- 1Department of Physics and Astronomy, The University of Minnesota, Minneapolis, MN, United States
- 2NASA Goddard Space Flight Center, Greenbelt, MD, United States
- 3Department of Atmospheric and Oceanic Sciences, The University of California, Los Angeles, Los Angeles, CA, United States
- 4Department of Physics and Astronomy, The University of Iowa, Iowa City, IA, United States
- 5Department of Physics, The University of Maryland at College Park, College Park, MD, United States
- 6The University of Colorado LASP, Boulder, CO, United States
- 7The University of Texas at Dallas, Richardson, TX, United States
- 8The Johns Hopkins Applied Physics Laboratory, Laurel, MD, United States
Microbursts are impulsive (<1s) injections of very energetic to relativistic electrons (energies from a few keV to MeV) into Earth’s atmosphere. They are important because they may represent a major loss process for the outer radiation belt (Ripoll and Claudepierre and Ukhorskiy and Colpitts and Li and Fennell and Crabtree, J. Geophys. Res. Space Physics, 2020, 125–e2019JA026735). Understanding and quantifying the underlying causes and consequences plus relative importance of microburst precipitation represent outstanding questions in radiation belt physics and may have significant implications ranging from space weather to atmospheric chemistry. Chorus waves are the likely dominant cause of microburst precipitation, but important questions remain regarding the exact nature of the resonance generating the microbursts and the overall importance of the precipitation. These important questions are limited by lack of systematic coordination of simultaneous observations of causative waves in the magnetosphere and resulting precipitating particles at low altitudes. Multi-spacecraft missions dedicated to answering these questions, themselves required to make progress in radiation belt physics, are critical.
Introduction and background
Many competing processes contribute to the formation and depletion of Earth’s radiation belts (see reviews by Thorne, 2010; Millan and Thorne, 2007; Ripoll et al, 2020). The outer radiation belt is highly dynamic, as many competing energization, loss and transport processes occur simultaneously and are energy dependent. Understanding and quantifying the importance of each process is fundamental to radiation belt physics and has significant implications ranging from human space flight (Lanzerotti and Baker, 2017) to space weather forecasting and atmospheric chemistry (Andersson et al., 2014; Mironova et al., 2015; van de Kamp et al., 2016; Meredith et al., 2017) and even climatology (e.g., Seppala et al., 2014; Matthes et al., 2017).
Microbursts are impulsive (<1s) injections of energetic (few keV to MeV) electrons into the atmosphere. They are important because they may represent a major loss mechanism from the outer radiation belt during storm main and recovery phases (O’Brien et al., 2004; Thorne et al., 2005; Millan and Thorne, 2007). Low energy (10s of keV) microburst precipitation has been observed since the 1960s via balloon measurements of bremsstrahlung X-rays produced by precipitating electrons when they enter Earth’s atmosphere (Winckler et al., 1962; Anderson and Milton, 1964; Millan et al, 2002). Microbursts at higher energies (>100 s keV) have also been observed on satellites (Imhof and Nightingale, 1992; Blake et al., 1996). Figure 1 (from Lorentzen et al., 2001) shows observations of >1 MeV electron flux taken by the Solar Anomalous and Magnetospheric Particle Explorer (SAMPEX) satellite, demonstrating that although the microbursts are short-lived, they can have fluxes more than an order of magnitude higher than the background precipitation. Therefore, microburst precipitation has the potential to be a significant loss mechanism for outer radiation belt energetic electrons.
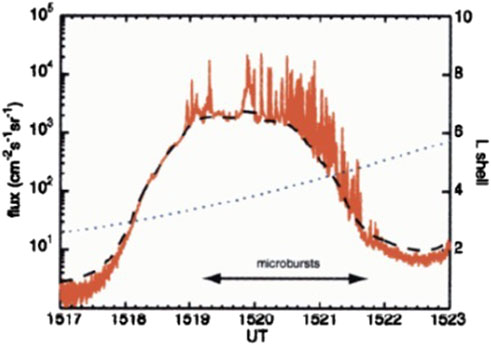
FIGURE 1. Electron microburst observations from Lorentzen et al., 2001. Electrons >1 MeV microbursts observed on 19 October 1998 (red). Estimate of the locally trapped population (black dashed line). L-shell position of satellite (blue dotted line).
