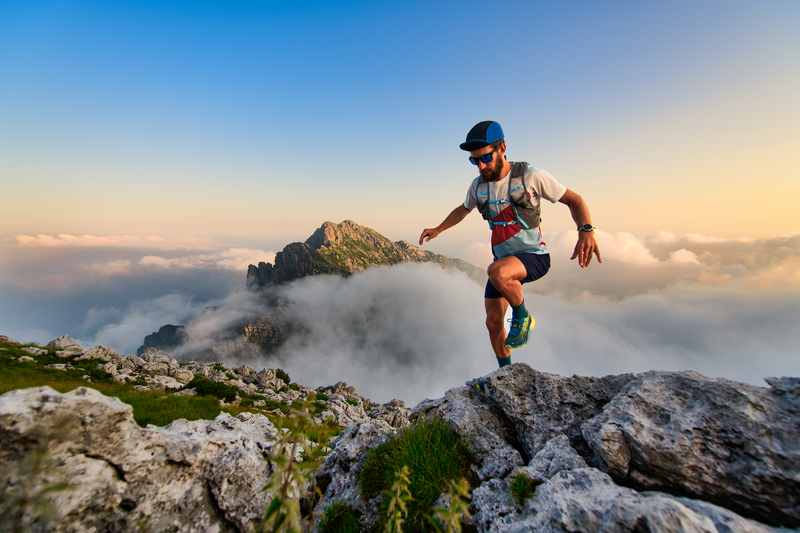
95% of researchers rate our articles as excellent or good
Learn more about the work of our research integrity team to safeguard the quality of each article we publish.
Find out more
PERSPECTIVE article
Front. Astron. Space Sci. , 02 December 2022
Sec. Stellar and Solar Physics
Volume 9 - 2022 | https://doi.org/10.3389/fspas.2022.1063995
This article is part of the Research Topic Planetary Nebulae as Tools for Astrophysics View all 8 articles
I relate an anecdotal history of the beginnings of a new initiative in studies of the chemical composition of planetary nebulae: namely, investigating abundances of elements beyond the iron-peak through infrared spectroscopy. In some planetary nebulae many of these elements display enrichments due to neutron capture reactions within the progenitor stars. This snippet of history provides an example of how science advances from the convergence of improvements in instrumentation, cross-over between fields, and constructive interaction between theory and observation. It also highlights the fact that terra incognita and discovery space remains even in seemingly well-established fields of science.
Stars alter the chemical composition of the Universe by hosting nuclear reactions in their interiors and subsequently finding ways to return the resulting chemically-altered material to their interstellar environments. The latter happens through stellar winds and more spectacular final stages of metal-rich outflows, namely supernova remnants and planetary nebulae (PNe). As agents of chemical enrichment, each lower mass star produces a smaller total amount of newly synthesized elements than an individual high mass star does, but the lower mass stars are collectively significant contributors since they are more prevalent. They are major sources of specific elements including C, N, and certain trans-iron nuclides produced by the type of neutron-capture reactions called the slow or s-process because it proceeds by adding neutrons to nuclei at intervals long enough to allow intermediate products to beta-decay prior to the next neutron capture (Käppeler et al., 2011). Low to intermediate mass stars provide one of the environments where such reactions can occur during their Asymptotic Giant Branch (AGB) phase of evolution (Busso et al., 1999; Karakas & Lattanzio 2014).
AGB stars have been known to be sites of the s-process as designated by B2FH (Burbidge et al., 1957) since the discovery of “live,” short-lived (radioactive) Technetium in Carbon stars by Merrill (1952). Enrichments of many other s-process products attributed to in situ synthesis have been detected in C-rich AGB stars analyzed in detail (e.g. Lambert et al., 1995; Abia et al., 2002, among many other works). PNe are constituted of the envelopes of former AGB stars, after multiple episodes of deep mixing driven by convection homogenize their composition, “salting” the surface with new atoms of trans-iron elements (Busso et al., 1999). Since PNe provide a direct view of these evolved stars caught in the act of enriching their environments in the products of stellar synthesis, by measuring the amounts of these species in PNe we empirically and quantitatively establish the roles of AGB stars as agents of galactic chemical evolution.
