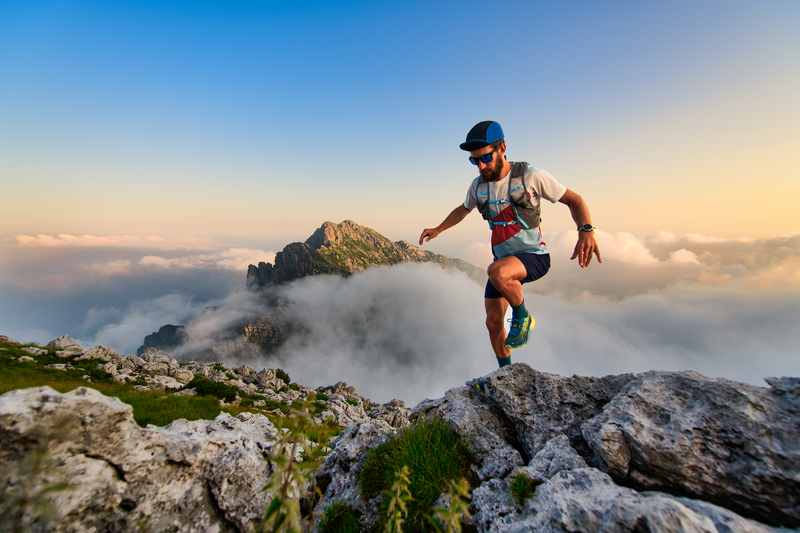
95% of researchers rate our articles as excellent or good
Learn more about the work of our research integrity team to safeguard the quality of each article we publish.
Find out more
REVIEW article
Front. Astron. Space Sci. , 30 January 2023
Sec. Space Physics
Volume 9 - 2022 | https://doi.org/10.3389/fspas.2022.1063190
This article is part of the Research Topic The Future of Space Physics 2022 View all 66 articles
The lower thermosphere-ionosphere (LTI) is a key transition region between Earth’s atmosphere and space. Interactions between ions and neutrals maximize within the LTI and in particular at altitudes from 100 to 200 km, which is the least visited region of the near-Earth environment. The lack of in situ co-temporal and co-spatial measurements of all relevant parameters and their elusiveness to most remote-sensing methods means that the complex interactions between its neutral and charged constituents remain poorly characterized to this date. This lack of measurements, together with the ambiguity in the quantification of key processes in the 100–200 km altitude range affect current modeling efforts to expand atmospheric models upward to include the LTI and limit current space weather prediction capabilities. We present focused questions in the LTI that are related to the complex interactions between its neutral and charged constituents. These questions concern core physical processes that govern the energetics, dynamics, and chemistry of the LTI and need to be addressed as fundamental and long-standing questions in this critically unexplored boundary region. We also outline the range of in situ measurements that are needed to unambiguously quantify key LTI processes within this region, and present elements of an in situ concept based on past proposed mission concepts.
The Lower Thermosphere and Ionosphere (LTI) has accurately been described as the transition layer between atmosphere and space. Within this layer, the neutral gases of the upper layers of the atmosphere coexist and interact with the ionized gases of the lower edge of the plasma-dominated space. In particular, interactions between ions and neutrals maximize at altitudes between 100 and 200 km: it is within this altitude range that electrical conductivities, electrical currents, ion-neutral collisions and the resulting frictional heating maximize (Richmond (2017); Strangeway (2012)); it is also within this region that the auroral peak emission height is found and auroral structures maximize (Partamies et al., 2022). At the same time, energetic particle precipitation passing through this region has profound effects on chemistry at altitudes below and significantly influences conductivity within this region.
Whereas the underlying principles of the physical processes related to ion-neutral interactions is well known theoretically, their quantification is still largely unknown. The reason is that processes related to ion-neutral interactions involve and require the knowledge of a large number of parameters, which include ion, electron and neutral temperatures (Ti, Te and Tn), ion, neutral and electron densities (Ni, Nn, Ne), ion and neutral composition (nix and nnx), ion drifts vi), neutral winds (un), electric field
However, these measurements have never been simultaneously available, as the altitude range from 100 to 200 km remains to this date the least visited region in the near-Earth environment (Sarris (2019); Palmroth et al. (2021)). With the exception of the Atmosphere Explorer satellites (1973–1981), which sampled periodically this region along elliptical orbits achieving a lowermost altitude of 128 km by use of propulsion, and also with the exception of short-lived sounding rocket flights, these altitudes have remained scarcely sampled in situ, primarily due to the enhanced atmospheric drag in this region. Whereas a wealth of information is currently provided by remote sensing measurements, either from space or from the ground, obtaining all the above parameters simultaneously can only be done with in situ measurements by a spacecraft equipped with a comprehensive instrument suite. This lack of co-located measurements of all involved parameters leads to great discrepancies between estimations of, for example, conductivities, electrical currents and frictional heating, which are critical parameters for the accurate description of the upper atmosphere.
In pursuit of the overall community goal to understand how geospace works within the larger heliophysical system, it is important to understand how the LTI acts as the dynamical interface between the atmosphere and space. The two-way interactions between the atmosphere and the LTI as well as those between the magnetosphere and the LTI that constitute this interface are strongly controlled by the variable conditions within LTI and its internal physical processes. Central to this multi-faceted interface are the features of the LTI system associated with direct interactions between the plasma and neutral gas. Thus the overarching goal and main theme of the mission discussed here is to: Understand the role of charged-neutral collisions in the LTI in its larger role as a physical mediator between the atmosphere and space.
The approach to making progress on this goal first divides the task into three broad areas: Energetics, Dynamics, and Chemistry. Key uncertainties in these areas are then used to develop focused science questions which, if answered, will provide significant progress toward this goal. Focused science questions under each area that will need to be answered in the coming decade include the following:
Q1. Energetics.
How and to what extent is energy deposited as frictional or Joule heating in the LTI? How does this heating affect, and is affected by, the thermal structure, local transport, and composition within LTI altitudes? How does the magnitude, extent and evolution of this heating affect neutral density and satellite drag?
Q2. Dynamics.
What is the momentum budget in the LTI and the relative contributions of magnetospheric, solar and atmospheric forcing? What are the consequences of these forcings on fluid dynamics and electrodynamics at high, middle and low latitudes? How do collisions between charged and neutral gases in the LTI affect the density, composition, winds and drifts in the region?
Q3. Chemistry.
What are the effects of Energetic Particle Precipitation (EPP) on the ionization and composition of the LTI? To what extent does EPP impact the mesosphere and stratosphere?
Addressing the above questions will fundamentally advance our understanding of the atmosphere-space transition region and of the neutral-plasma interactions that shape it; at the same time, addressing the above science questions will also assist in addressing topics related to critical societal and operational needs, as discussed below.
