- 1Space Science Center, University of New Hampshire, Durham, NH, United States
- 2Cooperative Programs for the Advancement of Earth System Science (CPAESS), University Corporation for Atmospheric Research, Boulder, CO, United States
- 3Heliophysics Science Division, NASA Goddard Space Flight Center, Greenbelt, MD, United States
- 4Institute of Physics, University of Graz, Graz, Austria
- 5Department of Physics, The Catholic University of America, Washington, DC, United States
- 6Observatoire de Paris, LESIA, UMR8109, CNRS, F-92195 Meudon Principal, Cedex, France
- 7Centre for mathematical Plasma Astrophysics, Department of Mathematics, KU Leuven, Leuven, Belgium
- 8School of Physics and Astronomy, University of Glasgow, Glasgow, United Kingdom
- 9Institute of Physics, University of Maria Curie-Skłodowska, Lublin, Poland
- 10Space Sciences Laboratory, University of California, Berkeley, Berkeley, CA, United States
- 11Naval Research Laboratory, Space Science Division, Washington, DC, United States
- 12Predictive Science Inc., San Diego, CA, United States
- 13Department of Physics and Astronomy, George Mason University, Fairfax, VA, United States
- 14Johns Hopkins University Applied Physics Laboratory, Laurel, MD, United States
- 15Department of Climate and Space Sciences and Engineering, University of Michigan, Ann Arbor, MI, United States
- 16Lockheed Martin Solar and Astrophysics Laboratory, Palo Alto, CA, United States
- 17Laboratoire d’Astrophysique de Bordeaux, University of Bordeaux, CNRS, Pessac, France
This perspective paper brings to light the need for comprehensive studies on the evolution of interplanetary coronal mass ejection (ICME) complexity during propagation. To date, few studies of ICME complexity exist. Here, we define ICME complexity and associated changes in complexity, describe recent works and their limitations, and outline key science questions that need to be tackled. Fundamental research on ICME complexity changes from the solar corona to 1 AU and beyond is critical to our physical understanding of the evolution and interaction of transients in the inner heliosphere. Furthermore, a comprehensive understanding of such changes is required to understand the space weather impact of ICMEs at different heliospheric locations and to improve on predictive space weather models.
1 Introduction and early studies
This paper [which is based on a white paper submitted to the Decadal Survey for Solar and Space Physics 2024–2033 (Winslow et al., 2022)] addresses the need to investigate, from a fundamental physics perspective, the interplanetary evolution of coronal mass ejections (CMEs), and specifically the evolution of their complexity in the inner heliosphere. The absolute complexity of an interplanetary CME (ICME) at any one heliocentric distance is difficult to define in isolation because it needs to be defined relative to a reference state of assumed low complexity (e.g., Jones et al., 2020). Generally speaking, however, complexity can be understood as the degree of similarity or deviation of a given ICME structure from a “standard” configuration characterized by a magnetic ejecta (ME) or magnetic cloud (MC) with a flux-rope magnetic structure connected back to the Sun by two “legs” (see, e.g., Figure 2 in Zurbuchen and Richardson, 2006). Such a picture, developed through decades of observations and the consideration of a large number of events (e.g., Burlaga et al., 1981; Zurbuchen and Richardson, 2006; Kilpua et al., 2017; Luhmann et al., 2020), presupposes the existence of a paradigm accepted by the community as a descriptor of a typical, or low complexity, state.
On the other hand, it is much simpler to determine the relative change in a particular ICME’s complexity between different heliocentric distances. By ICME complexity changes, we mean any significant changes in the different parts of the ICME structure (sheath/ME) both from a magnetic configuration and plasma characteristics/composition standpoint, that take the ICME from a simple (complex) starting point at one heliocentric distance to be more complex (simple) at a farther heliocentric distance (e.g., Figure 1).
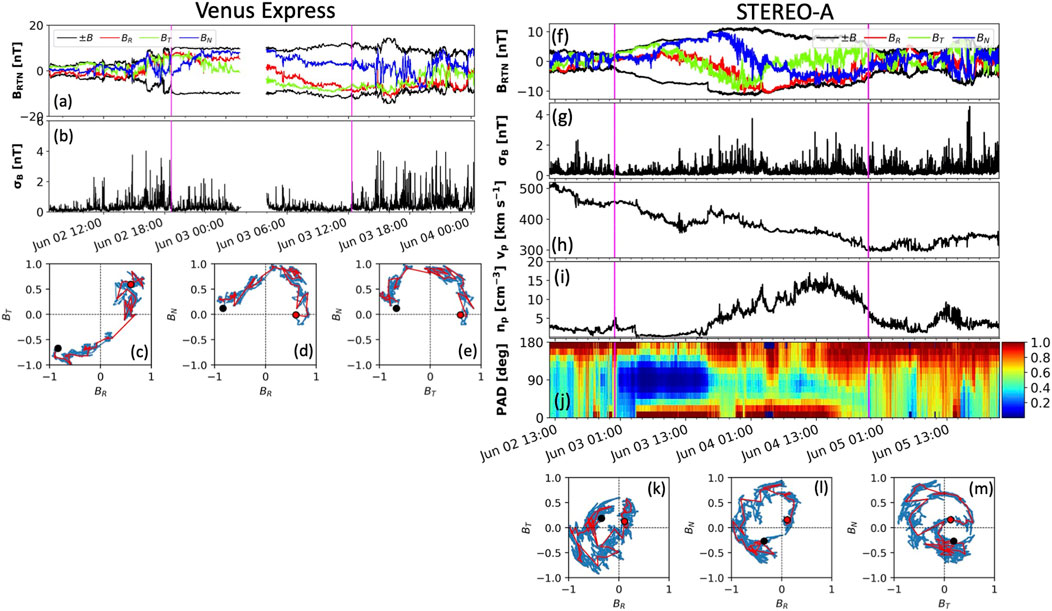
FIGURE 1. Example of an ICME observed at 0.73 au and 0.96 au in 2009 by Venus Express (A–E) and STEREO-A (F–M) in radial alignment (with a longitudinal separation of 9°). The flux-rope magnetic structure observed at the inner spacecraft (A) clearly appears as a more complex, non-MC structure by the time the ICME reaches the outer spacecraft (F) due to interaction with an interplanetary shock. From Scolini et al. (2022b), reproduced by permission of the AAS.
