed sequentially, including wash procedures and the analysis of amino acid standard solutions (Agilent, part number: 5061–3332). Amino acids were identified by comparison to retention times of known standards as well as their associated m/z ratio for the derivatized molecules. Proline was not measurable as it cannot be derivatized and is therefore not detectable using the applied method. Standards were run at the beginning and end of each run in order to track reagent degradation and system performance. Note that the intensity measured for the amino acids at T0 for both sites (SM and ISM) was normalized to 100%. For the amino acids measured in the negative controls, the intensity is given relative to the mean, which is normalized to 100%. For each amino acid investigated in the NC the standard deviation was calculated (to the right in the panel of NC’s in Figures 1–3). The standard deviation was added as error bars to the measurements of the amino acids of SM and ISM.
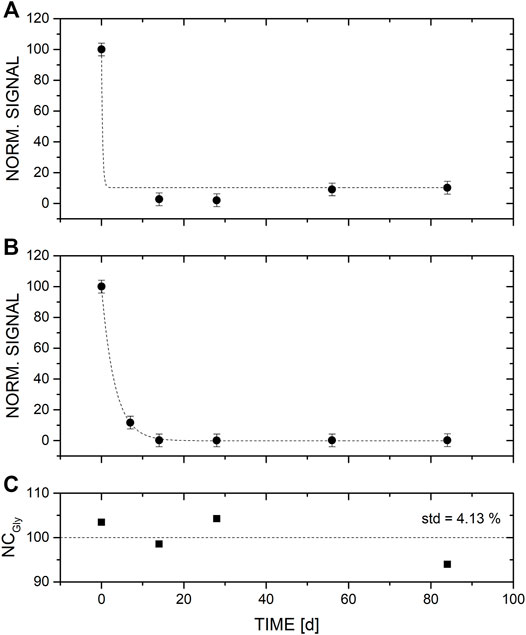
FIGURE 1. Degradation of glycine from the two different enrichments (A) Islinger Mühlbach (ISM), and (B) Sippenauer Moor (SM) spiked with a broth of amino acids (final concentration of each added amino acid was 10 mM) over a time of 3 months. (C) Negative control, i.e., medium spiked with the amino acid broth but no inoculum.
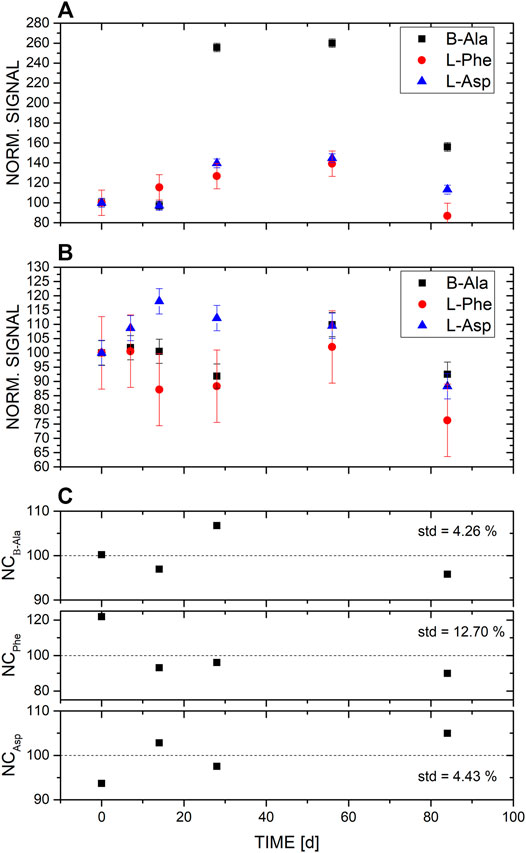
FIGURE 2. LC-MS measurements of the three amino acids β-alanine, L-aspartic acid, and L-phenylalanine from the two different enrichments over a time of 3 months. (A) Islinger Mühlbach (ISM), and (B) Sippenauer Moor (SM) spiked with a broth of amino acids (final concentration of each added amino acids was 10 mM). (C) Negative control, i.e., medium spiked with the amino acid broth but no inoculum.
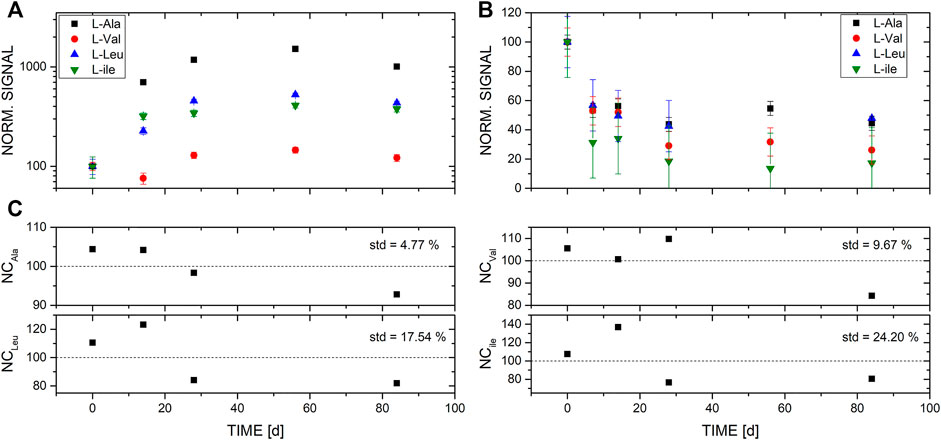
FIGURE 3. LC-MS measurements of the four amino acids L-alanine, L-valine, L-leucine, and L-isoleucine from the two different enrichments over a time of 3 months. (A) Islinger Mühlbach (ISM), and (B) Sippenauer Moor (SM) spiked with a broth of amino acids (final concentration of each added amino acid was 10 mM). (C) Negative control, i.e., medium spiked with the amino acid broth but no inoculum.