Recent studies have also suggested that relativistic electron microbursts are the high-energy tail of pulsating aurora (Miyoshi et al., 2020), and a high correlation between patchy aurora (a type of pulsating aurora) and >1 MeV microbursts was found by Shumko et al. (2021a). Figure 2 shows an example of concurrent pulsating aurora and relativistic electron microbursts. Microbursts are clearly observed at times when SAMPEX passes through pulsating aurora patches, however, the correlation is imperfect in part due to the uncertainty in the mapping. Physically, the imperfect correlation can also arise if the high-energy tail of a pulsating aurora patch does not exceed 1 MeV. Jones et al. (2013) analyzed a pulsating auroral event with a duration of >15 h with an extent larger than 10 h in MLT, indicating that these aurora events are more significant than previously predicted. These studies suggest electron microbursts have important implications for magnetosphere-ionosphere coupling and, in turn atmospheric composition. Seppala et al. (2018) modeled a 6-h microburst ‘storm’ with typical characteristics and found that this precipitation significantly altered NOx and HOx, resulting in a >10% decrease in both middle mesospheric and upper mesospheric ozone, which has implications for regional weather. Further understanding of energetic particle precipitation at Earth will aid in interpretation of similar processes in different plasma regimes. Despite a long history of observations, the detailed physics of the underlying scattering mechanism and the relative importance of microburst precipitation as a loss mechanism for outer belt energetic electrons is poorly understood (see review by Blum and Breneman, 2020; Breneman et al., 2017, Douma et al., 2019).
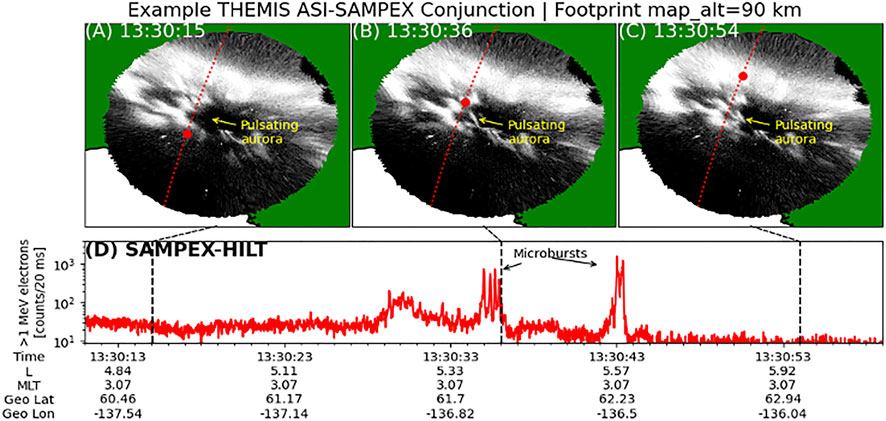
FIGURE 2. An example THEMIS ASI-SAMPEX conjunction with concurrent pulsating aurora and relativistic microbursts. (A–C) show auroral images projected onto a geographic map. Superposed on the map are the full and instantaneous SAMPEX satellite footprints, shown by the red dotted line and circle, respectively. (D) shows the >1 MeV electron precipitation observed by the HILT instrument onboard SAMPEX. Microbursts were clearly observed between 13:30:33-13:30:43 while SAMPEX passed through pulsating aurora patches. The minor ticks are at every second.
Current understanding and critical knowledge gaps
To date, strong evidence suggests that the dominant cause of microburst precipitation is through resonant wave-particle interactions with whistler mode chorus waves (Nakamura et al., 2000; Lorentzen et al., 2001; Breneman et al., 2017; Colpitts et al., 2020). Electromagnetic ion cyclotron (EMIC) waves are another type of plasma wave that can scatter electrons (typically in the MeV energies) through anomalous cyclotron resonant interactions (Thorne and Kennel, 1971). Recent observations have shown evidence that EMIC wave precipitation may extend to lower energies (e.g., Capannolo et al., 2021) in the hundreds of keV range, supported by simulation (Denton et al., 2019), and only recently has evidence of EMIC-driven microburst precipitation been discovered (Shumko et al., 2022). Therefore, investigating resonant interactions with EMIC waves is important in the overall understanding of microburst precipitation. Although chorus is the likely dominant driver of microbursts, many details regarding the scattering process, including the latitude where the interaction takes place and the exact nature of the resonance, remain unverified or unknown. Additionally, direct causation has only been shown in limited cases (e.g., Breneman et al., 2017). Furthermore, basic details of microbursts that are necessary to ascertain their significance as a loss mechanism including duration, isotropy, repetition, and temporal versus spatial variability are still largely unknown (e.g., Shumko et al., 2021b). The reason why these unanswered questions exist is largely due to lack of simultaneous observations of microbursts and waves over a large region and extended period of time.