Based on recent papers described in detail below (Richardson and Cane, 2010; Winslow et al., 2016; Winslow et al., 2021a; Winslow et al., 2021b; Scolini et al., 2021; Scolini et al., 2022a; Scolini et al., 2022b), the hypothesis exists that the complexity of ICMEs increases during propagation in the inner heliosphere mainly due to interaction with large-scale solar wind structures. The assumption is that this would occur regardless of the initial CME complexity state in the corona, i.e., some CMEs will be more complex than others at the time of initiation, however, their individual complexity state is generally expected to be lowest after launch from the Sun and increase during propagation primarily due to interactions with other transients and large-scale solar wind structures. Thus far, only a few studies, have addressed this hypothesis directly, largely due to the lack of suitable and comprehensive in situ data of the same ICMEs at multiple heliocentric distances.
However, currently, it is not a certainty that ICME complexity increases with increasing distance. For instance, it may be the case that a CME is formed with a relatively complex internal structure that evolves toward a simpler configuration as it expands outwards. For example, Janvier et al. (2019) found, from superposed epoch analyses of ICMEs between 0.3 and 1 AU, that the overall magnetic field profile of ICMEs became more symmetric as they propagated farther from the Sun, indicating a relaxation mechanism possibly taking place. Furthermore, Florido-Llinas et al. (2020) showed, via modeling with the circular–cylindrical analytic flux rope model (Nieves-Chinchilla et al., 2016), that there are conditions under which flux ropes can expand self-similarly and even become more kink-stable during propagation. It is also possible that certain regions (or substructures) of ICMEs undergo complexity increases while other areas might decrease in complexity during propagation, possibly arising from the fact that the ICME itself might not behave as a coherent unit (Owens et al., 2017). Further work is needed to properly classify and characterize such changes in ICMEs.
Many previous studies have shown that ICME properties evolve as they propagate through the solar wind due to expansion and interaction with the solar wind and transients within it (Manchester et al., 2017). Through observational and modeling work, studies have shown that during propagation, the ICME flux rope may kink and deform (Odstrčil and Pizzo, 1999a; Manchester et al., 2004; Savani et al., 2010; Török et al., 2018), reconnection/erosion of internal ICME magnetic flux may occur (Lavraud et al., 2014; Ruffenach et al., 2015), and the ICME may also get deflected (Wang et al., 2014; Kay and Opher, 2015) and rotated (Isavnin et al., 2014). Most importantly, all of the aforementioned effects are amplified by interactions with high-speed streams (HSSs), stream interaction regions (SIRs), the heliospheric current/plasma sheet (HCS/HPS) (Odstrčil and Pizzo, 1999b; Odstrčil and Pizzo, 1999c; Lavraud and Rouillard, 2014; Rodriguez et al., 2016; Zhou and Feng, 2017; Liu et al., 2019; Davies et al., 2020; Winslow et al., 2021b; Scolini et al., 2021), as well as other ICMEs (e.g., Lugaz et al., 2017; Verbeke et al., 2022), even ones observed out to Saturn (Palmerio et al., 2021), suggesting interactions with other interplanetary structures are a critical factor in the evolution of ICME structures during propagation. Overall, these studies have detailed the types of changes that ICMEs undergo during propagation, however, they have not considered them from the broader view of their overall complexity.
The idea that ICME structures become more and more complex with heliocentric distance has been first inferred from statistical investigations on the fraction of ICMEs that contain MC structures (Burlaga et al., 1981). At 1 AU, the fraction of non-MC ICMEs is strongly dependent on the solar cycle (Richardson and Cane, 2010), indicating that more ICMEs might have interacted with transients in the solar wind during solar maxima than during minima. Meanwhile, studies of individual ICMEs observed by multiple radially aligned spacecraft also increased our understanding of their complexity evolution. They showcased a wide variety of evolutionary behaviors, ranging from essentially self-similar (Nakwacki et al., 2011; Möstl et al., 2012; Good et al., 2015; Good et al., 2018) to strongly non-ideal (Nieves-Chinchilla et al., 2012; Winslow et al., 2016; Lugaz et al., 2020), posing questions on the frequency and causes of such a large variation in evolutionary trends.
Recent studies on ICME complexity changes (e.g., Winslow et al., 2016; Winslow et al., 2021b; Scolini et al., 2022b; Davies et al., 2022) have since uncovered many characteristics through which these changes can manifest: significant changes in flux rope structure and orientation; indications (in solar wind, magnetic field, suprathermal electron, and iron charge state data) that the ICME underwent reconnection, as well as changes in the detection of the MC substructure with heliocentric distance (e.g., if a clear MC configuration is no longer detected at spacecraft farther from the Sun although it was detected at smaller heliocentric distances). Additionally, the mass/density increase with distance in ICME-driven sheaths may affect the ME as it expands, thereby also affecting the complexity evolution (e.g., Good et al., 2020; Temmer et al., 2021). Similarly, atypical evolution of sheaths (such as significant growth beyond expected values from expansion only, as well as large increases in dynamic pressure) can also contribute to complexity changes (e.g., Winslow et al., 2021b).
It is important to note, however, that most studies of ICME complexity to date have not considered complexity changes holistically, i.e., by exploring changes in all aspects of the ICME as opposed to simply investigating one or two parameters (e.g., magnetic field configuration). Comprehensive investigations of ICME complexity are needed to test the hypothesis that complexity increases with distance, and to understand these complexity changes from a more fundamental physics standpoint.