Results
Using LC-MS measurements, we investigated the differences in the amino acid distribution in the medium of two different microbial enrichments and the negative control. We found that in the control samples in which no microbiota was added, no significant changes of amino acid concentrations were observed over time (Figures 1–3). Therefore, the changes observed in the inoculated samples are attributed to microbial activity. Note, because the signal of measured amino acids in NC for time point 56 days was significantly lower in comparison to the signal of the NC of the other time points, NC of time point 56 days was not considered for analysis. No such decrease was observed for the measurements of amino acids in the SM and ISM samples of the same time point, which points to a sample problem and not to an instrument malfunction.
Similar Glycine Fingerprint in Both Enrichments
The results after analysis using LC-MS revealed that the quantities of the non-glycine amino acids varied over time depending on the microbial community and the amino acid (see Figures 1–3). Although the concentrations of amino acids varied between the two enrichments, we found one amino acid characteristic that was consistent with both enrichments (Figure 1). Glycine was almost completely depleted from both samples from 100% to less than 3% after the first 2 weeks. The depletion follows an exponential decay; see fit to the date in panel B) of Figure 1. Note, the same fit could not be applied to ISM1 data (panel A)) because of missing sample for time point 7 days; the fit would be too steep at the beginning. These data suggest a preferential use of glycine by these microbial communities.
Different Metabolic Fingerprints of Amino Acids From Two Enrichments
For all amino acids, we observed two different patterns of the measured relative ratios: 1) a differential use of amino acids was revealed, i.e., the amount of detectable amino acid decreased or increased over time, but the same trend was seen in both enrichments (Figures 1, 2) or 2) the trend in amino acid degradation in the two enrichments were opposed (Figure 3).
For amino acids measured in ISM, significantly increased values of several hundreds of % relative to T0 can be observed, with Ala exceeding even 1,000% relative to the measured concentration at inoculation (Figure 3A). In contrast, in the SM samples, the same set of amino acids shows a significant depletion down to levels of about 50% and below relative to the concentration measured at T0. Because the observed enhancements and depletion in amino acids cannot be explained with the measurements conducted on the negative controls (scatter around 100%), the significant changes in concentrations relative to T0 are the result of the microbial activity of the respective microbial community.
B-ala, L-asp, and L-phe did not reveal a clear trend with time (Figure 2). For example, in the ISM inoculum, the amount of β-ala and L-asp decreased over the first 2 weeks, followed by a peak (2.5 × increase for β-ala; 1.5 × increase for L-asp) which remained stable until a decrease was measured on day 90 (Figure 2A). In the SM inoculum, L-asp increased over the first three measurements before a steady, but small decrease was observed. In contrast, for β-ala the initial decrease was prolonged, before a peak followed by a decrease (Figure 2B). L-phe followed a similar trend in the SM sample (Figure 2B), but it was less prevalent in the ISM sample (Figure 2A). However, these trends were not significant.
The measured amount of amino acids L-ala, L-val, L-leu, and L-ile in the media increased in IM enrichment compared to the observed decrease in the SM enrichment. All four amino acids in the IM enrichment followed a similar pattern: Within the first 3 weeks an increase was detected followed by a plateau phase (Figure 3A). The largest increase was seen for L-ala whereas only a small increase was detected for L-val. While in the SM sample, L-ile decreased the most and L-ala and L-leu were less depleted from the media, the plateau phase also started after about 2 weeks, revealing relatively small changes of the amino acid abundance (Figure 3B).
Discussion
This study investigated whether the fingerprints of microbial amino acid metabolism could be used as a potential biosignature.
Our approach was to identify fingerprints that are characteristic of a microbial community’s catalytic speed and/or selectivity as evidence of biogenicity. Beside a preferential use of amino acids, the microbial community can release certain amino acids as metabolic products into their surroundings. The release can occur by excretion or passive diffusion or the result of cell death followed by cell lysis. In addition, a variety of abiotic processes leading to the formation or degradation of certain amino acids can result in a change of prevalent amino acid abundance within an environment.
The following discussion is based on the assumption that potential extraterrestrial life uses similar biochemistry in liquid water environments as observed for Earth-based life. Life as we know it is based on mainly CHNOPS elements and other mineral sources for generating energy and the usage of amino acids to form proteins.
The Absence of Glycine as a Potential Biosignature
The data obtained for glycine suggest a preferential use of glycine by microbial communities. We found that almost all the glycine was depleted and we observed this for both communities, suggesting the possibility that glycine depletion in an environment would be consistent with life.
There are several mechanisms in bacteria involved in glycine uptake and metabolism (Sagers and Gunsalus, 1961; Andreesen, 1994). In anoxic environments, glycine can be a substrate in the Stickland reaction, which is a coupled oxidation-reduction reaction mainly for amino acid pairs (Andreesen, 1994). Glycine serves preferentially as an electron acceptor which can be coupled to an energy conservation step. Glycine and alanine can act as a redox couple in which glycine is reduced while alanine is oxidized. This reaction would also lead to a decrease in alanine, which is observed for the Sippenauer Moor (SM) sample (Figure 2B) but not for the Islinger Mühlbach (ISM) sample (Figure 2A). Consequently, these results could either indicate the presence of different metabolic activities in these communities or that the Stickland reaction is not the main mechanism leading to the reduction of glycine in the medium.