Recently, studies utilizing a conjunction dataset of high-altitude chorus wave observations from Van Allen Probes (RBSP) and microburst observations on the low-altitude Focused Investigations of Relativistic Electron Burst: Intensity, Range, and Dynamics (FIREBIRD) CubeSats (Spence et al., 2012; Crew et al., 2016) have unveiled details of the connection between chorus and microbursts. Breneman et al. (2017) used a conjunction event to prove the chorus/microburst connection, but this event only led to a very uncertain estimate of the importance of microbursts as a loss mechanism. The relative importance of microbursts as a loss process cannot be fully constrained without accurate measurements of the flux of microbursts through a given area over a given amount of time. Obtaining accurate measurements requires very good temporal resolution, energy resolution, and angular resolution, the combination of which is extremely challenging for existing instrument technology. Future missions focused on addressing this question are needed to enable an accurate determination of the loss due to microbursts in a way that it can be compared with the overall loss from the radiation belts. Furthermore, missions focused on the properties and extents of various plasma waves and their effects on energetic electrons in the inner magnetosphere are necessary to progress in our global understanding of microburst precipitation.
Another area that needs further investigation is how chorus wave properties, which change with geomagnetic activity and location, affect wave-induced precipitation. These properties are important for the computation of diffusion coefficients, which would be valuable for modeling long-term dynamics of radiation belts due to wave-particle interaction. The morphology of the wave regions is also important for understanding the significance of microburst precipitation. A recent study by Elliott et al. (2022) combined observations of chorus and microburst precipitation from nearly all available satellite-borne and ground-based data to estimate the typical size and duration of a microburst precipitation region. Even in this best-case scenario of available observations, significant assumptions were required regarding the continuity of chorus between two observational points. In addition, substantial gaps existed in regions of the magnetosphere due to lack of spacecraft observations. Figure 3 (from Elliott et al., 2022) shows the microburst precipitation and chorus extent for three intervals. Regions of overlap between the chorus wave and microburst precipitation observations were used for the lower estimates. There were regions where microbursts were observed, but there was no spacecraft coverage to detect chorus waves. This study highlights the critical need for constellation missions studying the wave properties along with particle measurements in order to determine where wave-particle interactions can occur, which particle energies resonate with the waves, and the dependence of these regions on geomagnetic activity. Additionally, there exists a latitude gap of missing data between the equatorial source region of chorus waves and the regions where the scattering occurs.
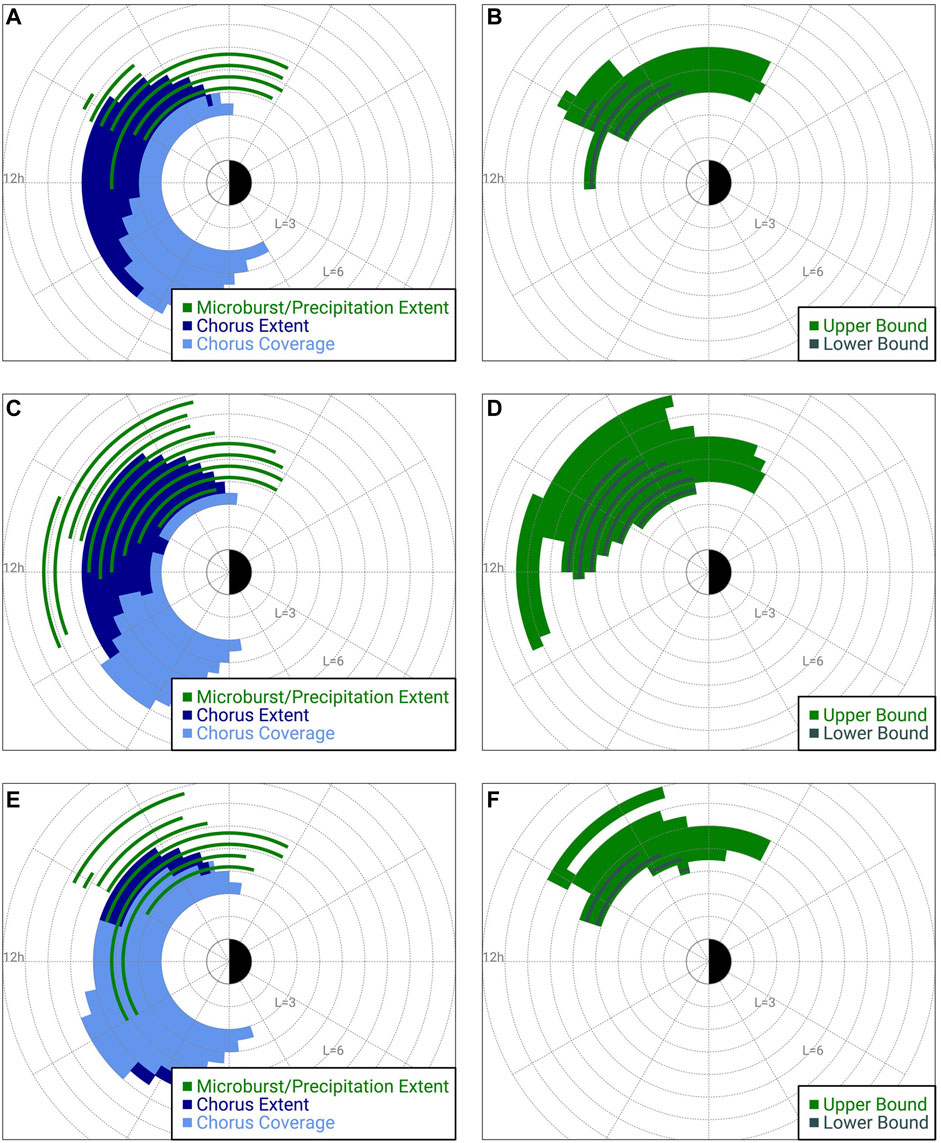