The first serious effort to seek signatures of the s-process in PNe was made by Péquignot and Baluteau (1994, hereafter PB94). They proposed assignments of many weak emission features in the spectrum of the bright PN NGC 7027 to transitions of elements in Rows 4, 5, and 6 of the Periodic Table, with confidence levels ranging from “definite,” to “possible,” to “hint.” This work was presented by Daniel Péquignot at the IAU Symposium 180 on Planetary Nebulae in Gröningen, Netherlands, in August 1996 (Péquignot 1997; Péquignot & Baluteau 1997). His proposal was received with considerable interest, yet skepticism that was not entirely unjustified. As PB94 point out, many of the suspected features from trans-iron elements could instead be intrinsically weak features of more abundant species, for example the copious population of permitted lines produced during the recombination cascades of abundant elements such as CNO, Ne, Si, etc. However, despite such reservations, a seed had been planted in many minds.
I was intrigued by the possibility of detecting elements with heavy atomic masses, very low abundances relative to hydrogen, and obscure names, in PNe. It was a tantalizing prospect, although one could hardly hope to match the cornucopia of 52 elements detected via absorption lines in the very metal-poor star CS 22892-052 (e.g. Sneden et al., 2003). I went so far as to obtain an optical spectrum of NGC 7027 in 1998, with my colleague and stellar abundance aficionado Chris Sneden, using the newly-launched 10 m Hobby-Eberly Telescope (HET) in west Texas. At the time, the HET was temporarily furnished with an existing medium resolution spectrometer while the custom-designed first-generation instruments for the HET were under development. The HET spectrum confirmed the claimed detection by PB94 of a (Kr III) line at 6,826 Å, but we deemed it unpublishable since it did not add significant new information.
From graduate school through my first postdoctoral position as a National Research Council Fellow at NASA Ames Research Center, I had studied fine-structure emission lines of more abundant ions in the mid (Dinerstein 1980) and far infrared (Dinerstein et al., 1985). The mid infrared hosts fine structure lines of elements in Row three of the Periodic Table (e.g. S, Cl, and Ar), plus Ne at the heavy end of Row 2, while lighter species in Row two such as C, N, and O tend to have lines in the far infrared (50–200 μm). Following a trend of larger energy level spacings for elements with higher nuclear charge, the fine-structure transitions of heavier species lie mostly in the near infrared. Infrared emission lines from low-lying energy states have advantages over optical transitions as tools for determining ionic and elemental abundances. The electron populations in the excited fine-structure levels of the ground term are considerably greater and less sensitive to temperature than those in higher energy levels from which optical lines originate (e.g., Dinerstein 1995). In addition, some ions do not possess any optical transitions, notably “two-level atoms” with only one low energy excited level and hence only one transition in the ground term. Examples include n p, n p5, n d, and n d9 species, where n is the principal quantum number of the outermost shell. These configurations are particularly favorable for observation since their excited electrons are consolidated into a single level. The next best cases are species for which the lowest term is a triplet with closely spaced energies, such as n p2 and n p4 ions.
The optical emission lines highlighted by PB94 are relatively weak due to the small populations in the relatively high-energy upper levels of the transitions. Why not look in the infrared for the corresponding fine-structure lines of the ground terms, which are likely to be stronger?
This thought intersected in an unexpected way with my long-term interest in the neutral and molecular components of PNe (Dinerstein 1991). Near-infrared vibration-rotation transitions of the ground electronic state of H2 were first detected in the PN NGC 7027 by Treffers et al. (1976). The predominant excitation mechanisms for these lines are collisional excitation in a warm thermalized gas (e.g., heated by shocks), and radiative excitation via absorption of 11.3–13.6 eV photons in the FUV Lyman-Werner bands of H2 followed by a radiative cascade (“fluorescence”). Initially it seemed easy to distinguish between these possibilities due to the sharp dichotomy in the character of their spectra. A fluorescent spectrum displays a rich array of emission lines from highly excited (v > 2) levels that are unreachable by collisions in gas that is cool enough to avoid dissociating H2 (e.g., Black and van Dishoeck 1987). The reason to care about which mechanism is responsible is that since radiative excitation is relatively inefficient, it takes much less molecular mass to produce as intense emission in key vibration-rotation lines from thermally-emitting H2 than through radiative excitation. Therefore, identifying the excitation mechanism as fluorescence would indicate the presence of large amounts of molecular material in PNe. Later the picture became less bifurcated, when additional factors such as collisional modification of the level populations in dense radiatively excited H2 (Sternberg & Dalgarno 1989) or time-dependent effects (e.g., Bertoldi and Draine 1996) were included in models.