Another explanation for the microbial removal of glycine from the medium could be the result of an energy-producing reaction where two molecules of glycine could be used to form serine and CO2. This has previously been reported for Pediococcus glycinophilus (Sagers and Gunsalus, 1961). Furthermore, glycine can be used as part of the peptidoglycan in the cell wall (Veuger et al., 2006).
With the current experimental set-up, a detailed analysis on the metabolic mechanisms underlying the preferred removal of glycine is not possible. However, the reduction of glycine and therefore the lack of detectability among the presence of other amino acids can be further explored as a potential biosignature.
In order to evaluate whether the absence of glycine is a valuable biosignature to find life on Mars, its abiotic stability on Mars needs to be considered. Glycine is one of the most abundant amino acids detected in meteorites and comets (Botta and Bada, 2002; Elsila et al., 2009). Various laboratory studies have investigated not only the abiotic degradation of glycine (Schuerger et al., 2011; Noblet et al., 2012; Gerakines and Hudson, 2015), but also its degradation under simulated Martian conditions (Ten Kate et al., 2005, 2006; Stalport et al., 2008).
Mars simulation studies determining the effect of UV irradiation on glycine revealed a degradation which results in the release of methane into the atmosphere (Schuerger et al., 2011). Owing to its simple chemical structure, glycine has the fastest degradation rate of amino acids. Extrapolated from ISS experiments, Noblet et al. (2012) estimated a half-life of approximately 51 h on the Martian surface. Another set of exposure experiments on the ISS (682 days for a total of 2,843 h solar constant radiation, equivalent to 1,043.4 MJm−2 of UV radiation in the range between 100 and 400 nm) showed that glycine was moderately stable in these conditions and even more so when mixed with meteoritic powder (Bertrand et al., 2015). Compared to UV radiation, galactic cosmic rays and solar energetic particles (mainly protons) can penetrate deeper into soil and ice (Mancinelli and Klovstad, 2000). A decrease by a factor 5–10 in a depth of a few meters is expected from the surface dose rate of 0.08 Gy/yr (Dartnell et al., 2007; Hassler et al., 2014).
Gerakines and Hudson (2015) performed experiments to study the half-lives of glycine in either CO2-ice or H2O-ice when irradiated with protons. The destruction rate constants indicated that glycine is less stable in CO2-ice (Mars) compared to H2O-ice (Mars and Europa). When extrapolating these data to conditions in the Martian subsurface, the half-life of glycine is modelled to be less than 100–200 million years even at depths of a few meters (Gerakines and Hudson, 2015). Other studies estimated that amino acids when shielded from radiation could potentially survive billions of years in cold and dry niches on Mars (Ehrenfreund and Charnley, 2000; Ehrenfreund et al., 2001b; Kminek and Bada, 2006; Aerts et al., 2016). Similar conclusions apply to Europa, Pluto, other icy satellites and to comets (Gerakines and Hudson, 2015).
Furthermore, a temperature effect has been observed in a previous study (Gerakines et al., 2012). With increasing temperatures, amino acids are less stable (Gerakines et al., 2012; Gerakines and Hudson, 2015). In addition, the mineralogy of the Martian regolith has an influence on the preservation of amino acids. Clay minerals or sulfate rich environments have been reported to show higher preservation rates compared to minerals containing ferrous iron (dos Santos et al., 2016). This effect was also noted in space exposure experiments where amino acids intermixed with meteorite powder had a higher stability than without (Bertrand et al., 2015).
These data imply that, in order to determine a biotic origin for the absence of glycine, several factors have to be considered. 1) Samples need to be shielded from radiation in general and UV radiation in particular. 2) Additional features such as the matrix in which glycine is trapped will have an effect on the stability. 3) Other environmental factors such as temperature can either decrease or increase the abiotic rate of glycine destruction in a certain niche. 4) Low molecular weight amino acids such as glycine, whose abiotic synthesis is kinetically favorable, dominate mixtures of amino acids synthesized by abiotic processes (Dorn et al., 2011). Due to the thermodynamics and kinetics of amino acid synthesis this observation is remarkably consistent for any synthesis environment. And lastly, the prevalence of glycine in an environment on Mars will be the result of a combination of these factors. In conclusion, the biotic degradation of glycine has potential as a biosignature of metabolic activity, but to avoid a false positive, it is necessary to understand the environmental conditions and context of the samples and the abiotic pathways and kinetics of glycine degradation.
Complexity of Other Amino Acids in Enrichments
Contrary to the glycine observations, there was no similar trend for the other amino acids in both samples. These complex and different changes in both enrichments could be the result of: 1) different chemical properties (Table 1), 2) the interaction of the various biochemical roles of amino acids, and 3) the composition of the microbial community. Amino acids are not only the building blocks of proteins, but have other functions including the use as energy metabolites, essential nutrients, or chemical messengers in communication between cells. Therefore, changes in the detectable amount of amino acids may represent the integrated effect of a diversity of metabolic pathways occurring in the respective microbial communities.