FIGURE 3. From Elliott et al. (2022) showing chorus observations from RBSP, Arase, and ground-based VLF stations, and microburst precipitation from FIREBIRD, AeroCube-6, and POES. (A,C,E) Overview of three periods of persistent chorus and microburst precipitation observations. Green bars represent microburst precipitation extent between 4 and 14 MLT. Dark blue bars show the chorus extent. Light blue bars show the chorus coverage. (B,D,F) Upper (green) and lower (gray) bounds on the size of the microburst-producing chorus region. Green bars (upper bound, in B,D,F) include regions where chorus and microburst precipitation are observed and regions where only microbursts are observed but no chorus waves due to lack of coverage.
Conclusion and science priorities
Important questions remain on microburst precipitation by waves in the inner magnetosphere, including, but not limited to, the exact nature of the resonance generating the microbursts, including the extent of the resonance region off the equator, and the overall importance microburst precipitation has on radiation belt electron loss. These gaps in our understanding largely exist due to the sparseness of simultaneous observations of waves and particles. Equatorial satellites are ideal for measuring in situ wave populations, such as chorus, generated near the equator. However, these satellites are typically unable to resolve electrons in the loss cone to quantify the amount of loss. In a similar vein, low Earth orbiting satellites, like FIREBIRD, are able to directly observe microburst precipitation, but are far away from the wave source region. This makes direct testing of microburst generation very difficult due to the large separation over which the waves and microbursts are observed. The current state of knowledge is therefore limited to multipoint observations and magnetic conjunctions between satellites.
It is important to improve our understanding of how and where plasma waves generate energetic electron microburst precipitation. Continued support of projects that investigate multipoint observations of waves ands microburst precipitation is necessary to make incremental, important steps towards our understanding of the generation mechanisms for microbursts. Modeling, including quasi-linear methods, ray tracing, and test particle simulations, will help us understand the mechanisms of the scattering process. Recent studies (e.g., Chen et al. 2020; Colpitts et al., 2020; Miyoshi et al. 2020; Chen et al. 2022) used ray tracing simulations and satellite observations to show direct evidence of chorus elements propagating from the equator to higher latitudes where microbursts can be produced. This was a critical step towards understanding such a fundamental radiation belt physics question. However, the flux of microbursts through a given area in a given amount of time remains unknown and is necessary to quantify the contribution of this process to radiation belt loss. A mission with both an imager and particle detector, which could directly measure the particles while imaging a significant sector of the precipitation region in order to quantify the flux and spectrum of microburst precipitation throughout the region, is desirable. In addition, constellation missions dedicated towards answering these questions are essential. Measurements of both waves and particles along magnetic field lines from where the waves are generated near the magnetic equator, up to higher latitudes would provide insight into the structure of the waves and the impact they have on the particle populations. Additionally, the generation regions of microbursts and wave properties responsible could be identified, which would significantly constrain the spatial and temporal scale of microburst precipitation.