Together with the need to fundamentally advance our understanding of the physics of the atmosphere-space transition region, as documented, e.g., by Palmroth et al. (2021) and Karlsson et al. (2020), the urgency to significantly improve the climatological specification and empirical models of the LTI by incorporating new LTI datasets has been described by, e.g., Emmert et al. (2021); furthermore, the importance of enhancing the predictive capability of the LTI for operational needs and applications by better knowledge of the LTI has been highlighted by Crisp et al. (2020). A key reason is that the LTI is the epicentre of multiple activities of modern, technology-based civilization, and addressing the above science questions is linked to addressing a number of societal and operational space weather needs. In particular, knowledge of the neutral density and its variability are critical to predicting orbit trajectories of low Earth orbiting satellites: in view of the recent transition of space usage from GEO (which dominated public usage of space until recently) to LEO (where New Space mega-constellations are operating), accurate predictions of atmospheric drag in relation to geomagnetic activity is an essential parameter in spacecraft operations. The recent loss of 40 Starlink satellites near 210 km during a moderate geomagnetic storm (Hapgood et al. (2022)), which occurred due to enhanced Joule heating in the LTI, shows that such predictions are still lacking. Furthermore, the induced currents in power grids associated with high latitude ionospheric currents driven by storms is another space weather application where detailed knowledge of the current systems and of conductivity will advance our understanding of how sudden current surges affect power infrastructure when large scale magnetic storms are present. Finally, localised electron density increases in the LTI and above, associated with particle precipitation or other sources of density irregularities, are known to affect radio wave propagation. This leads to uncertainties in navigation in the form of scintillation of Global Navigation Satellite System (GNSS) signals, as well as distortion of telecommunication signals (Xiong et al. (2016)). The development of mitigation techniques for radio propagation perturbations require new insight on LTI processes that lead to the increase in ionisation, in particular during active geomagnetic conditions.
In the following, focused science questions in the LTI are further described under each of the three broad areas in terms of energetics, dynamics, and chemistry/energetic particle precipitation effects. The need for in situ measurements to address these questions and elements of a notional “way forward” to address these questions are further elaborated in the Discussions section.
This paper evolved from a white paper (Sarris et al., 2022b) that was submitted in response to the call for white papers for the Decadal Survey for Solar and Space Physics 2024–2033, which aims to present a prioritized strategy of basic and applied research to advance scientific understanding in the field of Heliophysics.
The largest uncertainty in the energetics of the LTI is the impact of frictional heating, or, equivalently, Joule heating (Strangeway, 2012). Frictional or Joule heating occurs within the LTI when the ionospheric plasma is forced by electromagnetic fields to convect relative to the neutral gas. This coupling is strongly related to solar activity: During geomagnetically active times, the closure of field aligned currents at high latitudes occurs within the ionospheric E and F regions where plasma-neutral collisional interactions are important. The resulting Joule heating subsequently modifies the neutral wind and density patterns globally (see Figure 1). While the heating rates maximize in the E region, the effects are significant also above in the F region where the relatively lower density atmosphere clearly reacts to these processes in geomagnetically active periods. This has the effect that spacecraft within this region will experience enhanced atmospheric drag, which can lead to sudden loss of orbital altitude, decrease in orbital lifetime and potentially reentry (e.g., Fang et al. (2022)). Without detailed knowledge of Joule heating rate, its distribution in altitude, latitude and local time and its effects on the thermosphere, predicting drag effects and spacecraft orbits is extremely difficult. During even moderate geomagnetic storms, our present knowledge indicates, albeit with large uncertainties, that Joule heating is the largest heating source, larger than the heat created by absorption of EUV solar radiation, which otherwise is the major driver for upper atmospheric dynamics. At moderate to strong activity, the effects of electromagnetic coupling with space are not confined to high latitudes but will propagate to the equator.
FIGURE 1. The closure of field aligned currents at high latitudes via perpendicular currents within the altitudes where plasna-neutral interactions maximize leads to Joule heating that subsequently modifies the neutral wind and density patterns at lower latitudes. The vertical structure of Hall and Pedersen perpendicular currents are drawn in the upper left inset of this figure based on Global Circulation Model results. Adapted from ESA Daedalus RfA (2020).
The ways in which neutral winds, ion drifts and electric fields interact in this heating process, which maximizes in the LTI, are largely unknown, primarily due to the lack of co-located measurements of all key parameters involved. Current estimations are based on primarily ground-based measurements, which, however, only provide a subset of the required measurements that are needed to resolve Joule heating in the LTI (for example, they lack neutral wind measurements), with limited spatial resolution, with lower accuracy compared to in situ measurements and above geographically fixed locations. Key factors affecting Joule heating include the ionospheric Pedersen conductivity, σP, neutral winds, un, electric fields, E, and ion drifts, vi. In particular, σP is dependent on knowledge of precipitating electrons, whereas neutral winds are affected by Lorentz forcing, both of which are poorly characterized in the LTI. Accurate estimates of σP and neutral wind variations in the LTI are also needed for improved space weather forecasts (Mannucci et al., 2016), and for Joule heating statistics (Cai et al., 2016).
Comparisons of different models and estimation methodologies show great discrepancies in the amount of energy that is deposited via Joule heating in the LTI (e.g., Palmroth et al., 2021). A main reason for these discrepancies is that models can not currently resolve small scales, which are believed to contribute significantly to the total Joule heating, even though the distribution of this heating at various scales is not known. It is known, however, that auroral arcs, which are important conduits for energy input, are structured at scales on the order of 1 km and lower (Maggs and Davis (1968); Borovsky and Suszcynsky (1993)). Electric field structures can be significant on these and possibly even smaller scales, and the effect of these small-scale structures does not average to zero since Joule heating is proportional to E2. This energy is hence significantly under-estimated in physics-based general circulation models (GCMs), which lack the ability to resolve intense localised electric fields in the vicinity of auroral arcs (see, e.g., Deng and Ridley (2007); Aikio and Selkälä (2009)). In lack of an accurate characterization of Joule heating at small scales, GCMs commonly need to introduce a scaling or multiplication factor that arbitrarily adjusts Joule heating (e.g., Emery et al. (1999)) so as to reproduce realistic temperatures in GCMs that can be compared reasonably well against those of empirical models and observations. This insufficient Joule heating in GCMs or uncertainty of its altitude distribution (e.g., Huang et al. (2012)) has been attributed primarily to the contribution of small-scale variability or structuring of the electric field (e.g.; Codrescu et al. (1995)), the relative importance of which can only be obtained in situ: For example, previous studies based on DE-2 data (e.g., Matsuo et al. (2003); Matsuo and Richmond (2008)) have shown that this variability in the electric field can be comparable in magnitude or can even exceed the average.
Measurements of all parameters involved in the Joule heating equation need to be obtained simultaneously, at relevant scales where Joule heating is significant, down to 1 km or below. This includes measurements of neutral winds, ion drifts, electric field, magnetic field, ion and neutral composition, densities and temperatures, and energetic particle precipitation are needed. These are summarized in Table 1, separated in terms of ionosphere (or plasma) parameters, thermosphere (or neutral) parameters, and fields. In the right column of Table 1, instruments that are commonly used to obtain these parameters in situ are also listed. These measurements should be made by collecting statistically representative distributions that cover the pertinent range of auroral latitudes, local times and targeted altitudes, which lie in the 100–200 km region, and, ideally, down to the altitudes where plasma-neutral interactions and hence Joule heating maximize, which is estimated to be at ∼120 km. At the same time, the altitude profile of Joule heating and its contributing parameters should be resolved, statistically and with a combination of satellite and ground-based assets. These measurements can be used to anchor ground-based observations and test current assumptions and parameterizations of Joule heating as well as of Pedersen conductivity in models.
TABLE 1. List of primary products or geophysical observables needed to address the science questions listed herein and commonly used instruments for their derivation.
Forcing from the magnetosphere, from the atmosphere, and direct solar influences have profound impact on the LTI and how it evolves. These influences on the LTI are affected by the exchange of momentum between charged and neutral components, resulting in the transport of momentum vertically and horizontally. Dynamics in the LTI refer to the motion of plasma and neutral gases in relation to the forces that drive and affect them, and to the overall momentum budget in the LTI. The presence of an ionised component and the geomagnetic field within the LTI greatly affect the forces that drive the motion of both neutral and ion species. Electromagnetic coupling introduces a direct Lorentz force to the set of forces governing the dynamics of the LTI. Deviations between ion and electron
FIGURE 2. Ion and electron (E × B) drifts in the frame of the neutral gas as a function of altitude (i.e. neutral density and collision frequency), and resulting electric currents.