During the mid-1980s I was using the 2.7 m Harlan J. Smith Telescope at the University of Texas’ McDonald Observatory to examine the H2 emission spectra of PNe. In some but not all cases, it was possible to observe a spatial shift between the ionized gas and the excited H2, due to the commonly stratified structure of the photodissociation region (PDR), outside the H II zone (Hollenbach and Tielens 1997). By serendipity, I chose to observe the relatively obscure PN Hubble 12 (Hb 12). I arbitrarily started mapping beyond its compact ionized core by moving to the east (these were the days of single-beam mapping, long before the introduction of integral field unit spectroscopy), and stumbled upon a peak of H2 emission, about 4″ E of the central ionized nebula, that displayed a fluorescent spectrum (Dinerstein et al., 1988). For a brief period, Hb 12 became a favorite target for studying radiatively excited H2 (Ramsay et al., 1993; Luhman and Rieke 1996). Although it has again sunk into relative obscurity, Hb 12 shows one of the most remarkably fluorescent H2 spectra known (Dinerstein et al., 2015; Kaplan et al., 2016).
A few years later, McDonald Observatory acquired a new and more capable near-infrared spectrometer, CoolSpec (Lester et al., 2000). In collaboration with Lauren Likkel (Univ. of Wisconsin, Eau Claire), we resumed investigating H2 emission from PNe. This survey (Likkel et al., 2006) found mixed excitation indicators for the H2. In order to more cleanly distinguish fluorescent from shock excitation, we focused on the K-band lines from vibrational level v = 3. Although at first taking their strengths in some PNe as indicators of fluorescence, I soon became aware of the interfering presence of two unidentified lines at 2.199 and 2.287 μm. The latter had been noted by Treffers et al. (1976), the discovery paper for the H2 emission, and is seen in many PNe including some that showed no other H2 lines. Geballe et al. (1991) discussed these lines, established that they could not be the v = 3–2 S(2) and S(3) lines that are nearly coincident in wavelength with the mystery lines, and probably arose from an ionized metal species, although they failed to find an identification among elements up through Zn (element 30).
Returning to the quest for the fine-structure lines corresponding to the optical emission lines suspected of originating from trans-iron elements, a good place to start was Kr (atomic number 36), the element responsible for the [Kr IV] lines cited as the strongest of these features by PB94. One morning in early December of 2000, when I had procured a rare semester’s leave from teaching, I sat down in our department’s private reading room (Péridier Library, now replaced by a computer lab) with a dusty volume of Atomic Energy Levels (Moore 1952). I looked through the ground-configuration energy levels of the lowest few ions of Kr. To my shock, I found that the transition from the first excited level to the ground level in Kr+2 had exactly the right energy to be responsible for the unidentified line at 2.199 μm. Since the line strength might have been enhanced by synthesis of Kr during the s-process, here was a plausible identification at last!
This was the most dramatic instance of the answer to a puzzle suddenly falling into place that I have experienced; both surprising and yet essentially expected. I call it my “nebulium” moment. Such an obscure reference requires me to fill in the backstory about line identifications in astronomical spectra. The first part might be familiar: the discovery of helium through its prominent yellow emission line at 5876 Å in the solar chromosphere (independently) by Janssen and Lockyer in 1868 (Aldersey-Williams, 2012, pp. 191-92). The designation “helium” came from its discovery site, and it was not until 1895 that the same emission line was observed by Ramsay from helium outgassed from terrestrial minerals (Aldersey-Williams, 2012, p. 332).
By analogy with helium, “nebulium” was proposed as the species responsible for a pair of strong green emission lines near Hβ, seen for the first time in the PN NGC 6543 (now widely known as the “Cat’s Eye Nebula”). This discovery was made by William Huggins in 1864, and was secondary only to his realization that, since the spectrum showed emission lines, it must be “… not an aggregation of stars, but a luminous gas” (Glass 2006, p. 130). I first became aware that the green lines, later found to be [O III] 4959 and 5007 Å, were discovered in NGC 6543 from the first paragraph of a paper by Czyzak et al. (1968). That led me eventually to personally track down and read the original article (Huggins and Miller 1864) in a bound volume of the proceedings of the Royal Academy, while sitting in a small, wood-paneled reading room of the main Public Library on 42nd Street in New York City (my home town, as it happens). I had to do a quick calculation to verify the identity of the PN, since the latitude coordinate was given in north polar distance rather than declination! I later read the identification paper (Bowen 1927), published 63 years after discovery of these lines. There I discovered that not only had Bowen reasoned that those lines arise from metastable energy levels of doubly-ionized oxygen, but he also identified the optical [O II] and [N II] lines also seen in nebulae. These so-called “strong” optical lines are used today to determine metallicities in the high-redshift Universe, where they originate from H II regions lit up by hot, massive young stars in star-forming galaxies (e.g., Kewley et al., 2013).