The importance of microburst precipitation relative to other radiation belt loss processes has yet to be established. Breneman et al. (2017) estimated from FIREBIRD microburst data the differential flux loss rate to the atmosphere due to microbursts. This was then used to estimate the amount of time it would take for this mechanism to deplete the entire outer radiation belt. This loss rate was then compared to other loss rates in order to determine the relative importance of microburst precipitation. However, the differential flux loss rate is poorly constrained due to lack of accurate measurements of the microburst flux within a given area over a given amount of time. Measuring the spatial distribution and energy spectrum of precipitating electrons, including microbursts, is therefore required to accurately determine the loss timescales. Further complications arise from other competing mechanisms for radiation belt electron loss, including magnetopause shadowing (e.g. Tu et al., 2019; Staples et al., 2022), therefore making it difficult to separate and quantify the relative importance of each. Improved measurement capabilities, including good temporal resolution, energy resolution, and angular resolution are needed. We also recommend constellation missions, including multipoint observations at low Earth orbit and wave and particle measurements along field lines at varying latitudes. This would provide wave and particle measurements near where the scattering is occurring in an effort to fully understand the overall importance of microburst precipitation.
Author contributions
All authors listed have made a substantial, direct, and intellectual contribution to the work and approved it for publication.
Funding
Work at the University of Minnesota and NASA Goddard was supported by the NASA Heliophysics Supporting Research program NNH18ZDA001N-HSR award 80NSSC19K0842. AH and LB were in part supported by the Space Precipitation Impacts project at Goddard Space Flight Center through the Heliophysics Internal Science Funding Model.
Conflict of interest
The authors declare that the research was conducted in the absence of any commercial or financial relationships that could be construed as a potential conflict of interest.
Publisher’s note
All claims expressed in this article are solely those of the authors and do not necessarily represent those of their affiliated organizations, or those of the publisher, the editors and the reviewers. Any product that may be evaluated in this article, or claim that may be made by its manufacturer, is not guaranteed or endorsed by the publisher.
References
Anderson, K. A., and Milton, D. W. (1964). Balloon observations of X-rays in the auroral zone: 3. High time resolution studies. J. Geophys. Res. 69 (21), 4457–4479. doi:10.1029/JZ069i021p04457
Andersson, M. E., Verronen, P. T., Rodger, C. J., Cliverd, M. A., and Seppälä, A. (2014). Missing driver in the Sun-Earth connection from energetic electron precipitation impacts mesospheric ozone. Nat. Commun. 5, 5197. doi:10.1038/ncomms6197
Blake, J. B., Looper, M. D., Baker, D. N., Nakamura, R., Klecker, B., and Hovestadt, D. (1996). New high temporal and spatial resolution measurements by SAMPEX of the precipitation of relativistic electrons. Adv. Space Res. 18 (8), 171–186. doi:10.1016/0273-1177(95)00969-8
Blum, L. W., and Breneman, A. W. (2020). “Chapter 3—observations of radiation belt losses due to cyclotron wave-particle interactions ’’in The dynamic loss of Earth’s radiation belts. Tharamani, Chennai: Elsevier. doi:10.1016/B978-0-12-813371-2.00003-2
Breneman, A. W., Crew, A., Sample, J., Klumpar, D., Johnson, A., Agapitov, O., et al. (2017). Observations directly linking relativistic electron microbursts to whistler mode chorus: Van Allen Probes and FIREBIRD II. Geophys. Res. Lett. 44 (22), 11265. doi:10.1002/2017GL075001