Microbial amino acid metabolism is a complex system involving transporters for uptake, biosynthesis as well as degradation and extraction of amino acids in a single microorganism. Transport mechanisms and metabolic pathways for the individual amino acids vary considerably in complexity (Krämer, 1994). There are two mechanisms that could lead to different fingerprints. On the one hand, as is the case in our experimental set-up, when amino acids are available in the environment, they are transported into the cells using different uptake systems. Differential consumption and utilization rates of the available substrate lead to a decrease of a certain amino acid. On the other hand, amino acids can be excreted from the cells. The formation of amino acids and intermediates in the course of amino acid metabolism which are released into the environment results in an increase of a certain amino acid. In addition, the complexity increases when investigating microbial communities as amino acid production and utilization are characterized by the sequential action of different metabolic pathways in organisms belonging to a consortium. There is the potential that an amino acid released into the medium from one member of the community can be utilized by another member and is therefore not detectable in the medium.
The results from the ISM enrichment revealed a higher amount of L-ala, L-val, L-leu and L-ile after incubation than initially added to the medium. This could be the result of amino acid synthesis from precursor molecules which then have been released into the medium either via active transport, diffusion or is a result of cell death and subsequent lysis (Gutiérrez-Preciado et al., 2010). Furthermore, the external increase of alanine might be indicative of a passive efflux which also has been observed for proline, aromatic and branched chain amino acids (Driessen, 1989; Krämer, 1994).
These results show how, in addition to the potential biosignature of the cells themselves, microbial metabolisms might increase the surrounding environmental concentrations of amino acids above those expected abiotically, suggesting that anomalously high concentrations of amino acids could be a biosignature. If these amino acids leached into preserved sediments where there was no preservation of cells themselves, they might act as an indirect signature of the proximal presence of life. Although we observed high concentrations of alanine, in principle an increase in any amino acid anomalously above the expected abiotic background could be an agnostic signature of biological processes.
We observed a decrease in the abundance of amino acids in the surrounding environment in the SM enrichment. One explanation for higher rates of substrate metabolism compared to biosynthesis leading to a reduction of amino acids, might be that the microbial community was performing maintenance metabolism rather than active growth. As with glycine, in order to be useful as a biosignature, these observed decreases would have to be considered alongside abiotic degradation rates. As for glycine, the UV photodestruction rate for L-alanine and β-alanine is dependent on whether the amino acids are on the surface (free) or embedded in UV non-penetrable solid surfaces (subsurface), or embedded in UV penetrable surfaces such as ice. On Mars, the half-life of ala with a radiation dose rate of 2.9 × 10–8 eV per year on the surface is 9.6 × 107 years compared to 1.7 × 108 years at 1 m depth with a radiation dose rate of 1.7 × 10–8 eV. Similar values with variations of about two orders of magnitude have been proposed for Pluto, comets in the outer Solar System, a cold diffuse and a dense interstellar medium (Gerakines and Hudson, 2015). As seen for glycine, the half-lives are several orders of magnitude lower for Europa at the near surface (1,200 years at 1 cm).
In summary, these data suggest that the individual fingerprints of the amino acids alanine, aspartic acid, valine, leucine, isoleucine and phenylalanine can vary, i.e., increase or decrease, depending on the composition of the microbial community. Cultivation and subsequent isolation has shown that the microbial communities from these two samples vary significantly (Cockell et al., 2017; Bashir et al., 2021). Although these complexities do not rule out such amino acid degradation patterns as biosignatures, they suggest that further investigation would be needed on any given sample, including its geological and temporal context, to determine if the differential changes in the amino acids in different communities can be disentangled from the expected abiotic degradation processes and attributed to a potential metabolic influence. Nevertheless, the observed synthesis and extracellular excretion of amino acids, leading to a large local increase in the concentration of some amino acids, as observed in the ISM sample, might be another promising signature of life.
Conclusion
The results of this study demonstrate a new type of amino acid imprint in an environment as a biomarker, which could be used alongside other methods to identify past and present life in extraterrestrial environments. The results indicate that the biologically mediated and almost complete depletion of glycine could be one amino acid signature that could be sought to corroborate the presence of life. Other amino acids showed diverse changes. Depletions could only be a biosignature if, like glycine, they can be disentangled from abiotic changes, but their presence would at least be consistent with life. Perhaps more interestingly, large increases in concentrations of amino acids resulting from excretion, might also be an agnostic indication of the presence of microbiota metabolizing amino acids. Finally, we note that the results address Objective 1A outlined in the Europa Lander SDT Report “… to seek evidence of biological activity by detecting and characterizing the organic composition of the sampled material.” (Hand et al., 2017). The results we present here show how the effects of life on the surrounding amino acid profile may be another organic signature of its presence and metabolic activities.
Data Availability Statement
The original contributions presented in the study are included in the article, further inquiries can be directed to the corresponding author.
Author Contributions
PS contributed to the experimental protocol, performed the experiments, data analysis, and wrote the manuscript. AR and DM performed the LC-MS measurements; PH, RL assisted in performing experiments and contributed by proof reading the manuscript. CS conceptualized the project as a whole and helped write the manuscript. All authors engaged in discussions, proof-read and approved the final manuscript.
Funding
AR acknowledges the support from the European Union’s Horizon 2020 research and innovation programme under the Marie Skłodowska-Curie grant agreement No. 750353. MASE is supported by European Community’s Seventh Framework Programme (FP7/2007-2013) under Grant Agreement n° 607297. Charles Cockell acknowledges the Science and Technology Facilities Council (grant ST/V000586/1).
Conflict of Interest
The authors declare that the research was conducted in the absence of any commercial or financial relationships that could be construed as a potential conflict of interest.
Publisher’s Note
All claims expressed in this article are solely those of the authors and do not necessarily represent those of their affiliated organizations, or those of the publisher, the editors and the reviewers. Any product that may be evaluated in this article, or claim that may be made by its manufacturer, is not guaranteed or endorsed by the publisher.