2 Causes and effects of complexity changes
In-depth analyses of ICME case studies through multi-point spacecraft measurements in radial alignment have first illustrated the impacts that solar wind structures can have on ICMEs. Winslow et al. (2016) showcased an ICME that underwent significant deformation causing increased ICME complexity as it propagated from Mercury to 1 AU. This increased complexity was found to be due to interaction/reconnection with the HCS and HPS. More recently, Winslow et al. (2021b) presented a comparative analysis of two ICME case studies observed in radial alignment at Mercury and 1 au, of which one propagated essentially self-similarly, while the other exhibited major changes to its global structure (affecting both the flux rope and preceding sheath) due to interaction with an SIR. In a complementary study, Winslow et al. (2021a) investigated an ICME overtaken and accelerated by a HSS that was observed simultaneously by Parker Solar Probe and STEREO-A during a period of close radial alignment. In this case, the ICME interacted with the HSS for at least ∼2.5 days prior to arrival at STEREO-A (i.e., the interaction began well before arrival at either spacecraft), and therefore the flux rope configuration detected in the ICME was the same at both locations. However, the ICME as a whole exhibited significant complexity (e.g., the shorter duration of the flux rope compared to the duration of the entire ME) due to the compressing action of the overtaking HSS.
The expansion of such investigations to a statistical set of events has been long complicated by the limited amount of assets capable of performing high-quality observations of ICME structures at multiple heliocentric distances. When restricting the study to only the magnetic structures of ICMEs (i.e., focusing on MEs and neglecting ICME-driven shocks and sheaths; see, e.g., ME configurations illustrated in Nieves-Chinchilla et al., 2018; Nieves-Chinchilla et al., 2019), Scolini et al. (2022b) were able to generalize previous case study results and draw statistically-valid conclusions (albeit based on small-number statistics) on the frequency, causes, and effects of ICME magnetic complexity changes. They found, from 31 ICMEs observed in radial alignment between 0.3 and 1 au, that ICMEs tend to increase their magnetic complexity with heliocentric distance, and that these changes are in most cases induced by the interaction with multiple solar wind structures, i.e., HSSs, SIRs, the HCS, or other interplanetary shocks (Figure 2). On the contrary, ICMEs that preserved their magnetic configuration during propagation tended to either lack any interaction with other interplanetary structures, or to interact only with a single one. An example of an ICME increasing its magnetic complexity from an inner to an outer observing spacecraft is provided in Figure 1. It is important to mention, however, that the Scolini et al. (2022b) study is a “trailblazer” in the sense that it is a first-of-its-kind statistical study on ICME complexity, and it is not conclusive or comprehensive. It is based on small-number statistics, the observations used lack plasma data at the inner spacecraft, and it relies on magnetohydrodynamic (MHD) model simulations of the background solar wind to identify solar wind structures that the ICME interacted with (i.e., lacks data in the propagation space). More comprehensive statistical studies are needed in the future, which can only be achieved through multiple spacecraft simultaneously probing heliospheric conditions at different distances.
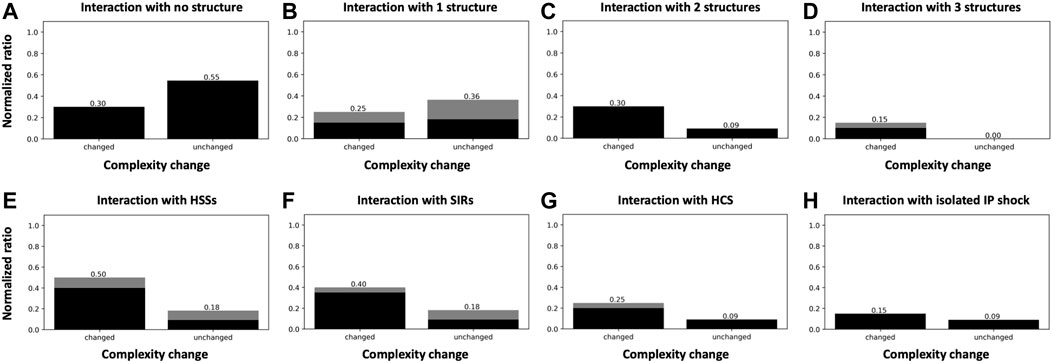
FIGURE 2. Summary of the frequency of ICME magnetic complexity changes as a function of the number of interactions that the ICME underwent during propagation (A–D), and of the type of interacting solar wind structure (E–H). Certain and probable interactions are indicated in black and gray, respectively. Adapted from Scolini et al. (2022b), reproduced by permission of the AAS.
Aside from alterations in the magnetic field configuration of ICMEs, Scolini et al. (2022b) also found that complexity changes are statistically correlated with reduced periods of bi-directional suprathermal electron strahls observed within ICMEs, indicative of major alterations to their magnetic topology and connectivity back to the Sun (e.g., Gosling et al., 1987; Kahler and Reames, 1991; Shodhan et al., 2000), and randomization of the average ICME internal properties such as the breaking of the speed–magnetic field relationship (e.g., Gonzalez et al., 1998; Owens and Cargill, 2002; Owens et al., 2005) that holds for unperturbed ICMEs (Scolini et al., 2022b). These results caution against the use of inner heliospheric observations to predict the magnetic field strength and orientation at a downstream target location separated by more than ∼0.2 au.
Numerical models have allowed us to investigate the effect of solar wind interactions on ICME structures to a level of detail exceeding current observational capabilities. Of particular relevance is the possibility to include simulated spacecraft at orbits and relative positions not available in reality. Taking advantage of such flexibility, Scolini et al. (2021) quantified the probability of detecting changes in ICME complexity through a swarm of simulated spacecraft placed in perfect radial alignment between 0.1 and 1.6 au within global heliospheric simulations, given the absence/presence of corotating solar wind structures. Results of this study suggest that HSSs and SIRs dominate contributions to ICME magnetic complexity increases throughout the inner heliosphere.
We underline that such numerical investigations have been performed using global heliospheric models in simplified numerical set-ups, which facilitate the interpretation of propagation effects on different ICME regions. Future studies should address more realistic numerical set-ups and investigations of real ICME events, including comparisons with observations, as well as the use of more sophisticated models (see recommendations in Section 4).