Currents flowing through the LTI are associated with plasma motions and co-located neutral gas motions via ion-neutral collisions. Furthermore, the LTI is the critical region where neutral dynamics, including tidal, planetary and gravity waves, drive the ionospheric dynamo and its variability in the E and bottom-F regions (Liu, 2016). Although these processes are understood theoretically, their quantification and characterization are largely absent due to a lack of measurements in the regions where magnetospheric forcing and collisions between charged and neutral gases maximize, which is mainly within the 100–200 km altitude range. In particular, comprehensive measurements of neutral winds are critical in advancing current knowledge of LTI dynamics and, presently, such measurements are sparsely distributed in time and space (Heelis and Maute (2020); Emmert et al. (2021)). It is noted in particular that no current or prior set of observations combines simultaneous and co-spatial measurements of horizontal1 and vertical neutral winds together with electric currents. In addition, estimates of collision rates are currently based on cross-sections from ground laboratory experiments, which, however, cannot reproduce the complex LTI environmental plasma, neutral and electromagnetic conditions (Yamauchi et al., 2022). At small scales, strong flow shears and steep gradients can drive a variety of instabilities and turbulence in both plasma and neutral gas, the understanding of which is an outstanding challenge in space physics community. Instabilities and turbulence, in turn, result in irregularities of various scales, which have practical implications for the propagation of waves. In particular, variations and gradients in the plasma density can strongly affect the propagation of trans-ionospheric radio waves on which our modern communication and navigation systems rely.
Sampling with high cadence the physical properties of the charged and neutral gas alongside electromagnetic fields within the 100–200 km altitude region, where collisions between charged and neutral particles maximize, would close the gaps in our understanding of the dynamics and its electrodynamic coupling on relevant scales. Co-temporal and co-spatial measurements of neutral winds, ion drifts, electric field, magnetic field, ion and neutral composition, densities and temperatures in the 100–200 km altitude range are needed, as summarized in Table 1.
It is noted that a single-satellite in situ mission can provide local measurements of the above properties, however resolving LTI electrodynamics, such as addressing the ionospheric dynamo problem, investigating the altitude distribution or 3D structure of currents, etc., is more challenging since it involves non-local processes. In particular, since the field lines are essentially equipotentials, flux tube integrated quantities are needed to fully explain the dynamics. In one approach, local measurements as provided by an in situ mission need to be combined with models to address this problem. Statistical ensembles of local measurements that extend up into the F-region can also be used to address the ionospheric dynamo problem. In another (optimal) approach, using twin-satellite measurements that are displaced in altitude enables obtaining altitudinal gradients and height- or flux-tube-integrated profiles of, e.g., electron density, Pedersen conductivity and Joule heating directly, rather than statistically. In a recent study, Vogt et al. (2022) investigated the optimal separation in altitude between two satellites for obtaining altitude profiles, via implementing extrapolation horizons, also involving models; this approach demonstrates the pathway towards vastly improving current estimates of height-integrated quantities.
A significant amount of the chemistry of the LTI and also the middle atmosphere below is controlled by energetic particle precipitation (EPP). This chemistry in turn strongly affects the balance of heating and cooling in and near the LTI, influencing not only the energetics discussed above, but the production and transport of these potent species, that changes the character of the LTI’s plasma-neutral interactions.
EPP consists of energetic (keV-MeV) electrons and ions that follow the Earth’s magnetic field towards the atmosphere (Figure 3). EPP leads to the excitation and ionization of neutrals, dissociation of molecular species, and electron temperature enhancements, all of which affect conductivity. EPP fluxes between 100 and 200 km and their contributions to key physical processes at these altitudes and below are largely unknown.
FIGURE 3. Left: Northern hemisphere diffuse auroral electron energy flux (mW/m2) based on Ovation Prime. Right: Simulation of particle gyro-motion for different pitch angles; Adapted from Sarris et al. (2020).
The altitude of maximum energy deposition by EPP is determined by the energies of the precipitating particles. Knowledge of the energy spectrum as a function of solar and geomagnetic conditions, magnetic latitude and local time is therefore key to understanding the role of EPP within the LTI and below. Investigation of coupled-climate model runs and atmospheric reanalysis datasets have further shown that, as higher energy
Energetic Particle Precipitation (EPP) impacts are currently implemented in chemistry-climate models reaching from the surface up to the mesosphere or lower thermosphere, however, there are still many open questions not only in the quantification but also in the theoretical description of the impacts of EPP. The most important issue concerns the uncertainties associated with the production rate of the different NOx species by EPP, and the complex coupling between chemical changes, atmospheric heating and cooling rates, and atmospheric dynamics. Improved EPP measurements will lead to the improvement of the EPP specification in models.
It is noted in particular that the recent solar forcing recommendation for the Coupled Model Intercomparison Project Phase 6 (CMIP6) includes EPP for the first time (Matthes et al., 2017), indicating a wider recognition of the potential importance of EPP for the middle and lower atmosphere. However, currently, CMIP6 forcing comes from a proxy model that is based on MEPED/POES satellite observations (Van de Kamp et al. (2016), which only provide three energy channels between 30 and 1,000 keV, and only measure a part of the precipitation entering the atmosphere Rodger et al. (2010). Due to the inadequate energy resolution and measurement geometry, it is believed that current measurements do not capture the precipitation fully, and a recent re-analysis of MEPED/POES data by Nesse Tyssøy et al. (2016) has shown that fluxes could be under-estimated or overestimated by a significant factor, depending on pitch angle and reaching close to an order of magnitude for lower flux levels. As a demonstration of the potential impacts of an improved EPP specification, in support of the Daedalus mission science case definition (ESA Daedalus RfA, 2020), a variation of EPP forcing was used in a climate mode run compared to the current CMIP6 recommendation, and impacts on HOx, NOx and O3 were simulated. The variation in EPP was based on the range of potential under-estimations or over-estimations of the current specification. The differences in middle atmosphere impacts between the two forcing datasets, via changes in atmospheric ionisation rates, have then been simulated with 1-year chemistry-climate model experiments with specified dynamics (WACCM-D, Verronen et al. (2016)). Averaged changes, shown in Figure 4 (adapted from Figure 51 of ESA Daedalus RfA (2020)), suggest that any substantially different fluxes that an in situ mission might detect would translate into substantial differences in simulated middle atmosphere chemistry. In this figure we can see that ozone depletion due to additional HOx maximises in the mesosphere in the southern auroral oval; stratospheric depletion is due to NOx and maximises during austral winter/spring. Crude extrapolations of the ozone changes onto stratospheric temperatures, dynamics and regional surface climate impacts, by scaling results from e.g. Rozanov et al. (2005), Rozanov et al. (2012), could imply significant polar regional surface temperature signals. These need to be known when assessing long-term warming trends due to global climate change, to account for solar-driven natural variability and regional biases in simulations.
FIGURE 4. Middle atmospheric impact from using Daedalus-based EPP forcing, based on WACCM chemistry-climate simulations over (maximum EPP) year 2003. (A) Mean SH odd hydrogen (HOx) increase in June-August at 65–75 km altitude, relative to simulations using the CMIP6 EPP forcing; (B) Same as (A), but for O3 decrease; (C) Monthly mean odd nitrogen (NOx) increase in the SH polar cap, relative to simulations using the CMIP6 EPP forcing; (D) Same as (C), but for O3 decrease. For reference, the NOx mixing ratio background peaks in the uppermost mesosphere and lowermost thermosphere (near 90 km and above), O3 around 30–40 km. Figure by M. Szelag, P. Verronen; adapted from ESA Daedalus RfA (2020).