References
Aerts, J., Röling, W., Elsaesser, A., and Ehrenfreund, P. (2014). Biota and Biomolecules in Extreme Environments on Earth: Implications for Life Detection on Mars. Life 4, 535–565. doi:10.3390/life4040535
Aerts, J. W., Elsaesser, A., Röling, W. F. M., and Ehrenfreund, P. (2016). A Contamination Assessment of the CI Carbonaceous Meteorite Orgueil Using a DNA-Directed Approach. Meteorit. Planet. Sci. 51, 920–931. doi:10.1111/maps.12629
Aerts, J. W., Riedo, A., Melton, D. J., Martini, S., Flahaut, J., Meierhenrich, U. J., et al. (2020). Biosignature Analysis of Mars Soil Analogs from the Atacama Desert: Challenges and Implications for Future Missions to Mars. Astrobiology 20, 766–784. doi:10.1089/ast.2019.2063
Andreesen, J. R. (1994). “Acetate via glycine: A Different Form of Acetogenesis,” in Acetogenesis. Editor H. L. Drake (New York, NY: Chapman & Hall). doi:10.1007/978-1-4615-1777-1_23
Avnir, D. (2021). Critical Review of Chirality Indicators of Extraterrestrial Life. New Astron. Rev. 92, 101596. doi:10.1016/j.newar.2020.101596
Bada, J. L., and McDonald, G. D. (1996). Peer Reviewed: Detecting Amino Acids on Mars. Anal. Chem. 68, 668A–673A. doi:10.1021/ac9621231
Bashir, A. K., Wink, L., Duller, S., Schwendner, P., Cockell, C., Rettberg, P., et al. (2021). Taxonomic and Functional Analyses of Intact Microbial Communities Thriving in Extreme, Astrobiology-Relevant, Anoxic Sites. Microbiome 9, 50. doi:10.1186/s40168-020-00989-5
Bertrand, M., Chabin, A., Colas, C., Cadène, M., Chaput, D., Brack, A., et al. (2015). The AMINO experiment: Exposure of Amino Acids in the EXPOSE-R experiment on the International Space Station and in Laboratory. Int. J. Astrobiol. 14, 89–97. doi:10.1017/S1473550414000354
Botta, O., and Bada, J. L. (2002). Extraterrestrial Organic Compounds in Meteorites. Surv. Geophys. 23, 411–467. doi:10.1023/A:1020139302770
Busemann, H., Young, A. F., Alexander, C. M. O. D., Hoppe, P., Mukhopadhyay, S., and Nittler, L. R. (2006). Interstellar Chemistry Recorded in Organic Matter from Primitive Meteorites. Science 312, 727–730. doi:10.1126/science.1123878
Cleaves, H. J., Chalmers, J. H., Lazcano, A., Miller, S. L., and Bada, J. L. (2008). A Reassessment of Prebiotic Organic Synthesis in Neutral Planetary Atmospheres. Orig Life Evol. Biosph. 38, 105–115. doi:10.1007/s11084-007-9120-3
Cobb, A. K., and Pudritz, R. E. (2014). Nature's Starships. I. Observed Abundances and Relative Frequencies of Amino Acids in Meteorites. ApJ 783, 140. doi:10.1088/0004-637x/783/2/140
Cockell, C. S., Schwendner, P., Perras, A., Rettberg, P., Beblo-Vranesevic, K., Bohmeier, M., et al. (2017). Anaerobic Microorganisms in Astrobiological Analogue Environments: from Field Site to Culture Collection. Int. J. Astrobiol. 17, 314–328. doi:10.1017/S1473550417000246
Creamer, J. S., Mora, M. F., and Willis, P. A. (2017). Enhanced Resolution of Chiral Amino Acids with Capillary Electrophoresis for Biosignature Detection in Extraterrestrial Samples. Anal. Chem. 89, 1329–1337. doi:10.1021/acs.analchem.6b04338
Cronin, J. R., and Pizzarello, S. (1983). Amino Acids in Meteorites. Adv. Space Res. 3, 5–18. doi:10.1016/0273-1177(83)90036-4
Dartnell, L. R., Desorgher, L., Ward, J. M., and Coates, A. J. (2007). Modelling the Surface and Subsurface Martian Radiation Environment: Implications for Astrobiology. Geophys. Res. Lett. 34, L0227. doi:10.1029/2006GL027494
Davila, A. F., and McKay, C. P. (2014). Chance and Necessity in Biochemistry: Implications for the Search for Extraterrestrial Biomarkers in Earth-like Environments. Astrobiology 14, 534–540. doi:10.1089/ast.2014.1150
Deamer, D., Dworkin, J. P., Sandford, S. A., Bernstein, M. P., and Allamandola, L. J. (2002). The First Cell Membranes. Astrobiology 2, 371–381. doi:10.1089/153110702762470482
Dorn, E. D., Nealson, K. H., and Adami, C. (2011). Monomer Abundance Distribution Patterns as a Universal Biosignature: Examples from Terrestrial and Digital Life. J. Mol. Evol. 72, 283–295. doi:10.1007/s00239-011-9429-4
dos Santos, R., Patel, M., Cuadros, J., and Martins, Z. (2016). Influence of Mineralogy on the Preservation of Amino Acids under Simulated Mars Conditions. Icarus 277, 342–353. doi:10.1016/j.icarus.2016.05.029
Driessen, A. J. (1989). Secondary Transport of Amino Acids by Membrane Vesicles Derived from Lactic Acid Bacteria. Antonie Van Leeuwenhoek 56, 139–160. doi:10.1007/BF00399978