The identity of the infrared [Kr III] line was checked by comparing its strength to that of [Kr III] 6,826 Å using atomic data from Schöning (1997) and finding that the intensity ratio of the two lines was consistent with theoretical predictions. My approach to identifying the 2.287 μm line was to assume “Kr-adjacency.” I looked for another, nearby (in the Periodic Table) element also produced in the s-process and with an even atomic number (in view of the odd-even effect that causes even-numbered elements to be more abundant than odd-numbered ones), and fairly quickly found that there is a [Se IV] line with a wavelength close to 2.287 μm. A precise check was made by contacting Yogi Joshi, an atomic physicist at St. Francis Xavier University in Canada, who kindly provided more exact values of the energy levels (private communication). It was not possible to check the identification with another line from the same ion, since Se+3 is a “two-level atom” as described above and has no other transitions in its ground configuration.
The identifications of the [Kr III] and [Se IV] lines were published in Dinerstein (2001), in the 1 April issue, which might have led to suspicions that it was an April Fool’s joke. (At least one possibly skeptical author subsequently attributed it to [K III]!) This also implied that there were probably other lines of trans-iron elements in the infrared. While the first two lines were found through serendipity, from that point on the strategy became a targeted search for lines that were anticipated to be detectable. In fall 2001 I was joined in this endeavor by N. C. Sterling, who initially was my Ph.D. advisee, and continues to be a valued collaborator of mine and a leader in this field. His survey of over 100 Galactic PNe in Se and Kr (Sterling and Dinerstein 2008; Sterling et al., 2015) remains the largest-scale survey of neutron-capture elements in PNe.
Nature places atomic transitions wherever they happen to land in wavelength, not for the convenience of astronomers. Since there is no panchromatic instrument that covers the entire electromagnetic spectrum, if the goal is to pursue lines from elements of interest wherever they reside, one must be prepared to utilize a variety of instruments across a range of spectral regions.
The arrival of a new generation of sensitive, high-spectral resolution infrared spectrometers around 2014 prompted great advances. At the University of Texas’ McDonald Observatory we began using the Immersion Grating Infrared Spectrometer, IGRINS (Park et al., 2014) on the 2.7 m telescope. We quickly found three new lines, weaker than the Se and Kr lines (Sterling et al., 2016), from the elements Ge (element 32), Rb (37), and Cd (48). Using IGRINS, and EMIR (Garzón et. al., 2016) on the Gran Telescopio Canarias on La Palma, we detected lines of Br (35) and Te (52) (Madonna et al., 2018). With the Habitable-zone Planet Finder HPF (Mahadevan et al., 2014) on the 10 m Hobby-Eberly Telescope in west Texas, we discovered lines from a second ion of Te and two ions of Xe (54) (Dinerstein et al., 2022). Finally, using iSHELL (Rayner et al., 2012) on the IRTF, NASA’s 3 m Infrared Telescope Facility on Maunakea, we detected lines from additional ions of Br and Rb (Dinerstein et al., 2021). These new lines were discovered at spectral resolving powers of R ≥ 30,000, rendering even weak lines visible against the continuum. Meanwhile, detections of optical emission lines of trans-iron elements have been accumulating in the literature (e.g., Sterling 2020; Kwitter and Henry 2022; Manea et al., 2022).
The role of theory should not be overlooked, as it provides an invaluable guiding hand for observing strategies. Models of the evolution and s-process nucleosynthesis of AGB stars tell us which elements are likely to be enriched and by what factor. These depend on such parameters as stellar mass and metallicity, and the treatment of convection and other mixing processes. Groups using different approaches to these simulations find different predicted enrichments for some species (e.g., Cristallo et al., 2015; Karakas and Lugaro 2016; Busso et al., 2021; Yagüe López et al., 2022). Observations of PNe can provide leverage for selecting among these alternatives.