3 Observational gaps: Data products and spacecraft location
When considering observational gaps, we must first consider the kind of measurements available at different spacecraft various locations in the inner heliosphere. Measurements of key solar wind plasma properties, including bulk thermal properties, suprathermal populations, and composition data are of utmost importance to decipher the fundamental nature of ICME complexity changes. By observational gaps, we mean a lack of spacecraft measurements (all or just some types) along the specific radial, longitudinal, and latitudinal locations of interest. Currently, the largest obstacle to advancing our studies of ICME complexity evolution is the lack of a full suite of in situ plasma and magnetic field data at small enough spatial separations but also covering a large distance range (and therefore likely needing to involve a large number of spacecraft) from the Sun to 1 au to unambiguously detect complexity changes and determine their causes.
In general, gaps in the aforementioned data products available may: 1) lead to higher uncertainties in the identification of ICME boundaries (e.g., Cane and Richardson, 2003; Riley et al., 2004; Jian et al., 2006; Al-Haddad et al., 2013; Winslow et al., 2015; Good and Forsyth, 2016; Davies et al., 2021); 2) prevent a detailed investigation of the physical phenomena (e.g., magnetic reconnection, forces, conversion/transfer of energy between ICME substructures, generation/propagation of plasma waves—see Manchester et al., 2017 for reference) involved in complexity changes observed within ICMEs (e.g., Winslow et al., 2016; Farrugia et al., 2020); 3) complicate the interpretation of ICME kinematics/propagation (e.g., Hess and Zhang, 2014; Lugaz et al., 2020); 4) complicate the identification of large-scale structures interacting with ICMEs (e.g., Scolini et al., 2021); 5) complicate the identification of complexity changes that may have occurred due to the lack of the same type of observations at multiple spacecraft locations (e.g., Scolini et al., 2022b).
Unfortunately, the limited number of spacecraft able to cross individual ICME structures (Lugaz et al., 2018) has prevented the spatial (i.e., longitudinal and latitudinal) characterization of the magnetic complexity distribution within MEs and their radial evolution or temporal changes. This is true both near 1 au, where a maximum of three spacecraft crossing individual ICME structures along different radial directions has been achieved only in the early phases of the STEREO mission at solar minimum (Farrugia et al., 2011; Kilpua et al., 2011; Ruffenach et al., 2012), and also in the inner and outer heliosphere, where measurements are typically rare and single-spacecraft based. Exploiting the ability to simulate spacecraft swarms in global heliospheric simulations, an exploratory numerical investigation by Scolini et al. (2022a) estimated the minimum number of spacecraft that would be required to characterize the spatial distribution of magnetic complexity within MEs and its evolution with heliocentric distance, depending on the ICME propagation scenario (i.e., whether there were interactions with other large-scale solar wind structures). The study revealed that ICME magnetic complexity requires a minimum of ∼10 spacecraft crossings at every 25° to be fully characterized globally. With less spacecraft crossings available, the complexity determined based on in situ data may not be indicative of the actual complexity of the ICME structure as a whole. Interactions with other large-scale solar wind structures such as SIRs and HSSs are also found to increase the minimum number of spacecraft crossings required by a factor of 4–10, bringing it up to a minimum of 50–65 spacecraft at a minimum of 10° of angular separation.
The simulations by Scolini et al. (2022a) also suggest that ICMEs may retain a lower complexity level along their magnetic axis. In this respect, future missions composed of spacecraft swarms orbiting in the ecliptic plane may characterize the complexity evolution of low-inclination ICME flux ropes (i.e., having their magnetic axes approximately aligned with the ecliptic plane; Kilpua et al., 2017) near the ecliptic plane with as little as ∼6 spacecraft crossings, rising to ∼9 in case of interaction with HSSs and SIRs. More such numerical studies are needed to inform preparations for future spacecraft missions to optimize necessary observations and allow for significant progress to be made in this field.
4 Open questions and suggestions for the community
As described above, although a number of recent studies have investigated complexity changes in ICMEs with multi-spacecraft measurements and simulations, many open questions remain. It is important to highlight that most studies on this topic so far have addressed magnetic complexity changes only, not viewing ICME complexity from a holistic standpoint (i.e., looking at both large-scale magnetic configuration changes as well as changes in the plasma characteristics and composition). A holistic view is necessary, however, in order to fully test the hypothesis that ICME complexity generally increases with increasing heliocentric distance. The main limitation to such comprehensive studies is the lack of plasma and magnetic field measurements of the same ICMEs at various radial and longitudinal locations in the inner heliosphere.
Compelling open questions in this area, from a fundamental science perspective, are:
• In general, does the overall complexity of individual ICMEs (including magnetic configuration and plasma characteristics in the different ICME substructures) increase with heliocentric distance in the inner heliosphere? Or is it more common for the ICME internal structure to simplify as it evolves (through Taylor relaxation for example)? Alternatively, is it more likely that different ICME substructures behave differently from each other, i.e., ICME complexity increases in some parts while it decreases in others? Furthermore, does the trend in complexity evolution change as the ICME propagates?
• What are all possible drivers of ICME complexity changes during propagation? Also, what is the main driver?
• To what extent are ICMEs coherent and how does this affect their complexity evolution?
• How do instabilities/small-scale processes contribute to global ICME complexity changes?
• To what degree, if at all, does the presence of a shock/sheath protect the ICME ME from large-scale and comprehensive complexity changes?
• How does ICME–ICME interaction affect the individual ICME’s complexity?
• How do ICMEs of different complexity levels affect the global heliospheric magnetic field and contribute to the heliospheric flux budget?
• Can we leverage proxy observations to gain information about ICME complexity?
Here, we suggest the following ideas to the community to tackle together these compelling open questions:
1) More comprehensive in situ ICME measurements are needed from the Sun to 1 au at multiple heliocentric distances and angular separations. This would entail having magnetic field, solar wind plasma, and suprathermal electron measurements at many locations radially outwards from the Sun. The current standard time resolution of magnetometers and plasma and electron spectrometers at a continuous duty cycle should be sufficient for these studies. Initially, we recommend pathfinder mission(s) combined with simulations allowing for the exploration of the parameter space, while for the following decades, a dedicated flagship mission building on these findings would be necessary to substantially advance our understanding of ICME evolution.