EPP observations with a better geometry and improved pitch angle and energy resolution are essential when addressing the question of EPP-climate connection. Satellite-based observations of electron fluxes with high temporal, energy and pitch angle resolution within the 100–200 km altitude range are needed, together with coincident electric and magnetic field data, so as to determine their correlation with EPP for its accurate parameterization in models.
The science questions that are presented herein in terms of energetics, dynamics and effects of precipitating particles are intricately linked, and addressing all relevant processes requires a methodological approach that utilizes comprehensive instrumentation to measure all parameters involved. For example, Joule heating maximizes per unit volume at low altitudes from 100 to 200 km where the Pedersen conductivity is highest; heating in this region also occurs due to energy deposited by impacting particle precipitation, which, similarly to Joule heating, is enhanced during active times. Particle precipitation is also directly linked to enhanced Pedersen conductivity. Field aligned currents close in this region through fundamental processes that occur in high latitudes: these processes concern perpendicular (horizontal) currents, which are of critical importance for dissipation and heating in the upper atmosphere, and feedback mechanisms, which help to regulate magnetosphere-ionosphere coupling. Furthermore, in this region originate large-scale neutral wind circulation changes, but also smaller-scale intense neutral wind “jet streams,” which have been associated with auroral formations. These “jet streams” peak at 150 km (Brinkman et al., 1995), and close to the region where the ion-drag force per unit mass peaks. Quantifying such large-scale and small-scale neutral winds, and determining their circulation patterns and relevant intensities, are critical in determining the dynamics and the overall momentum budget in the LTI. At the same time, the neutral winds are known to significantly contribute to and influence the resulting Joule heating rates, demonstrating a linkage between energetics and dynamics in the LTI. In addition, the relative motion of ions (which are slowed down by neutrals), electrons (which undergo an ExB drift in this region), and neutrals (whose dependencies and intensities are among the largest unknowns in the LTI) are all inter-connected in complex neutral-plasma interactions and neutral as well as plasma circulation patterns. Resolving the interplay of the above processes requires simultaneous measurements of all relevant parameters.
In the following, we first summarize key reasons that highlight the need for in situ measurements to address the above science questions. We then provide an overview of the status of in situ measurements in the LTI. We proceed with presenting the notional concept for a space mission targeting to sample the LTI below 200 km with in situ measurements. Specific advantages of multi-point measurements and synergistic activities with ground-based instruments are also presented. This section is concluded by a summary of the notional way forward.
Whereas ground-based remote sensing facilities have been providing continuous measurements of key LTI parameters as a function of altitude and local time, and are currently a main source of information on ion-neutral interactions across the LTI (see, e.g., Wahlund et al. (1992); Rishbeth and Van Eyken (1993); and, as examples of recent studies, e.g. the work by Tesema et al. (2022); Günzkofer et al. (2022)), there are limitations of remote sensing methods in addressing the science questions outlined herein. These limitations are related to the temporal and spatial resolution that ground-based instruments can provide as well as to the need for co-temporal, co-spatial measurements of all parameters involved. In contrast, in situ measurements offer a number of specific advantages and unique capabilities, as outlined in the following.
In terms of spatial and temporal scales, in situ measurements offer vastly better resolution, enabling to resolve the contribution of small-scale variability to the total Joule heating, which is a major unknown in the LTI. For example, electric fields down to 1 km or smaller are known to be significant at times, with variability at these scales often exceeding the average. High-cadence in situ measurements also enable detecting field-aligned currents at small scales (10 km and less) through measurements of magnetic perturbations, δB. Further to the spatial resolution, in situ measurements offer the ability to resolve E and δB fields on time scales of 10 s and faster, which are in the realm of energy transfer via Alfvén waves (e.g., Lotko and Zhang (2018)).
In terms of the completeness of the datasets, to accurately and unambiguously determine Joule heating in the LTI, according to Strangeway (2012) (eq. 38), simultaneous and co-located measurements of the electron number density, Ne, ion and electron velocities perpendicular to the geomagnetic field,
The distribution and magnitude of neutral winds is another missing piece in accurately estimating the energy budget and determining the dynamics in the LTI: for example, Joule heating has a neutral wind contribution which can be either positive or negative (e.g., Lu et al. (1995)), and which can be significant, even though this contribution is often ignored, due to lack of co-located measurements. As some rare co-located in situ rocket and trail-gas measurements indicate (e.g., Sangalli et al. (2009)), the effects of the neutral winds can be significant but can only be evaluated by co-temporal, co-spatial measurements of all the relevant parameters, which cannot be provided by ground-based remote sensing measurements. The ability to make neutral density and composition measurements co-located with plasma particle and field measurements in the LTI is also key, as these are necessary for direct estimation of conductivities; it is noted that conductivities are critically important for ongoing efforts to couple ionosphere and magnetosphere models (Ridley et al., 2004), and are currently estimated only from the ground through the combined use of measurements and models, which have significant uncertainties. Furthermore, an in situ platform allows to examine neutral density structuring and compositional changes and, importantly, to determine the causes of these variations, which cannot be determined remotely. Another example highlighting the importance of comprehensive in situ observations is the direct comparison of Joule heating with Poynting flux energy input at the same location, while examining how the heating effects the ambient neutral density, winds, and composition locally. Finally, the ability to resolve ion composition, including species N+, O+,
In summary, the required parameters include the vector ion motions and
Such direct measurements in the LTI can only be performed by an in situ mission of a fully instrumented spacecraft, which flies at optimal orbital configurations that permit detailed, in-depth analyses of the physical processes both via event studies as well as via statistical studies of the behavior of the LTI region as a function of local time, latitude, season, and magnetic activity. The ability of an in situ mission to statistically represent global processes and the global state of the LTI as a function of geomagnetic latitude, local time and activity levels by measurements performed over the mission’s lifetime has been demonstrated in ESA Daedalus RfA (2020) for the case of the Daedalus mission concept by performing mission performance demonstration simulations using Daedalus MASE (Mission Assessment through Simulation Exercise), an open-source package of scientific analysis tools aimed at research in the LTI (Sarris et al., 2022a).
The only systematic in situ spacecraft observations within the 100–200 km region to date were made by the Atmosphere Explorer AE-C, AE-D, and AE-E satellites (1973–1981), which measured density, ion drifts, neutral and ion composition and temperatures along elliptical orbits, achieving measurements down to a lowermost altitude of 128 km by use of propulsion. However, as shown in Figure 5, the total observation time from the three missions combined is only ∼60.3 h below 200 km, with a much smaller subset of this time allocated to high latitudes, the theater of key LTI processes. At the same time, AE spacecraft lacked neutral wind, electrodynamics and energetic particle measurements, and the interpretation of some critical measurements, such as mass spectrometer composition data, proved difficult at low altitudes. The Dynamics Explorer DE-2 satellite (1981–1983) included also measurements of energetic particles, electric field and neutral winds; however DE-2 did not include propulsion and the lowermost altitudes sampled were above 250 km. In the absence of other systematic co-temporal and co-spatial measurements, sounding rockets have proved to be an invaluable mean to obtain in situ measurements in the LTI, however, by nature, they are limited by providing only a snapshot of an altitude profile and most often carry limited instrumentation.