Additionally, atomic data from theoretical and experimental studies are needed to translate line strengths into ionic abundances, and are often not available in the literature for the species we observe. Important parameters include accurate energy level differences, transition probabilities, and collision strengths. For elemental abundances, we need to correct for the portion of the element in unobserved ions. Methods for making such corrections include solving the detailed ionization structure of a nebula using nebular modeling codes such as Cloudy (Ferland et al., 2017), or adopting formulations based on grids of such models (e.g., Delgado-Inglada et al., 2014; Sterling et al., 2015). Both approaches rely on additional atomic data: photoionization cross-sections, recombination coefficients, and other microphysical parameters.
Today we are entering an era when infrared spectroscopy will again open up less-explored spectral regions: segments of wavelength space that are blocked by the Earth’s atmosphere. The spectrometers on the James Webb Space Telescope have lower spectral resolving power than those on many ground-based facilities, but may yet reveal new insights, while observations with ground-based telescopes and instruments will continue to develop new capacity for discovery.
The data described here have been previously published in the cited references. Further inquiries should be directed to the corresponding author.
HLD is fully responsible for the content of this article.
The author's research in this field is supported by NSF grant AST-1715332, and was previously support by NSF grants AST-0406809 and AST-0708245.
I have been fortunate to have worked on the topic of s-process enrichments in planetary nebulae with many excellent collaborators and students including (in roughly chronological order) C. Sneden, T. R. Geballe, N. C. Sterling and K. F. Kaplan (formerly graduate students at the University of Texas), K. Butler, A. Karakas, M. Lugaro, M. Bautista, S. Madonna, W. Vacca, C. Manea, M. Stephenson, and others.
The author declares that the research was conducted in the absence of any commercial or financial relationships that could be construed as a potential conflict of interest.
All claims expressed in this article are solely those of the authors and do not necessarily represent those of their affiliated organizations, or those of the publisher, the editors and the reviewers. Any product that may be evaluated in this article, or claim that may be made by its manufacturer, is not guaranteed or endorsed by the publisher.
Abia, C., Domínguez, I., Gallino, R., Busso, M., Masera, S., Straniero, O., et al. (2002). s-Process nucleosynthesis in Carbon stars. Astrophys. J. 579, 817–831. doi:10.1086/342924
Aldersey-Williams, M. (2012). Periodic tales: A cultural history of the elements, from arsenic to zinc. New York: Harper Collins Publishers.
Bertoldi, F., and Draine, B. T. (1996). Nonequilibrium photodissociation regions: Ionization-dissociation fronts. Astrophys. J. 458, 222–232. doi:10.1086/176805
Black, J. H., and van Dishoeck, E. F. (1987). Fluorescent excitation of interstellar H2. Astrophys. J. 322, 412–449. doi:10.1086/165740
Burbidge, E. M., Burbidge, G. R., Fowler, W. A., and Hoyle, F. (1957). Synthesis of the elements in stars. Rev. Mod. Phys. 29, 547–650. doi:10.1103/RevModPhys.29.547
Busso, M., Gallino, R., and Wasserburg, G. J. (1999). Nucleosynthesis in Asymptotic Giant Branch stars: Relevance for galactic enrichment and solar system formation. Annu. Rev. Astron. Astrophys. 37, 239–309. doi:10.1146/annurev.astro.37.1.239
Busso, M., Vescovi, D., Palmerini, S., Cristallo, S., and Antonuccio-Delogu, V. (2021). S-Processing in AGB stars revisited. III. Neutron captures from MHD mixing at different metallicities and observational constraints. Astrophys. J. 908 (55), 55. doi:10.3847/1538-4357/abca8e
Cristallo, S., Straniero, O., Piersanti, L., and Gobrecht, G. (2015). Evolution, nucleosynthesis, and yields of AGB stars at different metallicities. III. Intermediate-Mass models, revised low-mass models, and the ph-FRUITY interface. Astrophys. J. Suppl. Ser. 219, 40. doi:10.1088/0067-0049/219/2/40
Czyzak, S. J., Aller, L. H., and Kaler, J. H. (1968). Spectrophotometric studies of gaseous nebulae. XI. The planetary NGC 6543. Astrophys. J. 154, 543–555. doi:10.1086/149780
Delgado-Inglada, G., Morisset, C., and Stasinska, G. (2014). Ionization correction factors for planetary nebulae I. Using optical spectra. Mon. Not. R. Astron. Soc. 440, 536–554. doi:10.1093/mnras/stu341
Dinerstein, H. L. (1995). “Infrared emission lines as probes of gaseous nebulae,” in The analysis of emission lines. Editors R. Williams, and M. Livio (Cambridge University Press), 134. Available at:http://ned.ipac.caltech.edu/level5/March01/Dinerstein2/frames.html.