2) Options should be explored on the optimal spacecraft configuration in terms of radial, longitudinal, and latitudinal spacing through simulations to achieve the necessary spatial resolution in the data needed to fully explore ICME complexity evolution in the inner heliosphere.
3) Once the data are available, more comprehensive/holistic studies of ICME complexity evolution are needed, integrating the results from many vantage points.
Exploratory investigations using global heliospheric simulations are already showing us how spacecraft swarms can be best used to investigate the physical origin, evolution, and spatial distribution of magnetic complexity within ICMEs. In the future, we stress that simulations need to be able to resolve more physics (e.g., achieving more accurate characterizations of small-scale phenomena such as magnetic reconnection and shock–ICME interactions), and be able to include more realistic descriptions of the global internal magnetic configuration of MEs, including alterations arising from their early evolution in the solar corona, in order to test such numerical simulations against real ICME events.
Data availability statement
The original contributions presented in the study are included in the article, further inquiries can be directed to the corresponding author.
Author contributions
RW and CS wrote the majority of the manuscript, with input from all other co-authors. All authors provided input and comments on the manuscript.
Funding
RW and ED acknowledges support from NASA grant 80NSSC19K0914. RW, NL, ED, and AG were partially supported by the NASA STEREO grant 80NSSC20K0431. CS acknowledges support from the NASA Living With a Star (LWS) Jack Eddy Postdoctoral Fellowship Program, administered by UCAR’s Cooperative Programs for the Advancement of Earth System Science (CPAESS) under Award No. NNX16AK22G. SP acknowledges support obtained in the framework of the projects C14/19/089 (C1 project Internal Funds KU Leuven), G.0D07.19N (FWO-Vlaanderen), SIDC Data Exploitation (ESA Prodex-12), and Belspo project B2/191/P1/SWiM. EP and TT acknowledge support from NASA grants 80NSSC19K0858, 80NSSC20K1274, and 80NSSC22K0349, as well as from NSF grant ICER-1854790. NL acknowledges support from NASA grant 80NSSC20K0700. LJ thanks the support of NASA STEREO mission and LWS research program. TN-C Thanks the support of NASA Solar Orbiter and Heliophysics Internal Funds (HIF) programs.
Conflict of interest
Authors EP and TT were employed by Predictive Science Inc.
The remaining authors declare that the research was conducted in the absence of any commercial or financial relationships that could be construed as a potential conflict of interest.
Publisher’s note
All claims expressed in this article are solely those of the authors and do not necessarily represent those of their affiliated organizations, or those of the publisher, the editors and the reviewers. Any product that may be evaluated in this article, or claim that may be made by its manufacturer, is not guaranteed or endorsed by the publisher.
References
Al-Haddad, N., Nieves-Chinchilla, T., Savani, N. P., Möstl, C., Marubashi, K., Hidalgo, M. A., et al. (2013). Magnetic field configuration models and reconstruction methods for interplanetary coronal mass ejections. Sol. Phys. 284, 129–149. doi:10.1007/s11207-013-0244-5
Burlaga, L., Sittler, E., Mariani, F., and Schwenn, R. (1981). Magnetic loop behind an interplanetary shock: Voyager, Helios, and IMP 8 observations. J. Geophys. Res. 86, 6673–6684. doi:10.1029/JA086iA08p06673
Cane, H. V., and Richardson, I. G. (2003). Interplanetary coronal mass ejections in the near-Earth solar wind during 1996-2002. J. Geophys. Res. 108, 1156. doi:10.1029/2002JA009817
Davies, E. E., Forsyth, R. J., Good, S. W., and Kilpua, E. K. J. (2020). On the radial and longitudinal variation of a magnetic cloud: ACE, wind, ARTEMIS and juno observations. ARTEMIS Juno Observations 295, 157. doi:10.1007/s11207-020-01714-z
Davies, E. E., Forsyth, R. J., Winslow, R. M., Möstl, C., and Lugaz, N. (2021). A Catalog of Interplanetary Coronal Mass Ejections Observed by Juno between 1 and 5.4 au. Astrophys. J. 923, 136. doi:10.3847/1538-4357/ac2ccb
Davies, E. E., Winslow, R. M., Scolini, C., Forsyth, R. J., Möstl, C., Lugaz, N., et al. (2022). Multi-spacecraft Observations of the Evolution of Interplanetary Coronal Mass Ejections between 0.3 and 2.2 au: Conjunctions with the Juno Spacecraft. Conjunctions Juno Spacecr. 933, 127. doi:10.3847/1538-4357/ac731a
Farrugia, C. J., Berdichevsky, D. B., Möstl, C., Galvin, A. B., Leitner, M., Popecki, M. A., et al. (2011). Multiple, distant (40°) in situ observations of a magnetic cloud and a corotating interaction region complex. J. Atmos. Sol. Terr. Phys. 73, 1254–1269. doi:10.1016/j.jastp.2010.09.011
Farrugia, C. J., Lugaz, N., Vasquez, B. J., Wilson, L. B., Yu, W., Paulson, K., et al. (2020). A study of a magnetic cloud propagating through large-amplitude alfvén waves. J. Geophys. Res. Space Phys. 125, e2019JA027638. doi:10.1029/2019JA027638
Florido-Llinas, M., Nieves-Chinchilla, T., and Linton, M. G. (2020). Analysis of the helical kink stability of differently twisted magnetic flux ropes. Sol. Phys. 295, 118. doi:10.1007/s11207-020-01687-z