FIGURE 5. Left: Total observation time from AE-C, AE-D and AE-E combined, as a function of altitude and magnetic latitude. Total observation time at altitudes below 200 km is 60.3 h (Unified Abstract data). Right: Targeted total observation time from a 3-year in situ mission dedicated to studying LTI processes: ∼2000 h at altitudes
Since the time of the Atmosphere Explorers, there have been a number of matured mission concepts highlighting the need to sample plasma-neutral interactions at altitudes below 200 km with a fully instrumented satellite. These include: (a) the Thermosphere-Ionosphere-Mesosphere Energetics and Dynamics (TIMED) mission concept as originally proposed, and in particular the descoped in situ components of the twin-spacecraft mission, labeled TIMED-H and TIMED-L (NASA TIMED SDT, 1991); (b) the Geospace Electrodynamic Connections (GEC) mission concept (Sojka et al. (2001); Grebowsky and Gervin (2001)) (not to be confused with the Geospace Dynamics Constellation (GDC) mission concept (NASA GDC STDT, 2019), currently being selected for Phase A studies, which targets altitudes at 350 km or higher); (c) the Atmosphere-Space Transition Region Explorer (ASTRE) mission concept (Pfaff, 2022); (d) the Daedalus mission concept (Sarris et al. (2020); ESA Daedalus RfA (2020)). These mission concepts promise to offer an order-of-magnitude or more measurements than are currently available: as shown in Figure 5, whereas the total observation time at altitudes below 200 km is only 60.3 h from all past in situ measurements combined, the targeted total observation time from a 3-year in situ mission dedicated to studying LTI processes is ∼2000 h at altitudes
For sampling altitudes below the nominal perigee, all the above mission concepts make use of propulsion to episodically lower perigee, a maneuver that has been called a “deep-dip” or “deep-dive.” The lowermost altitudes targeted by the above concepts are all below 200 km: TIMED-H, TIMED-L and Daedalus target measurements down to 120 km, GEC targets 130 km “and possibly lower,” and ASTRE aims to perform measurements “below 200 km.” With respect to the lowermost altitudes to be sampled, it is noted that, whereas the entire LTI range from 100 to 200 km is of interest to LTI ion-neutral interaction processes, the lowermost altitudes targeted by the missions listed above are as low as 120 km. This is based on trade-offs between science return, such as capturing the peak of Joule heating and Pedersen conductivity, which is assumed to be at 120 km (see, e.g., Figure 16 of ESA Daedalus RfA (2020)), versus risks and mission performance related to the enhanced densities at the lowermost altitudes to be sampled. A proposed approach is further discussed below in Section 5.6.
Another striking similarity across the above mission concepts, highlighting the optimal path towards conclusively resolving processes related to plasma-neutral interactions in the 100–200 km transition region, is the need for comprehensive measurements of all parameters involved; thus, the instrument suites of all missions include in situ measurements of neutral winds, ion drifts, electric-magnetic fields, ion-neutral composition, densities and temperatures, and EPP.
Several of the above mission concepts have highlighted the substantial additional benefits of multi-point measurements: beyond significantly enhancing coverage, a second spacecraft can bring about estimation of gradients, shears and altitude profiles, or space-time effect disambiguation. For example, it has been demonstrated how simultaneous measurements at two altitudes by identically instrumented satellites enable determining altitudinal gradients or profiles of electron density, Pedersen conductivity and Joule heating directly, rather than statistically (Vogt et al., 2022). A concept has been proposed where nano-satellites in the form of CubeSats are deployed from a mothership (Sarris et al., 2020); these can be used as reference points or “buoys” for the estimation of local gradients of key properties while the mothership dips down to lower altitudes. Other concepts such as GEC emphasize the need for multi-point measurements in a “pearls-on-a-string” and “petal” configuration, addressing, respectively, spatial/temporal scales and exploring spatial extent of processes (Sojka et al., 2001). It is noted that the mission concepts involving multi-spacecraft observations emphasize the possibility of modifying the orbital configurations at different phases of the mission, to address different aspects of spatial and temporal characteristics of LTI processes.
An in situ mission, such as described above, can uniquely be paired with ground-based measurements, such as from Incoherent Scatter Radars and SuperDARN. Networks of such ground-measurements can provide the large to regional picture of the LTI as well as context measurements for an in situ mission concept. In addition, investigations by a mission with in situ probes that are carried out in conjunction with radars and other ground based instruments offer a unique telescope-microscope view across multiple scales, enhancing the return of an in situ mission. At higher altitudes, this approach has been demonstrated in a number of investigations, including the work by Aikio et al. (2018), who used Swarm satellite and EISCAT radar observations of electrodynamical parameters to reveal details of a plasma flow channel, and Ogawa et al. (2008), who used Reimei satellite and EISCAT radar observations to study the relationship between ion upflows and suprathermal ions. In situ missions have also been used in conjunction with ground-based stations that are used as heaters; in this case, satellite overflights are used to provide in situ measurements of the response of the magnetosphere and ionosphere to the heating (e.g., Robinson et al. (2000); Pokhotelov et al. (2004)). We note in particular the capabilities enabled by the upcoming EISCAT_3D facility with volumetric measurement capabilities of electron density and ion velocity vector that is currently under development, which offers unique opportunities for highly complementary and synergistic measurement campaigns with an in situ mission such as outlined herein; details of EISCAT_3D and on its important role in supporting current and future spacecraft missions has been outlined in McCrea et al. (2015).
In addition to the above synergistic opportunities, in situ measurements within the fields of view of remote sensing measurements offer the unique opportunity to test different assumptions and methodologies used in the retrievals of ionospheric parameters. For example, Kavanagh et al. (2022) used data from three decades of operations of the EISCAT radar to study the difference between the tristatic method and the scanning monostatic method. They found that large variability exists in the relation between the two techniques, resulting in different levels of Joule heating, particularly during active times. An in situ mission with appropriate instrumentation would provide a unique opportunity to test such assumptions and retrieval techniques.
Based on heritage from the early AEs of the 1970’s and from the NASA and ESA studies listed above, an in situ mission to sample the lower thermosphere-ionosphere to perigee passes of at least 120 km is entirely feasible. Since the 1970’s, early generations of instruments covering all required parameters have been flown successfully within the LTI region (80–200 km) on sounding rockets (with nominal velocities of ∼1 km/s), however, comprehensive measurements of the region will require coverage from orbit. For some measurements, this demands a new generation of measurement techniques capable of coping with non-geophysical perturbations, such as shock-induced variations and sputtered ions from the spacecraft surfaces at the lowermost altitudes. Fortunately, well-established techniques exist to model and address such satellite-generated disturbances and can be studied to mitigate these concerns. Given that satellite drag and re-entry considerations become a concern below 120 km, and due to the tremendous scientific return promised from such an unprecedented low perigee mission, we strongly urge that a satellite be flown as soon as possible with instruments to measure all of the relevant parameters to altitudes as low as 120 km; measurements below 120 km can be carried out on an exploratory basis during an extended mission phase based on findings from initial low perigee excursions to 120 km. The mission promises to open a new world of understanding of how the lower thermosphere and ionosphere works as a system, addressing all of the major outstanding questions outlined above.