Ehrenfreund, P., and Charnley, S. B. (2000). Organic Molecules in the Interstellar Medium, Comets, and Meteorites: A Voyage from Dark Clouds to the Early Earth. Annu. Rev. Astron. Astrophys. 38, 427–483. doi:10.1146/annurev.astro.38.1.427
Ehrenfreund, P., Bernstein, M. P., Dworkin, J. P., Sandford, S. A., and Allamandola, L. J. (2001a). The Photostability of Amino Acids in Space. Astrophys. J. 550, L95–L99. doi:10.1086/319491
Ehrenfreund, P., Glavin, D. P., Botta, O., Cooper, G., and Bada, J. L. (2001b). Extraterrestrial Amino Acids in Orgueil and Ivuna: Tracing the Parent Body of CI Type Carbonaceous Chondrites. Proc. Natl. Acad. Sci. 98, 2138–2141. doi:10.1073/pnas.051502898
Elsila, J. E., Glavin, D. P., and Dworkin, J. P. (2009). Cometary glycine Detected in Samples Returned by Stardust. Meteorit. Planet. Sci. 44, 1323–1330. doi:10.1111/j.1945-5100.2009.tb01224.x
Elsila, J. E., Aponte, J. C., Blackmond, D. G., Burton, A. S., Dworkin, J. P., and Glavin, D. P. (2016). Meteoritic Amino Acids: Diversity in Compositions Reflects Parent Body Histories. ACS Cent. Sci. 2, 370–379. doi:10.1021/acscentsci.6b00074
Fichtner, M., Voigt, K., and Schuster, S. (2017). The Tip and Hidden Part of the Iceberg: Proteinogenic and Non-proteinogenic Aliphatic Amino Acids. Biochim. Biophys. Acta (Bba) - Gen. Subj. 1861, 3258–3269. doi:10.1016/j.bbagen.2016.08.008
Georgiou, C. D. (2018). Functional Properties of Amino Acid Side Chains as Biomarkers of Extraterrestrial Life. Astrobiology 18, 1479–1496. doi:10.1089/ast.2018.1868
Gerakines, P. A., and Hudson, R. L. (2015). The Radiation Stability of glycine in Solid CO2 - In Situ Laboratory Measurements with Applications to Mars. Icarus 252, 466–472. doi:10.1016/j.icarus.2015.02.008
Gerakines, P. A., Hudson, R. L., Moore, M. H., and Bell, J.-L. (2012). In Situ measurements of the Radiation Stability of Amino Acids at 15-140K. Icarus 220, 647–659. doi:10.1016/j.icarus.2012.06.001
Glavin, D. P., Aubrey, A. D., Callahan, M. P., Dworkin, J. P., Elsila, J. E., Parker, E. T., et al. (2010). Extraterrestrial Amino Acids in the Almahata Sitta Meteorite. Meteorit. Planet. Sci. 45, 1695–1709. doi:10.1111/j.1945-5100.2010.01094.x
Glavin, D. P., Elsila, J. E., Burton, A. S., Callahan, M. P., Dworkin, J. P., Hilts, R. W., et al. (2012). Unusual Nonterrestrial L-Proteinogenic Amino Acid Excesses in the Tagish Lake Meteorite. Meteorit. Planet. Sci. 47, 1347–1364. doi:10.1111/j.1945-5100.2012.01400.x
Grotzinger, J., and Rothman, D. H. (1996). An Abiotic Model for Stromatolite Morphogenesis. Nature 382, 432–425. doi:10.1038/383423a0
Gutiérrez-Preciado, A., Romero, H., and Peimbert, M. (2010). An Evolutionary Perspective on Amino Acids. Nat. Sci. Educ. 3, 29.
Hand, K. P., Murray, A. E., Garbing, J. B., Brinkeckerhoff, W. B., Christner, B. C., Edgett, K. S., et al. (2017). Report of the Europa Lander Science Definition Team. Posted February, 2017. Available at: https://europa.nasa.gov/resources/58/europa-lander-study-2016-report/.
Hassler, D. M., Zeitlin, C., Wimmer-Schweingruber, R. F., Ehresmann, B., Rafkin, S., Eigenbrode, J. L., et al. (2014). Mars' Surface Radiation Environment Measured with the Mars Science Laboratory's Curiosity Rover. Science 343, 1244797. doi:10.1126/science.1244797
Hays, L. E., Graham, H. V., Des Marais, D. J., Hausrath, E. M., Horgan, B., McCollom, T. M., et al. (2017). Biosignature Preservation and Detection in Mars Analog Environments. Astrobiology 17, 363–400. doi:10.1089/ast.2016.1627
House, C. H., William Schopf, J., McKeegan, K. D., Coath, C. D., Mark Harrison, T., and Stetter, K. O. (2000). Carbon Isotopic Composition of Individual Precambrian Microfossils. Geology 28, 707–710. doi:10.1130/0091-7613(2000)028<0707:cicoip>2.3.co;2
Kaiser, K., and Benner, R. (2008). Major Bacterial Contribution to the Ocean Reservoir of Detrital Organic Carbon and Nitrogen. Limnol. Oceanogr. 53, 99–112. doi:10.4319/lo.2008.53.1.0099
Kminek, G., and Bada, J. (2006). The Effect of Ionizing Radiation on the Preservation of Amino Acids on Mars. Earth Planet. Sci. Lett. 245, 1–5. doi:10.1016/j.epsl.2006.03.008
Kozlowski, L. P. (2016). Proteome-pI: Proteome Isoelectric point Database. Nucleic Acids Res. 45, D1112–D1116. doi:10.1093/nar/gkw978
Krämer, R. (1994). Systems and Mechanisms of Amino Acid Uptake and Excretion in Prokaryotes. Arch. Microbiol. 162, 1–13.