Gonzalez, W. D., de Gonzalez, A. L. C., Dal Lago, A., Tsurutani, B. T., Arballo, J. K., Lakhina, G. K., et al. (1998). Magnetic cloud field intensities and solar wind velocities. Geophys. Res. Lett. 25, 963–966. doi:10.1029/98GL00703
Good, S. W., Ala-Lahti, M., Palmerio, E., Kilpua, E. K. J., and Osmane, A. (2020). Radial evolution of magnetic field fluctuations in an interplanetary coronal mass ejection sheath. ApJ. 893, 110. doi:10.3847/1538-4357/ab7fa2
Good, S. W., Forsyth, R. J., Eastwood, J. P., and Möstl, C. (2018). Correlation of ICME magnetic fields at radially aligned spacecraft. Sol. Phys. 293, 52. doi:10.1007/s11207-018-1264-y
Good, S. W., and Forsyth, R. J. (2016). Interplanetary coronal mass ejections observed by MESSENGER and Venus express. Sol. Phys. 291, 239–263. doi:10.1007/s11207-015-0828-3
Good, S. W., Forsyth, R. J., Raines, J. M., Gershman, D. J., Slavin, J. A., and Zurbuchen, T. H. (2015). Radial evolution of a magnetic cloud: MESSENGER, STEREO, and Venus express observations. Astrophys. J. 807, 177. doi:10.1088/0004-637X/807/2/177
Gosling, J. T., Baker, D. N., Bame, S. J., Feldman, W. C., Zwickl, R. D., and Smith, E. J. (1987). Bidirectional solar wind electron heat flux events. J. Geophys. Res. 92, 8519–8535. doi:10.1029/JA092iA08p08519
Hess, P., and Zhang, J. (2014). Stereoscopic study of the kinematic evolution of a coronal mass ejection and its driven shock from the Sun to the Earth and the prediction of their arrival times. Astrophys. J. 792, 49. doi:10.1088/0004-637X/792/1/49
Isavnin, A., Vourlidas, A., and Kilpua, E. K. J. (2014). Three-Dimensional evolution of flux-rope CMEs and its relation to the local orientation of the heliospheric current sheet. Sol. Phys. 289, 2141–2156. doi:10.1007/s11207-013-0468-4
Janvier, M., Winslow, R. M., Good, S., Bonhomme, E., Démoulin, P., Dasso, S., et al. (2019). Generic magnetic field intensity profiles of interplanetary coronal mass ejections at Mercury, Venus, and Earth from superposed epoch analyses. J. Geophys. Res. Space Phys. 124, 812–836. doi:10.1029/2018JA025949
Jian, L., Russell, C. T., Luhmann, J. G., and Skoug, R. M. (2006). Properties of interplanetary coronal mass ejections at one AU during 1995 2004. Sol. Phys. 239, 393–436. doi:10.1007/s11207-006-0133-2
Jones, S. R., Scott, C. J., Barnard, L. A., Highfield, R., Lintott, C. J., and Baeten, E. (2020). The visual complexity of coronal mass ejections follows the solar cycle. Space weather. 18, e2020SW002556. doi:10.1029/2020SW002556
Kahler, S. W., and Reames, D. V. (1991). Probing the magnetic topologies of magnetic clouds by means of solar energetic particles. J. Geophys. Res. 96, 9419–9424. doi:10.1029/91JA00659
Kay, C., and Opher, M. (2015). The heliocentric distance where the deflections and rotations of solar coronal mass ejections occur. Astrophys. J. Lett. 811, L36. doi:10.1088/2041-8205/811/2/L36
Kilpua, E. K. J., Jian, L. K., Li, Y., Luhmann, J. G., and Russell, C. T. (2011). Multipoint ICME encounters: Pre-STEREO and STEREO observations. J. Atmos. Sol.-Terr. Phys. 73, 1228–1241. doi:10.1016/j.jastp.2010.10.012
Kilpua, E., Koskinen, H. E. J., and Pulkkinen, T. I. (2017). Coronal mass ejections and their sheath regions in interplanetary space. Living Rev. Sol. Phys. 14, 5. doi:10.1007/s41116-017-0009-6
Lavraud, B., and Rouillard, A. (2014). Properties and processes that influence CME geo-effectiveness. Nat. Prominences their Role Space Weather 300, 273–284. doi:10.1017/S1743921313011095
Lavraud, B., Ruffenach, A., Rouillard, A. P., Kajdic, P., Manchester, W. B., and Lugaz, N. (2014). Geo-effectiveness and radial dependence of magnetic cloud erosion by magnetic reconnection. J. Geophys. Res. Space Phys. 119, 26–35. doi:10.1002/2013JA019154
Liu, Y., Shen, F., and Yang, Y. (2019). Numerical simulation on the propagation and deflection of fast coronal mass ejections (CMEs) interacting with a corotating interaction region in interplanetary space. Astrophys. J. 887, 150. doi:10.3847/1538-4357/ab543e
Lugaz, N., Farrugia, C. J., Winslow, R. M., Al-Haddad, N., Galvin, A. B., Nieves-Chinchilla, T., et al. (2018). On the Spatial Coherence of Magnetic Ejecta: Measurements of Coronal Mass Ejections by Multiple Spacecraft Longitudinally Separated by 0.01 au. Astrophys. J. Lett. 864, L7. doi:10.3847/2041-8213/aad9f4
Lugaz, N., Temmer, M., Wang, Y., and Farrugia, C. J. (2017). The interaction of successive coronal mass ejections: A review. Sol. Phys. 292, 64. doi:10.1007/s11207-017-1091-6
Lugaz, N., Winslow, R. M., and Farrugia, C. J. (2020). Evolution of a long-duration coronal mass ejection and its sheath region between Mercury and Earth on 9-14 july 2013. J. Geophys. Res. Space Phys. 125, e27213. doi:10.1029/2019JA027213
Luhmann, J. G., Gopalswamy, N., Jian, L. K., and Lugaz, N. (2020). ICME evolution in the inner heliosphere. Sol. Phys. 295, 61. doi:10.1007/s11207-020-01624-0
Manchester, W. B., Gombosi, T. I., Roussev, I., de Zeeuw, D. L., Sokolov, I. V., Powell, K. G., et al. (2004). Three-dimensional MHD simulation of a flux rope driven CME. J. Geophys. Res. 109, A01102. doi:10.1029/2002JA009672