The focused questions presented here argue strongly for an in situ mission to sample the lower thermosphere—ionosphere in the coming decade, to address critical, yet unquantified processes related to plasma-neutral interactions in the transition region from the neutral atmosphere to plasma-dominated space. A satellite equipped with instruments to provide co-located measurements of ion drifts, neutral winds, electric and magnetic fields, ion and neutral density, composition and temperature, and energetic particle precipitation is anticipated to yield a wealth of crucially lacking information, which will support a broad range of advances in atmospheric and space sciences. The above measurements in the heart of the under-sampled atmosphere-space transition region are key toward future advances in modeling the lower/middle/upper atmosphere - ionosphere - magnetosphere as a multi-coupled system. These measurements will enable the accurate quantification of Joule heating, the specification of which is currently a major challenge in global circulation models. Accurate estimates of ionospheric conductivity will lead to improvements in magnetospheric models, as conductivity specification determines, among others, the closure of currents, the evolution of magnetospheric and ionospheric dynamics, the convection state, and the timing and strength of auroral substorms. Measurements within this region will lead to improvements of whole atmosphere models that include the LTI. Data assimilation using these new datasets will help improve numerical models. Better understanding and parameterizations will improve predictions of drag effects and spacecraft re-entry. In situ measurements of perpendicular electrical currents and magnetospheric forcing will provide insights on their relationship and will improve our understanding of the cause of Geomagnetically Induced Currents, a significant Space Weather threat. Measurements within this region will also assist in additional topics, not described above, such as in improving satellite-level gas-surface interaction modelling algorithms and assumptions, enhancing existing models of Earth’s global lithospheric magnetic fields at medium to small scales (e.g., Olsen, 2022), and investigating the interaction of particles with electromagnetic waves (e.g., Tourgaidis and Sarris, 2022).
An in situ mission based on a single spacecraft can achieve all of the above objectives. However, beyond significantly enhancing coverage, simultaneous measurements by a second spacecraft at a different altitude would enable the estimation of gradients, shear and altitude profiles; measurements by a second spacecraft in a “pearls-on-a-string” configuration would enable differentiating between temporal and spatial effects; and measurements by a second spacecraft on a “petal” configuration would enable exploring the latitudinal extent of processes.
This mission can be paired with longer-term ground-based remote-sensing measurements, such as with SuperDARN and EISCAT_3D, to provide context measurements and a telescope-microscope view across multiple scales; this mission can also be paired with upcoming missions such as GDC (NASA GDC STDT, 2019) that will sample the 300–400 km altitude range, significantly above the altitude ranges of interest targeted herein, and DYNAMIC (NASA, 2022), that will provide a more global perspective of the neutrals in the lower thermosphere.
TS coordinated the writting of the manuscript and wrote the first draft. All authors contributed to the drafting of the manuscript and the ideas presented herein. All authors contributed to manuscript revision and approved the submitted version.
The authors declare that the research was conducted in the absence of any commercial or financial relationships that could be construed as a potential conflict of interest.
All claims expressed in this article are solely those of the authors and do not necessarily represent those of their affiliated organizations, or those of the publisher, the editors and the reviewers. Any product that may be evaluated in this article, or claim that may be made by its manufacturer, is not guaranteed or endorsed by the publisher.
Aikio, A., and Selkälä, A. (2009). Statistical properties of joule heating rate, electric field and conductances at high latitudes. Ann. Geophys. Copernic. GmbH) 27, 2661–2673. doi:10.5194/angeo-27-2661-2009
Aikio, A., Vanhamäki, H., Workayehu, A., Virtanen, I., Kauristie, K., Juusola, L., et al. (2018). Swarm satellite and eiscat radar observations of a plasma flow channel in the auroral oval near magnetic midnight. J. Geophys. Res. Space Phys. 123, 5140–5158. doi:10.1029/2018ja025409
Borovsky, J. E., and Suszcynsky, D. M. (1993). Optical measurements of the fine structure of auroral arcs, 80. Washington DC: American Geophysical Union Geophysical Monograph Series, 25.
Brinkman, D., Walterscheid, R., Lyons, L., Kayser, D., Christensen, A., Sharber, J., et al. (1995). E region neutral winds in the postmidnight diffuse aurora during the atmospheric response in aurora 1 rocket campaign. J. Geophys. Res. Space Phys. 100, 17309–17320. doi:10.1029/94ja02814
Cai, L., Aikio, A., and Milan, S. (2016). Joule heating hot spot at high latitudes in the afternoon sector. J. Geophys. Res. Space Phys. 121, 7135–7152. doi:10.1002/2016JA022432
Codrescu, M., Fuller-Rowell, T., and Foster, J. (1995). On the importance of e-field variability for joule heating in the high-latitude thermosphere. Geophys. Res. Lett. 22, 2393–2396. doi:10.1029/95gl01909
Crisp, N. H., Roberts, P. C., Livadiotti, S., Oiko, V. T. A., Edmondson, S., Haigh, S., et al. (2020). The benefits of very low Earth orbit for Earth observation missions. Prog. Aerosp. Sci. 117, 100619. doi:10.1016/j.paerosci.2020.100619
Deng, Y., and Ridley, A. J. (2007). Possible reasons for underestimating joule heating in global models: E field variability, spatial resolution, and vertical velocity. J. Geophys. Res. Space Phys. 112. doi:10.1029/2006ja012006
Emery, B., Lathuillere, C., Richards, P. G., Roble, R., Buonsanto, M., Knipp, D., et al. (1999). Time dependent thermospheric neutral response tothe 2–11 November 1993 storm period. J. Atmos. solar-terrestrial Phys. 61, 329–350. doi:10.1016/s1364-6826(98)00137-0
Emmert, J. T., Drob, D. P., Picone, J. M., Siskind, D. E., Jones, M., Mlynczak, M., et al. (2021). NRLMSIS 2.0: A whole-atmosphere empirical model of temperature and neutral species densities. Earth Space Sci. 8, e2020EA001321. doi:10.1029/2020EA001321
ESA Daedalus RfA (2020). Earth explorer 10 candidate mission Daedalus report for assessment (RfA). Report for assessment, ESA Available at: https://esamultimedia.esa.int/docs/EarthObservation/EE10_Daedalus_Report-for-Assessment-v1.0_13Nov2020.pdf (Accessed December 27, 2022).
Fang, T.-W., Kubaryk, A., Goldstein, D., Li, Z., Fuller-Rowell, T., Millward, G., et al. (2022). Space weather environment during the spacex starlink satellite loss in february 2022. Space Weather. e2022SW003193.