Dinerstein, H. L. (1980). Infrared line measurements and the abundance of sulfur in planetary nebulae. Astrophys. J. 237, 486–490. doi:10.1086/157892
Dinerstein, H. L., Kaplan, K. F., and Jaffe, D. T. (2015). On the complexity of H2 excitation near hot stars: High spectral and spatial resolution observations of compact planetary nebulae with IGRINS. IAU General Assem. Meet. 29, 2255756.
Dinerstein, H. L., Lester, D. F., Carr, J. S., and Harvey, P. M. (1988). Detection of fluorescent molecular hydrogen emission in the planetary nebula Hubble 12. Astrophys. J. 327, L27–L30. doi:10.1086/185133
Dinerstein, H. L., Lester, D. F., and Werner, M. W. (1985). Far-infrared line observations of planetary nebulae. I - the forbidden O III spectrum. Astrophys. J. 291, 561–570. doi:10.1086/163096
Dinerstein, H. L. (1991). Neutral matter in planetary nebulae. Publ. Astron. Soc. Pac. 103, 861–864. doi:10.1086/132895
Dinerstein, H. L. (2001). Neutron-Capture Elements in Planetary Nebulae: Identification of Two Near-Infrared Emission Lines as [Kr III] and [Se IV]. Astrophys. J. Lett. 550, L223–L226. doi:10.1086/319645
Dinerstein, H. L., Sterling, N., Kaplan, K., and Bautista, M. (2022). Enrichments of Te and Xe in planetary nebulae from new infrared emission lines observed with HPF on HET. Bull. Amer. Astr. Soc. 240, 351.06.
Dinerstein, H. L., Sterling, N., Kaplan, K., and Bautista, M. (2021). New infrared emission lines of the s-process enriched neutron-capture elements Br and Rb in planetary nebulae. Bull. Amer. Astr. Soc. 237, 548.13.
Ferland, G. J., Chatzikos, M., Guzmán, F., Lykins, M. L., van Hoof, P. A. M., Williams, R. J. R., et al. (2017). The 2017 release Cloudy. Rev. Mex. Astron. Y Astrof. 53, 385–438.
Garzón, F., Castro, N., Insausti, M., Manjavacas, E., Miluzio, M., Hammersley, P., et al. (2016). EMIR at the GTC: results on the commissioning at the telescope. Proc. SPIE, 9908, 99081J9. doi:10.1117/12.2232885
Geballe, T. R., Burton, M. G., and Isaacman, R. (1991). Observations of the 2-μm unidentified lines in planetary nebulae. Mon. Not. R. Astron. Soc. 253, 75–79. doi:10.1093/mnras/253.1.75
Glass, I. S. (2006). Revolutionaries of the cosmos: The astro-physicists. Oxford: Oxford University Press.
Hollenbach, D. J., and Tielens, A. G. G. M. (1997). Dense photodissociation regions (PDRs). Annu. Rev. Astron. Astrophys. 35, 179–215. doi:10.1146/annurev.astro.35.1.179
Huggins, W., and Miller, W. A. (1864). On the spectra of some of the nebulae. A supplement to the paper on the spectra of some of the fixed stars. Phil. Trans. Roy. Soc. Lond. 154, 437–444.
Kaplan, K. F., Dinerstein, H. L., and Jaffe, D. T. (2016). Resolving shocked and UV excited components of H2 emission in planetary nebulae with high-resolution near-infrared spectroscopy. Bull. Amer. Astr. Soc. 228, 219.06.