Kvenvolden, K. A. (1973). Criteria for Distinguishing Biogenic and Abiogenic Amino Acids: Preliminary Considerations. Space Life Sci. 4, 60–68. doi:10.1007/bf02626342
Lam, H., Oh, D.-C., Cava, F., Takacs, C. N., Clardy, J., de Pedro, M. A., et al. (2009). D-amino Acids Govern Stationary Phase Cell Wall Remodeling in Bacteria. Science 325, 1552–1555. doi:10.1126/science.1178123
Mancinelli, R. L., and Klovstad, M. (2000). Martian Soil and UV Radiation: Microbial Viability Assessment on Spacecraft Surfaces. Planet. Space Sci. 48, 1093–1097. doi:10.1016/S0032-0633(00)00083-0
Martins, Z., Botta, O., and Ehrenfreund, P. (2007a). Amino Acids in Antarctic CM1 Meteorites and Their Relationship to Other Carbonaceous Chondrites. Meteorit. Planet. Sci. 42, 81–92. doi:10.1111/j.1945-5100.2007.tb00219.x
Martins, Z., Alexander, C. M. O. D., Orzechowska, G. E., Fogel, M. L., Ehrenfreund, P., and Ehrenfreund, P. (2007b). Indigenous Amino Acids in Primitive CR Meteorites. Meteorit. Planet. Sci. 42, 2125–2136. doi:10.1111/j.1945-5100.2007.tb01013.x
Martins, Z., Hofmann, B. A., Gnos, E., Greenwood, R. C., Verchovsky, A., Franchi, I. A., et al. (2007c). Amino Acid Composition, Petrology, geochemistry,14C Terrestrial Age and Oxygen Isotopes of the Shişr 033 CR Chondrite. Meteorit. Planet. Sci. 42, 1581–1595. doi:10.1111/j.1945-5100.2007.tb00592.x
Miller, S. L. (1993). The Prebiotic Synthesis of Organic Compounds on the Early Earth. Org. Geochem. 11, 625–637. doi:10.1007/978-1-4615-2890-6_30
Moissl, C., Rudolph, C., and Huber, R. (2002). Natural Communities of Novel Archaea and Bacteria with a String-of-Pearls-like Morphology: Molecular Analysis of the Bacterial Partners. Appl. Environ. Microbiol. 68, 933–937. doi:10.1128/aem.68.2.933-937.2002
Nimura, N., and Kinoshita, T. (1986). o-Phthalaldehyde-N-acetyl-L-cysteine as a Chiral Derivatization Reagent for Liquid Chromatographic Optical Resolution of Amino Acid Ernantiomers and its Application to Conventional Amino Acid Analysis. J. Chromatogr. A 352, 169–177. doi:10.1016/S0021-9673(01)83377-X
Nixon, S. L., Cockell, C. S., and Tranter, M. (2012). Limitations to a Microbial Iron Cycle on Mars. Planet. Space Sci. 72, 116–128. doi:10.1016/j.pss.2012.04.003
Noblet, A., Stalport, F., Guan, Y. Y., Poch, O., Coll, P., Szopa, C., et al. (2012). The PROCESS Experiment: Amino and Carboxylic Acids under Mars-Like Surface UV Radiation Conditions in Low-Earth Orbit. Astrobiology 12, 436–444. doi:10.1089/ast.2011.0756
Parnell, J., Cullen, D., Sims, M. R., Bowden, S., Cockell, C. S., Court, R., et al. (2007). Searching for Life on Mars: Selection of Molecular Targets for ESA's Aurora ExoMars Mission. Astrobiology 7, 578–604. doi:10.1089/ast.2006.0110
Pizzarello, S., and Holmes, W. (2009). Nitrogen-containing Compounds in Two CR2 Meteorites: 15N Composition, Molecular Distribution and Precursor Molecules. Geochim. Cosmochim. Acta 73, 2150–2162. doi:10.1016/j.gca.2009.01.022
Pizzarello, S., and Shock, E. (2010). The Organic Composition of Carbonaceous Meteorites: the Evolutionary story Ahead of Biochemistry. Cold Spring Harbor Perspect. Biol. 2, a002105. doi:10.1101/cshperspect.a002105
Pizzarello, S. (2006). The Chemistry of Life's Origin: A Carbonaceous Meteorite Perspective. Acc. Chem. Res. 39, 231–237. doi:10.1021/ar050049f
Probst, A. J., Weinmaier, T., Raymann, K., Perras, A., Emerson, J. B., Rattei, T., et al. (2014). Biology of a Widespread Uncultivated Archaeon that Contributes to Carbon Fixation in the Subsurface. Nat. Commun. 5, 5497. doi:10.1038/ncomms6497
Rudolph, C., Moissl, C., Henneberger, R., and Huber, R. (2004). Ecology and Microbial Structures of Archaeal/bacterial Strings-Of-Pearls Communities and Archaeal Relatives Thriving in Cold Sulfidic Springs. FEMS Microbiol. Ecol. 50, 1–11. doi:10.1016/j.femsec.2004.05.006
Sagers, R. D., and Gunsalus, I. C. (1961). Intermediary Metabolism of Diplococcus Glycinophilus I. J. Bacteriol. 81, 541–549. doi:10.1128/jb.81.4.541-549.1961
Schuerger, A. C., Clausen, C., and Britt, D. (2011). Methane Evolution from UV-Irradiated Spacecraft Materials under Simulated Martian Conditions: Implications for the Mars Science Laboratory (MSL) mission. Icarus 213, 393–403. doi:10.1016/j.icarus.2011.02.017