Grebowsky, J., and Gervin, J. (2001). Geospace electrodynamic connections. Phys. Chem. Earth, Part C Sol. Terr. Planet. Sci. 26, 253–258. doi:10.1016/S1464-1917(00)00117-3
Günzkofer, F., Pokhotelov, D., Stober, G., Liu, H., Liu, H.-L., Mitchell, N. J., et al. (2022). Determining the origin of tidal oscillations in the ionospheric transition region with eiscat radar and global simulation data. J. Geophys. Res. Space Phys. 127, e2022JA030861. doi:10.1029/2022ja030861
Hapgood, M., Liu, H., and Lugaz, N. (2022). SpaceX—Sailing close to the space weather?. Space Weather 20, e2022SW003074. doi:10.1029/2022SW003074
Heelis, R., and Maute, A. (2020). Challenges to understanding the Earth’s ionosphere and thermosphere. J. Geophys. Res. Space Phys. 125, e2019JA027497. doi:10.1029/2019JA027497
Huang, Y., Richmond, A. D., Deng, Y., and Roble, R. (2012). Height distribution of joule heating and its influence on the thermosphere. J. Geophys. Res. Space Phys. 117, 17885. doi:10.1029/2012ja017885
Jakosky, B. M., Lin, R. P., Grebowsky, J. M., Luhmann, J. G., Mitchell, D., Beutelschies, G., et al. (2015). The Mars atmosphere and volatile evolution (maven) mission. Space Sci. Rev. 195, 3–48. doi:10.1007/s11214-015-0139-x
Karlsson, T., Andersson, L., Gillies, D., Lynch, K., Marghitu, O., Partamies, N., et al. (2020). Quiet, discrete auroral arcs—Observations. Space Sci. Rev. 216, 16–50. doi:10.1007/s11214-020-0641-7
Kavanagh, A. J., Ogawa, Y., and Woodfield, E. E. (2022). Two techniques for determining f-region ion velocities at meso-scales: Differences and impacts on joule heating. J. Geophys. Res. Space Phys. 127, e2021JA030062. doi:10.1029/2021ja030062
Killeen, T., Hays, P., Carignan, G., Heelis, R., Hanson, W., Spencer, N., et al. (1984). Ion-neutral coupling in the high-latitude F region: Evaluation of ion heating terms from dynamics explorer 2. J. Geophys. Res. Space Phys. 89, 7495–7508. doi:10.1029/ja089ia09p07495
Liu, H.-L. (2016). Variability and predictability of the space environment as related to lower atmosphere forcing. Space weather. 14, 634–658. doi:10.1002/2016SW001450
Lotko, W., and Zhang, B. (2018). Alfvénic heating in the cusp ionosphere-thermosphere. J. Geophys. Res. Space Phys. 123, 10–368. doi:10.1029/2018ja025990
Lu, G., Richmond, A., Emery, B., and Roble, R. (1995). Magnetosphere-ionosphere-thermosphere coupling: Effect of neutral winds on energy transfer and field-aligned current. J. Geophys. Res. Space Phys. 100, 19643. doi:10.1029/95ja00766
Maggs, J., and Davis, T. (1968). Measurements of the thicknesses of auroral structures. Planet. Space Sci. 16, 205–209. doi:10.1016/0032-0633(68)90069-x
Mannucci, A. J., Hagan, M. E., Vourlidas, A., Huang, C. Y., Verkhoglyadova, O. P., and Deng, Y. (2016). Scientific challenges in thermosphere-ionosphere forecasting–conclusions from the October 2014 nasa jpl community workshop. J. Space Weather Space Clim. 6, E01. doi:10.1051/swsc/2016030
Matsuo, T., and Richmond, A. D. (2008). Effects of high-latitude ionospheric electric field variability on global thermospheric joule heating and mechanical energy transfer rate. J. Geophys. Res. Space Phys. 113, 12993. doi:10.1029/2007ja012993
Matsuo, T., Richmond, A. D., and Hensel, K. (2003). High-latitude ionospheric electric field variability and electric potential derived from DE-2 plasma drift measurements: Dependence on imf and dipole tilt. J. Geophys. Res. Space Phys. 108, 1005. SIA–1. doi:10.1029/2002ja009429
Matthes, K., Funke, B., Andersson, M. E., Barnard, L., Beer, J., Charbonneau, P., et al. (2017). Solar forcing for cmip6 (v3. 2). Geosci. Model. Dev. 10, 2247–2302. doi:10.5194/gmd-10-2247-2017
McCrea, I., Aikio, A., Alfonsi, L., Belova, E., Buchert, S., Clilverd, M., et al. (2015). The science case for the EISCAT_3D radar. Prog. Earth Planet. Sci. 2, 21–63. doi:10.1186/s40645-015-0051-8
NASA, (2022). Dynamic homepage. Available at: https://soma.larc.nasa.gov/stp/dynamic/ (Accessed December 27, 2022).
NASA GDC STDT (2019). Science technology definition team for the geospace dynamics constellation. Final report, NASA. Available at: https://science.nasa.gov/science-pink/s3fs-public/atoms/files/GDCSTDTReportFINAL.pdf.
NASA TIMED SDT (1991). “The TIMED mission and science program, report of the science definition team,” in Executive summary NASA-CR-191377, 19930007494, NASA. Available at: https://ntrs.nasa.gov/api/citations/19930007494/downloads/19930007494.pdf (Accessed December 27, 2022).
Nesse Tyssøy, H., Sandanger, M. I., Ødegaard, L.-K., Stadsnes, J., Aasnes, A., and Zawedde, A. (2016). Energetic electron precipitation into the middle atmosphere—Constructing the loss cone fluxes from meped poes. J. Geophys. Res. Space Phys. 121, 5693–5707. doi:10.1002/2016ja022752
Ogawa, Y., Seki, K., Hirahara, M., Asamura, K., Sakanoi, T., Buchert, S., et al. (2008). Coordinated eiscat svalbard radar and reimei satellite observations of ion upflows and suprathermal ions. J. Geophys. Res. Space Phys. 113, 12791. doi:10.1029/2007ja012791
Olsen, N. (2022). Modelling Earth’s lithospheric magnetic field using satellites in low-perigee elliptical orbits. Geophys. J. Int. 232 (3), 2035–2048.
Palmroth, M., Grandin, M., Sarris, T., Doornbos, E., Tourgaidis, S., Aikio, A., et al. (2021). Lower-thermosphere–ionosphere (LTI) quantities: Current status of measuring techniques and models. Ann. Geophys. Copernic. GmbH) 39, 189–237. doi:10.5194/angeo-39-189-2021
Partamies, N., Whiter, D., Kauristie, K., and Massetti, S. (2022). Magnetic local time (MLT) dependence of auroral peak emission height and morphology. Ann. Geophys. 40, 605–618. doi:10.5194/angeo-40-605-2022
Pfaff, R. F. (2022). The atmosphere-space transition region explorer (ASTRE) - a low perigee satellite to investigate the coupling of the Earth’s upper atmosphere and magnetosphere, to be submitted to space science reviews.
Pokhotelov, D., Lotko, W., and Streltsov, A. V. (2004). Simulations of resonant alfvén waves generated by artificial HF heating of the auroral ionosphere. Ann. Geophys. Copernic. GmbH) 22, 2943–2949. doi:10.5194/angeo-22-2943-2004
Qian, L., Burns, A. G., Emery, B. A., Foster, B., Lu, G., Maute, A., et al. (2014). The NCAR TIE-GCM: A community model of the coupled thermosphere/ionosphere system. Model. ionosphere–thermosphere Syst., 73–83. doi:10.1002/9781118704417.ch7
Richmond, A. D. (2017). “Ionospheric electrodynamics,” in Handbook of atmospheric electrodynamics. Editor H. Volland (Boca Raton: CRC Press), Vol. II, 249–290.