Käppeler, F., Gallino, R., Bisterzo, S., and Aoki, W. (2011). Thesprocess: Nuclear physics, stellar models, and observations. Rev. Mod. Phys. 83, 157–193. doi:10.1103/RevModPhys.83.157
Karakas, A. I., and Lattanzio, J. (2014). The Dawes review 2: Nucleosynthesis and stellar yields of low- and intermediate-mass single stars. Pub. Astr. Soc. Aust. 31, 62. doi:10.1017/pasa.2014.21
Karakas, A. I., and Lugaro, M. (2016). Stellar yields from metal-rich Asymptotic Giant Branch models. Astrophys. J. 825 (26), 26. doi:10.3847/0004-637X/825/1/26
Kewley, L. J., Dopita, M. A., Leitherer, C., Davé, R., Yuan, T., Allen, M., et al. (2013). Theoretical evolution of optical strong lines across cosmic time. Astrophys. J. 774, 17. doi:10.10880004-637S774/2/100
Kwitter, K. B., and Henry, R. B. C. (2022). Planetary nebulae: Sources of enlightenment. Publ. Astron. Soc. Pac. 134, 022001. doi:10.1088/1538-3873/ac32b1
Lambert, D. L., Smith, V. V., Busso, M., Gallino, R., and Straniero, O. (1995). The chemical composition of red giants. IV. The neutron density at the s-process site. Astrophys. J. 450, 302. doi:10.1086/176141
Lester, D. F., Hill, G. J., Doppman, G., and Froning, C. S. (2000). CoolSpec: A near-infrared long-slit spectrometer for McDonald observatory. Pub. Astr. Soc. Pac. 112, 384–396. doi:10.1086/316542
Likkel, L., Dinerstein, H. L., Lester, D. F., Kindt, A., and Bartig, K. (2006). A spectrophotometric survey of K-band emission lines in planetary nebulae. Astron. J. 131, 1515–1529. doi:10.1086/500050
Luhman, K. L., and Rieke, G. H. (1996). High-resolution near-infrared spectroscopy of Hubble 12. Astrophys. J. 461, 298–306. doi:10.1086/177056
Madonna, S., Bautista, M., Dinerstein, H. L., Sterling, N. C., García-Rojas, J., Kaplan, K. F., et al. (2018). Neutron-capture Elements in Planetary Nebulae: First Detections of Near-infrared [Te III] and [Br V] Emission Lines. Astrophys. J. Lett. 861, L8, 7. doi:10.3847/2041-8213/aaccef
Mahadevan, S., Ramsey, L. W., Terrien, R., Halverson, S., Roy, A., Hearty, F., et al. (2014). The habitable-zone planet finder: A status update on the development of a stabilized fiber-fed near-infrared spectrograph for the hobby-eberly telescope. Proc. SPIE, 10. doi:10.1117/12.2056417
Manea, C., Dinerstein, H. L., Sterling, N. C., and Zeimann, G. (2022). Chemical abundances of eight highly-extincted milky way planetary nebulae. in press.Astron. J. 164 (185, 10) doi:10.3847/1538-3881/ac8a45
Merrill, P. W. (1952). Spectroscopic observations of stars of class. Astrophys. J. 116, 21–26. doi:10.1086/145589
Moore, C. E. (1952). Atomic Energy Levels as Derived from the Analyses of Optical Spectra, II (24Cr – 41Nb). Washington, DC: National Bureau of Standards.
Park, C., Jaffe, D. T., Yuk, I.-S., Chun, M.-Y., Pak, S., Kim, K.-M., et al. (2014). Design and early performance of IGRINS (immersion grating infrared spectrometer). Proc. SPIE 9147, 12. doi:10.1117/12.2056431
Péquignot, D., and Baluteau, J.-P. (1997). “The discovery of heavy elements (Z > 30) in NGC 7027,” in IAU symp., 180. Editors H. J. Habing, and H. J. G. L. M. Lamers (Dordrecht: Kluwer), 128.
Péquignot, D., and Baluteau, J.-P. (1994). The identification of krypton, xenon, and elements of rows 4, 5, and 6 of the periodic table in the planetary nebula NGC 7027. Astron. Astrophys. 283, 593–625.
Péquignot, D. (1997). “Deep optical spectroscopy and modelling of Planetary Nebulae,” in Planetary nebulae: IAU symp. 180. Editors H. J. Habing, and H. J. G. L. M. Lamers (Dordrecht: Kluwer), 167.