Manchester, W., Kilpua, E. K. J., Liu, Y. D., Lugaz, N., Riley, P., Török, T., et al. (2017). The physical processes of CME/ICME evolution. Space Sci. Rev. 212, 1159–1219. doi:10.1007/s11214-017-0394-0
Möstl, C., Farrugia, C. J., Kilpua, E. K. J., Jian, L. K., Liu, Y., Eastwood, J. P., et al. (2012). Multi-point shock and flux rope analysis of multiple interplanetary coronal mass ejections around 2010 august 1 in the inner heliosphere. Astrophys. J. 758, 10. doi:10.1088/0004-637X/758/1/10
Nakwacki, M. S., Dasso, S., Démoulin, P., Mandrini, C. H., and Gulisano, A. M. (2011). Dynamical evolution of a magnetic cloud from the Sun to 5.4 AU. Astron. Astrophys. 535, A52. doi:10.1051/0004-6361/201015853
Nieves-Chinchilla, T., Colaninno, R., Vourlidas, A., Szabo, A., Lepping, R. P., Boardsen, S. A., et al. (2012). Remote and in situ observations of an unusual Earth-directed coronal mass ejection from multiple viewpoints. J. Geophys. Res. 117, A06106. doi:10.1029/2011JA017243
Nieves-Chinchilla, T., Jian, L. K., Balmaceda, L., Vourlidas, A., dos Santos, L. F. G., and Szabo, A. (2019). Unraveling the internal magnetic field structure of the earth-directed interplanetary coronal mass ejections during 1995 - 2015. Sol. Phys. 294, 89. doi:10.1007/s11207-019-1477-8
Nieves-Chinchilla, T., Linton, M. G., Hidalgo, M. A., Vourlidas, A., Savani, N. P., Szabo, A., et al. (2016). A circular-cylindrical flux-rope analytical model for magnetic clouds. Astrophys. J. 823, 27. doi:10.3847/0004-637X/823/1/27
Nieves-Chinchilla, T., Vourlidas, A., Raymond, J. C., Linton, M. G., Al-haddad, N., Savani, N. P., et al. (2018). Understanding the internal magnetic field configurations of ICMEs using more than 20 Years of wind observations. Sol. Phys. 293, 25. doi:10.1007/s11207-018-1247-z
Odstrčil, D., and Pizzo, V. J. (1999a). Distortion of the interplanetary magnetic field by three-dimensional propagation of coronal mass ejections in a structured solar wind. J. Geophys. Res. 104, 28225–28239. doi:10.1029/1999JA900319
Odstrčil, D., and Pizzo, V. J. (1999b). Three-dimensional propagation of coronal mass ejections (CMEs) in a structured solar wind flow: 1. CME launched within the streamer belt. J. Geophys. Res. 104, 483–492. doi:10.1029/1998JA900019
Odstrčil, D., and Pizzo, V. J. (1999c). Three-dimensional propagation of coronal mass ejections (CMEs) in a structured solar wind flow: 2. CME launched adjacent to the streamer belt. J. Geophys. Res. 104, 493–503. doi:10.1029/1998JA900038
Owens, M. J., and Cargill, P. J. (2002). Correlation of magnetic field intensities and solar wind speeds of events observed by ACE. J. Geophys. Res. 107, 1050. doi:10.1029/2001JA000238
Owens, M. J., Cargill, P. J., Pagel, C., Siscoe, G. L., and Crooker, N. U. (2005). Characteristic magnetic field and speed properties of interplanetary coronal mass ejections and their sheath regions. J. Geophys. Res. 110, A01105. doi:10.1029/2004JA010814
Owens, M. J., Lockwood, M., and Barnard, L. A. (2017). Coronal mass ejections are not coherent magnetohydrodynamic structures. Sci. Rep. 7, 4152. doi:10.1038/s41598-017-04546-3
Palmerio, E., Nieves-Chinchilla, T., Kilpua, E. K. J., Barnes, D., Zhukov, A. N., Jian, L. K., et al. (2021). Magnetic structure and propagation of two interacting CMEs from the Sun to Saturn. J. Geophys. Res. (Space Phys. 126, e2021JA029770. doi:10.1029/2021JA029770
Richardson, I. G., and Cane, H. V. (2010). Near-earth interplanetary coronal mass ejections during solar cycle 23 (1996 - 2009): Catalog and summary of properties. Sol. Phys. 264, 189–237. doi:10.1007/s11207-010-9568-6
Riley, P., Linker, J. A., Lionello, R., Mikić, Z., Odstrcil, D., Hidalgo, M. A., et al. (2004). Fitting flux ropes to a global MHD solution: A comparison of techniques. J. Atmos. Sol.-Terr. Phys. 66, 1321–1331. doi:10.1016/j.jastp.2004.03.019
Rodriguez, L., Masías-Meza, J. J., Dasso, S., Démoulin, P., Zhukov, A. N., Gulisano, A. M., et al. (2016). Typical profiles and distributions of plasma and magnetic field parameters in magnetic clouds at 1 AU. Sol. Phys. 291, 2145–2163. doi:10.1007/s11207-016-0955-5
Ruffenach, A., Lavraud, B., Farrugia, C. J., Démoulin, P., Dasso, S., Owens, M. J., et al. (2015). Statistical study of magnetic cloud erosion by magnetic reconnection. J. Geophys. Res. Space Phys. 120, 43–60. doi:10.1002/2014JA020628
Ruffenach, A., Lavraud, B., Owens, M. J., Sauvaud, J. A., Savani, N. P., Rouillard, A. P., et al. (2012). Multispacecraft observation of magnetic cloud erosion by magnetic reconnection during propagation. J. Geophys. Res. Space Phys. 117, A09101. doi:10.1029/2012JA017624
Savani, N. P., Owens, M. J., Rouillard, A. P., Forsyth, R. J., and Davies, J. A. (2010). OBSERVATIONAL EVIDENCE OF a CORONAL MASS EJECTION DISTORTION DIRECTLY ATTRIBUTABLE TO a STRUCTURED SOLAR WIND. Astrophysical J. 714, L128–L132. doi:10.1088/2041-8205/714/1/l128
Scolini, C., Winslow, R. M., Lugaz, N., and Poedts, S. (2022a). Characteristic scales of complexity and coherence within interplanetary coronal mass ejections: Insights from spacecraft swarms in global heliospheric simulations. arXiv.