Ridley, A., Gombosi, T. I., and DeZeeuw, D. (2004). Ionospheric control of the magnetosphere: Conductance. Ann. Geophys. Copernic. GmbH) 22, 567–584. doi:10.5194/angeo-22-567-2004
Rishbeth, H., and Van Eyken, A. (1993). EISCAT: Early history and the first ten years of operation. J. Atmos. Terr. Phys. 55, 525–542. doi:10.1016/0021-9169(93)90002-g
Robinson, T., Strangeway, R., Wright, D., Davies, J., Horne, R., Yeoman, T., et al. (2000). Fast observations of ulf waves injected into the magnetosphere by means of modulated RF heating of the auroral electrojet. Geophys. Res. Lett. 27, 3165–3168. doi:10.1029/2000gl011882
Rodger, C. J., Clilverd, M. A., Green, J. C., and Lam, M. M. (2010). Use of POES SEM-2 observations to examine radiation belt dynamics and energetic electron precipitation into the atmosphere. J. Geophys. Res. Space Phys. 115, 14023. doi:10.1029/2008ja014023
Rozanov, E., Calisto, M., Egorova, T., Peter, T., and Schmutz, W. (2012). Influence of the precipitating energetic particles on atmospheric chemistry and climate. Surv. Geophys. 33, 483–501. doi:10.1007/s10712-012-9192-0
Rozanov, E., Callis, L., Schlesinger, M., Yang, F., Andronova, N., and Zubov, V. (2005). Atmospheric response to noy source due to energetic electron precipitation. Geophys. Res. Lett. 32, 23041. doi:10.1029/2005gl023041
Sangalli, L., Knudsen, D., Larsen, M., Zhan, T., Pfaff, R., and Rowland, D. (2009). Rocket-based measurements of ion velocity, neutral wind, and electric field in the collisional transition region of the auroral ionosphere. J. Geophys. Res. Space Phys. 114, 13757. doi:10.1029/2008ja013757
Sarris, T. E., Talaat, E. R., Palmroth, M., Dandouras, I., Armandillo, E., Kervalishvili, G., et al. (2020). Daedalus: A low-flying spacecraft for in situ exploration of the lower thermosphere–ionosphere. Geoscientific Instrum. Methods Data Syst. 9, 153–191. doi:10.5194/gi-9-153-2020
Sarris, T. E., Tourgaidis, S., Pirnaris, P., Baloukidis, D., Papadakis, K., Psychalas, C., et al. (2022a). Daedalus MASE (Mission Assessment through Simulation Exercise): A toolset for analysis of in situ missions and for processing global circulation model outputs in the lower thermosphere-ionosphere. Front. Astron. Space Sci. Sec. Space Physics. doi:10.3389/fspas.2022.1048318
Sarris, T. E., Aikio, A., Buchert, S., Clilverd, M., Dandouras, I., Doornbos, E., et al. (2022b). Plasma-Neutral Interactions in the Lower Thermosphere-Ionosphere: The need for in situ measurements to address focused questions, submitted to Bulletin of the American Astronomical Society.
Sarris, T. E. (2019). Understanding the ionosphere thermosphere response to solar and magnetospheric drivers: Status, challenges and open issues. Philosophical Trans. R. Soc. A 377, 20180101. doi:10.1098/rsta.2018.0101
Schunk, R., and Nagy, A. (2009). Ionospheres: Physics, plasma physics, and chemistry. Cambridge University Press.
Sinnhuber, M., Nieder, H., and Wieters, N. (2012). Energetic particle precipitation and the chemistry of the mesosphere/lower thermosphere. Surv. Geophys. 33, 1281–1334. doi:10.1007/s10712-012-9201-3
Sojka, J., Heelis, R., Grebowsky, J., DiJoseph, M., Bachanan, R., Bristow, W., et al. (2001). NASA/TM 2001-209980 understanding plasma interactions with the atmosphere: The geospace electrodynamic connections (GEC) mission. doi:10.13140/RG.2.2.22893.31206
Strangeway, R. J. (2012). The equivalence of joule dissipation and frictional heating in the collisional ionosphere. J. Geophys. Res. Space Phys. 117, 17302. doi:10.1029/2011JA017302
Tesema, F., Partamies, N., Whiter, D. K., and Ogawa, Y. (2022). Types of pulsating aurora: Comparison of model and EISCAT electron density observations. Ann. Geophys. Copernic. GmbH) 40, 1–10. doi:10.5194/angeo-40-1-2022
Tourgaidis, S., and Sarris, T. (2022). Wave-particle interactions toolset: A python-based toolset to model wave-particle interactions in the magnetosphere. Front. Astron. Space Sci. 9, 1005598. doi:10.3389/fspas.2022.1005598
Turunen, E., Verronen, P. T., Seppälä, A., Rodger, C. J., Clilverd, M. A., Tamminen, J., et al. (2009). Impact of different energies of precipitating particles on NOx generation in the middle and upper atmosphere during geomagnetic storms. J. Atmos. Solar-Terrestrial Phys. 71, 1176–1189. doi:10.1016/j.jastp.2008.07.005
Van de Kamp, M., Seppälä, A., Clilverd, M. A., Rodger, C. J., Verronen, P., and Whittaker, I. (2016). A model providing long-term data sets of energetic electron precipitation during geomagnetic storms. J. Geophys. Res. Atmos. 121, 12 520–612, 540. doi:10.1002/2015jd024212
Verronen, P., Andersson, M., Marsh, D., Kovács, T., and Plane, J. (2016). Waccm-d—Whole atmosphere community climate model with D-region ion chemistry. J. Adv. Model. Earth Syst. 8, 954–975. doi:10.1002/2015ms000592
Vogt, J., Marghitu, O., Blagau, A., Pick, L., Stachlys, N., Buchert, S., et al. (2022). Daedalus ionospheric profile continuation (dipcont). Geoscientific Instrum. Methods Data Syst. Discuss. 1, 12. doi:10.5194/gi-2022-12
Wahlund, J.-E., Opgenoorth, H., Häggström, I., Winser, K., and Jones, G. (1992). EISCAT observations of topside ionospheric ion outflows during auroral activity: Revisited. J. Geophys. Res. Space Phys. 97, 3019–3037. doi:10.1029/91ja02438
Xiong, C., Stolle, C., and Lühr, H. (2016). The swarm satellite loss of GPS signal and its relation to ionospheric plasma irregularities. Space weather. 14, 563–577. doi:10.1002/2016sw001439
Keywords: lower thermosphere ionosphere, plasma neutral interactions, in situ measurements, decadal survey, atmosphere space transition region, altitudes below 200 km, LTi, ion neutral coupling
Citation: Sarris T, Palmroth M, Aikio A, Buchert SC, Clemmons J, Clilverd M, Dandouras I, Doornbos E, Goodwin LV, Grandin M, Heelis R, Ivchenko N, Moretto-Jørgensen T, Kervalishvili G, Knudsen D, Liu H-L, Lu G, Malaspina DM, Marghitu O, Maute A, Miloch WJ, Olsen N, Pfaff R, Stolle C, Talaat E, Thayer J, Tourgaidis S, Verronen PT and Yamauchi M (2023) Plasma-neutral interactions in the lower thermosphere-ionosphere: The need for in situ measurements to address focused questions. Front. Astron. Space Sci. 9:1063190. doi: 10.3389/fspas.2022.1063190
Received: 06 October 2022; Accepted: 20 December 2022;
Published: 30 January 2023.
Edited by:
Benoit Lavraud, UMR5804 Laboratoire d’astrophysique de Bordeaux (LAB), FranceReviewed by:
Joseph Huba, Syntek Technologies, United StatesCopyright © 2023 Sarris, Palmroth, Aikio, Buchert, Clemmons, Clilverd, Dandouras, Doornbos, Goodwin, Grandin, Heelis, Ivchenko, M-Jørgensen, Kervalishvili, Knudsen, Liu, Lu, Malaspina, Marghitu, Maute, Miloch, Olsen, Pfaff, Stolle, Talaat, Thayer, Tourgaidis, Verronen and Yamauchi. This is an open-access article distributed under the terms of the Creative Commons Attribution License (CC BY). The use, distribution or reproduction in other forums is permitted, provided the original author(s) and the copyright owner(s) are credited and that the original publication in this journal is cited, in accordance with accepted academic practice. No use, distribution or reproduction is permitted which does not comply with these terms.
*Correspondence: Theodoros Sarris, dHNhcnJpc0BlZS5kdXRoLmdy; Minna Palmroth, bWlubmEucGFsbXJvdGhAaGVsc2lua2kuZmk=
Disclaimer: All claims expressed in this article are solely those of the authors and do not necessarily represent those of their affiliated organizations, or those of the publisher, the editors and the reviewers. Any product that may be evaluated in this article or claim that may be made by its manufacturer is not guaranteed or endorsed by the publisher.
Research integrity at Frontiers
Learn more about the work of our research integrity team to safeguard the quality of each article we publish.