Ramsay, S. K., Chrysostomu, A., Geballe, T. R., Brand, P. W. J. L., and Mountain, M. (1993). Pure fluorescent H2 emission from Hubble 12. Mon. Not. R. Astron. Soc. 263, 695–700. doi:10.1093/mnras/263.3.695
Rayner, J., Bond, T., Bonnet, M., Jaffe, D., Muller, G., and Tokunaga, A. (2012). iSHELL: a 1-5 micron cross-dispersed R=70, 000 immersion grating spectrograph for IRTF. Proc. SPIE, 844612. doi:10.1117/12.925511
Schöning, T. (1997). Effective collision strengths for transitions in the 4pk(k = 2-4) ground configurations of Kr III, Kr IV, and Kr V. Astron. Astrophys. Suppl. Ser. 122, 277–283. doi:10.1051/aas:1997133
Sneden, C., Cowan, J. J., Lawler, J. E., Ivans, I., Burles, S., Beers, T. C., et al. (2003). The extremely metal-poor, neutron capture-rich star CS 22892-052: A comprehensive abundance analysis. Astrophys. J. 591, 936–953. doi:10.1086/375491
Sterling, N. C., Dinerstein, H. L., Kaplan, K. F., and Bautista, M. A. (2016). Discovery of rubidium, cadmium, and germanium emission lines in the near-infrared spectra of planetary nebulae. Astrophys. J. 819, L9. doi:10.3847/2041-8205/819/1/l9
Sterling, N. C., and Dinerstein, H. L. (2008). The abundances of light neutron-capture elements in planetary nebulae. II. s-Process enrichments and interpretation. Astrophys. J. Suppl. Ser. 174, 158–201. doi:10.1086/520845
Sterling, N. C. (2020). Neutron-capture element abundances in planetary nebulae. Galaxies 8, 50–59. doi:10.3390/galaxies8020050
Sterling, N. C., Porter, R. L., and Dinerstein, H. L. (2015). The abundances of light neutron-capture elements in planetary nebulae. III. The impact of new atomic data on nebular selenium and krypton abundance determinations. Astrophys. J. Suppl. Ser. 218, 25. doi:10.1088/0067-0049/218/2/25
Sternberg, A., and Dalgarno, A. (1989). The infrared response of molecular hydrogen gas to ultraviolet radiation: High-density regions. Astrophys. J. 338, 197–233. doi:10.1086/167193
Treffers, R. R., Fink, U., Larson, H. P., and Gautier, T. N. (1976). The spectrum of the planetary nebula NGC 7027 from 0.9 to 2.7 microns. Astrophys. J. 209, 793–799. doi:10.1086/154777
Yagüe López, A., García-Hernández, D. A., Ventura, P., Doherty, C. L., den Hartogh, J. W., Jones, S. W., et al. (2022). First models of the s process in AGB stars of solar metallicity for the stellar evolutionary code ATON with a novel stable explicit numerical solver. Astron. Astrophys. 657, A28. doi:10.1051/0004-6361/202039318
Keywords: planetary nebulae, chemical abundances, nucleosynthesis, stellar evolution, s-process, Asymptotic Giant Branch (AGB) stars, infrared spectroscopy
Citation: Dinerstein HL (2022) Observing the signatures of AGB s-process nucleosynthesis in planetary nebulae: An origins story. Front. Astron. Space Sci. 9:1063995. doi: 10.3389/fspas.2022.1063995
Received: 07 October 2022; Accepted: 14 November 2022;
Published: 02 December 2022.
Edited by:
Karen B Kwitter, Williams College, United StatesReviewed by:
Jorge García-Rojas, Instituto de Astrofísica de Canarias, SpainCopyright © 2022 Dinerstein. This is an open-access article distributed under the terms of the Creative Commons Attribution License (CC BY). The use, distribution or reproduction in other forums is permitted, provided the original author(s) and the copyright owner(s) are credited and that the original publication in this journal is cited, in accordance with accepted academic practice. No use, distribution or reproduction is permitted which does not comply with these terms.
*Correspondence: Harriet L. Dinerstein, aGFycmlldEBhc3Ryby5hcy51dGV4YXMuZWR1
†ORCID: Harriet L. Dinerstein, orcid.org/0000-0002-4017-5572
Disclaimer: All claims expressed in this article are solely those of the authors and do not necessarily represent those of their affiliated organizations, or those of the publisher, the editors and the reviewers. Any product that may be evaluated in this article or claim that may be made by its manufacturer is not guaranteed or endorsed by the publisher.
Research integrity at Frontiers
Learn more about the work of our research integrity team to safeguard the quality of each article we publish.