Scolini, C., Winslow, R. M., Lugaz, N., and Poedts, S. (2021). Evolution of interplanetary coronal mass ejection complexity: A numerical study through a swarm of simulated spacecraft. Astrophys. J. Lett. 916, L15. doi:10.3847/2041-8213/ac0d58
Scolini, C., Winslow, R. M., Lugaz, N., Salman, T. M., Davies, E. E., and Galvin, A. B. (2022b). Causes and consequences of magnetic complexity changes within interplanetary coronal mass ejections: A statistical study. Astrophys. J. 927, 102. doi:10.3847/1538-4357/ac3e60
Shodhan, S., Crooker, N. U., Kahler, S. W., Fitzenreiter, R. J., Larson, D. E., Lepping, R. P., et al. (2000). Counterstreaming electrons in magnetic clouds. J. Geophys. Res. 105, 27261–27268. doi:10.1029/2000JA000060
Temmer, M., Holzknecht, L., Dumbović, M., Vršnak, B., Sachdeva, N., Heinemann, S. G., et al. (2021). Deriving CME density from remote sensing data and comparison to in situ measurements. J. Geophys. Res. Space Phys. 126, e28380. doi:10.1029/2020JA028380
Török, T., Downs, C., Linker, J. A., Lionello, R., Titov, V. S., Mikić, Z., et al. (2018). Sun-to-Earth MHD simulation of the 2000 july 14 “bastille day” eruption. Astrophys. J. 856, 75. doi:10.3847/1538-4357/aab36d
Verbeke, C., Schmieder, B., Démoulin, P., Dasso, S., Grison, B., Samara, E., et al. (2022). Over-expansion of coronal mass ejections modelled using 3D MHD EUHFORIA simulations. Adv. Space Res. 70, 1663–1683. doi:10.1016/j.asr.2022.06.013
Wang, Y., Wang, B., Shen, C., Shen, F., and Lugaz, N. (2014). Deflected propagation of a coronal mass ejection from the corona to interplanetary space. J. Geophys. Res. Space Phys. 119, 5117–5132. doi:10.1002/2013JA019537
Winslow, R. M., Lugaz, N., Philpott, L. C., Schwadron, N. A., Farrugia, C. J., Anderson, B. J., et al. (2015). Interplanetary coronal mass ejections from MESSENGER orbital observations at Mercury. J. Geophys. Res. Space Phys. 120, 6101–6118. doi:10.1002/2015JA021200
Winslow, R. M., Lugaz, N., Schwadron, N. A., Farrugia, C. J., Yu, W., Raines, J. M., et al. (2016). Longitudinal conjunction between MESSENGER and STEREO A: Development of ICME complexity through stream interactions. J. Geophys. Res. Space Phys. 121, 6092–6106. doi:10.1002/2015JA022307
Winslow, R. M., Lugaz, N., Scolini, C., and Galvin, A. B. (2021a). First simultaneous in situ measurements of a coronal mass ejection by parker solar Probe and STEREO-A. Astrophys. J. 916, 94. doi:10.3847/1538-4357/ac0821
Winslow, R. M., Scolini, C., Jian, L. K., Nieves-Chinchilla, T., Temmer, M., Carcaboso, F., et al. (2022). On the importance of investigating CME complexity evolution during interplanetary propagation. Bullet. Amer. Astron. Soc.
Winslow, R. M., Scolini, C., Lugaz, N., and Galvin, A. B. (2021b). The effect of stream interaction regions on ICME structures observed in longitudinal conjunction. Astrophys. J. 916, 40. doi:10.3847/1538-4357/ac0439
Zhou, Y., and Feng, X. (2017). Numerical study of the propagation characteristics of coronal mass ejections in a structured ambient solar wind. J. Geophys. Res. Space Phys. 122, 1451–1462. doi:10.1002/2016JA023053
Keywords: CME, heliosphere, magnetic ejecta, flux rope, Sun
Citation: Winslow RM, Scolini C, Jian LK, Nieves-Chinchilla T, Temmer M, Carcaboso F, Schmieder B, Poedts S, Lynch BJ, Wood BE, Palmerio E, Lugaz N, Farrugia CJ, Lee CO, Davies EE, Regnault F, Salman TM, Török T, Al-Haddad N, Vourlidas A, Manchester WB, Jin M, Lavraud B and Galvin AB (2022) On the importance of investigating CME complexity evolution during interplanetary propagation. Front. Astron. Space Sci. 9:1064175. doi: 10.3389/fspas.2022.1064175
Received: 07 October 2022; Accepted: 09 December 2022;
Published: 23 December 2022.
Edited by:
Julio Navarro, University of Victoria, CanadaReviewed by:
Shan Wang, University of Maryland, College Park, United StatesCopyright © 2022 Winslow, Scolini, Jian, Nieves-Chinchilla, Temmer, Carcaboso, Schmieder, Poedts, Lynch, Wood, Palmerio, Lugaz, Farrugia, Lee, Davies, Regnault, Salman, Török, Al-Haddad, Vourlidas, Manchester, Jin, Lavraud and Galvin. This is an open-access article distributed under the terms of the Creative Commons Attribution License (CC BY). The use, distribution or reproduction in other forums is permitted, provided the original author(s) and the copyright owner(s) are credited and that the original publication in this journal is cited, in accordance with accepted academic practice. No use, distribution or reproduction is permitted which does not comply with these terms.
*Correspondence: Réka M. Winslow, cmVrYS53aW5zbG93QHVuaC5lZHU=