Seager, S., Schrenk, M., and Bains, W. (2012). An Astrophysical View of Earth-Based Metabolic Biosignature Gases. Astrobiology 12, 61–82. doi:10.1089/ast.2010.0489
Sephton, M. A. (2002). Organic Compounds in Carbonaceous Meteorites. Nat. Prod. Rep. 19, 292–311. doi:10.1039/b103775g
Shen, Y., Buick, R., and Canfield, D. E. (2001). Isotopic Evidence for Microbial Sulphate Reduction in the Early Archaean Era. Nature 410, 77–81. doi:10.1038/35065071
Shimoyama, A., Harada, K., and Yanai, K. (1985). Amino Acids from the Yamato-791198 Carbonaceous Chondrite from Antarctica. Chem. Lett. 14, 1183–1186. doi:10.1246/cl.1985.1183
Souza-Corrêa, J. A., da Costa, C. A. P., and Da Silveira, E. F. (2019). Compaction and Destruction Cross-Sections for α-Glycine from Radiolysis Process via 1.0 keV Electron Beam as a Function of Temperature. Astrobiology 19, 1123–1138. doi:10.1089/ast.2018.1986
Stalport, F., Coll, P., Szopa, C., and Raulin, F. (2008). Search for Organic Molecules at the Mars Surface: The "Martian Organic Material Irradiation and Evolution" (MOMIE) Project. Adv. Space Res. 42, 2014–2018. doi:10.1016/j.asr.2007.07.004
Summons, R. E., Albrecht, P., McDonald, G., and Moldowan, J. M. (2008). “Molecular Biosignatures,” in Strategies of Life Detection. Space Sciences Series of ISSI. Editors O. Botta, J. L. Bada, J. Gomez-Elvira, E. Javaux, F. Selsis, and R. Summons (Boston, MA: Springer), 133–159. doi:10.1007/978-0-387-77516-6_11
Ten Kate, I. L., Garry, J. R. C., Peeters, Z., Quinn, R., Foing, B., and Ehrenfreund, P. (2005). Amino Acid Photostability on the Martian Surface. Meteorit. Planet. Sci. 40, 1185–1193. doi:10.1111/j.1945-5100.2005.tb00183.x
Ten Kate, I. L., Garry, J. R. C., Peeters, Z., Foing, B., and Ehrenfreund, P. (2006). The Effects of Martian Near Surface Conditions on the Photochemistry of Amino Acids. Planet. Space Sci. 54, 296–302. doi:10.1016/j.pss.2005.12.002
Veuger, B., van Oevelen, D., Boschker, H. T. S., and Middelburg, J. J. (2006). Fate of Peptidoglycan in an Intertidal Sediment: An In Situ 13 C-Labeling Study. Limnol. Oceanogr. 51, 1572–1580. doi:10.4319/lo.2006.51.4.1572
Westall, F., Cavalazzi, B., Lemelle, L., Marrocchi, Y., Rouzaud, J.-N., Simionovici, A., et al. (2011). Implications of In Situ Calcification for Photosynthesis in a ∼3.3Ga-old Microbial Biofilm from the Barberton Greenstone belt, South Africa. Earth Planet. Sci. Lett. 310, 468–479. doi:10.1016/j.epsl.2011.08.029
Keywords: amino acids, biomarker, search for life, glycine, habitability, microbial degradation
Citation: Schwendner P, Riedo A, Melton DJ, Horvath P, Lindner R, Ehrenfreund P, Beblo-Vranesevic K, Rettberg P, Rabbow E, Westall F, Bashir A, Moissl-Eichinger C, Garcia-Descalzo L, Gomez F, Amils R, Marteinsson VÞ, Walter N and Cockell CS (2022) Microbial Metabolism of Amino Acids—Biologically Induced Removal of Glycine and the Resulting Fingerprint as a Potential Biosignature. Front. Astron. Space Sci. 9:781542. doi: 10.3389/fspas.2022.781542
Received: 22 September 2021; Accepted: 25 February 2022;
Published: 04 April 2022.
Edited by:
Chandra Sivaram, Indian Institute of Astrophysics, IndiaReviewed by:
Mihaela Glamoclija, Rutgers University, United StatesJan Hendrik Bredehöft, University of Bremen, Germany
Copyright © 2022 Schwendner, Riedo, Melton, Horvath, Lindner, Ehrenfreund, Beblo-Vranesevic, Rettberg, Rabbow, Westall, Bashir, Moissl-Eichinger, Garcia-Descalzo, Gomez, Amils, Marteinsson, Walter and Cockell. This is an open-access article distributed under the terms of the Creative Commons Attribution License (CC BY). The use, distribution or reproduction in other forums is permitted, provided the original author(s) and the copyright owner(s) are credited and that the original publication in this journal is cited, in accordance with accepted academic practice. No use, distribution or reproduction is permitted which does not comply with these terms.
*Correspondence: Petra Schwendner, cGV0cmEuc2Nod2VuZG5lckBlZC5hYy51aw==