Capannolo, L., Li, W., Spence, H., Johnson, A. T., Shumko, M., Sample, J., et al. (2021). Energetic electron precipitation observed by FIREBIRD-II potentially driven by EMIC waves: Location, extent, and energy range from a multievent analysis. Geophys. Res. Lett. 48 (5), e2020GL091564. doi:10.1029/2020GL091564
Chen, L., Breneman, A. W., Xia, Z., and Zhang, X.-J. (2020). Modeling of bouncing electron microbursts induced by ducted chorus waves. Geophys. Res. Lett. 47, e2020GL089400. doi:10.1029/2020GL089400
Chen, L., Zhang, X.-J., Artemyev, A., Angelopoulos, V., Tsai, E., Wilkins, C., et al. (2022). Ducted chorus waves cause sub-relativistic and relativistic electron microbursts. Geophys. Res. Lett. 49, e2021GL097559. doi:10.1029/2021GL097559
Colpitts, C., Miyoshi, Y., Kasahara, Y., Delzanno, G. L., Wygant, J. R., Cattell, C. A., et al. (2020). First direct observations of propagation of discrete Chorus elements from the equatorial source to higher latitudes, using the Van Allen probes and Arase satellites. JGR. Space Phys. 125 (10), e2020JA028315. doi:10.1029/2020JA028315
Crew, A. B., Spence, H. E., Blake, J. B., Klumpar, D. M., Larsen, B. A., O'Brien, T. P., et al. (2016). First multipoint in situ observations of electron microbursts: Initial results from the NSF FIREBIRD II mission. J. Geophys. Res. Space Phys. 121 (6), 5272–5283. doi:10.1002/2016ja022485
Denton, R. E., Ofman, L., Shprits, Y. Y., Bortnik, J., Millan, R. M., Rodger, C. J., et al. (2019). Pitch angle scattering of sub-MeV relativistic electrons by electromagnetic ion cyclotron waves. JGR. Space Phys. 124, 5610–5626. doi:10.1029/2018JA026384
Douma, E., Rodger, C., Blum, L., O’Brien, T., Clilverd, M., and Blake, J. (2019). Characteristics of relativistic microburst intensity from SAMPEX observations. JGR. Space Phys. 124 (7), 5627–5640. doi:10.1029/2019JA026757
Elliott, S. S., Breneman, A. W., Colpitts, C., Pettit, J. M., Cattell, C. A., Halford, A. J., et al. (2022). Quantifying the size and duration of a microburst-producing chorus region on 5 December 2017. Geophys. Res. Lett. 49–e2022GL099655. doi:10.1029/2022GL099655
Imhof, W. L., and Nightingale, R. W. (1992). Relativistic electron enhancements observed over a range of L shells trapped at high altitudes and precipitating at low altitudes into the atmosphere. J. Geophys. Res. 97 (A5), 6397–6403. doi:10.1029/92ja00229
Jones, S. L., Lessard, M. R., Rychert, K., Spanswick, E., Donovan, E., and Jaynes, A. N. (2013). Persistent, widespread pulsating aurora: A case study. JGR. Space Phys. 118, 2998–3006. doi:10.1002/jgra.50301
Lanzerotti, L. J., and Baker, D. N. (2017). Space weather research: Earth’s radiation belts. Space weather. 15, 742–745. doi:10.1002/2017SW001654
Lorentzen, K. R., Looper, M. D., and Blake, J. B. (2001). Relativistic electron microbursts during the GEM storms. Geophys. Res. Lett. 28 (13), 2573–2576. doi:10.1029/2001gl012926
Matthes, K., Funke, B., Andersson, M. E., Barnard, L., Beer, J., Charbonneau, P., et al. (2017). Solar forcing for CMIP6 (v3.2). Geosci. Model Dev. 10, 2247–2302. doi:10.5194/gmd-10-2247-2017
Meredith, N. P., Horne, R. B., Sandberg, I., Papadimitriou, C., and Evans, H. D. R. (2017). Extreme relativistic electron fluxes in the Earth’s outer radiation belt: Analysis of INTEGRAL IREM data. Space weather. 15, 917–933. doi:10.1002/2017SW001651
Millan, R. M., Lin, R. P., Smith, D. M., Lorentzen, K. R., and McCarthy, M. P. (2002). X-ray observations of MeV electron precipitation with a balloon-borne germanium spectrometer. Geophys. Res. Lett. 29 (24), 47-1–47-44. doi:10.1029/2002GL015922
Millan, R. M., and Thorne, R. M. (2007). Review of radiation belt relativistic electron losses. J. Atmos. Sol. Terr. Phys. 69, 362–377. doi:10.1016/j.jastp.2006.06.019
Mironova, I. A., Aplin, K. L., Arnold, F., Bazilevskaya, G. A., Harrison, R. G., Krivolutsky, A. A., et al. (2015). Energetic particle influence on the earth’s atmosphere. Space Sci. Rev. 194 (1-4), 1–96. doi:10.1007/s11214-015-0185-4
Miyoshi, Y., Saito, S., Kurita, S., Asamura, K., Hosokawa, K., Sakanoi, T., et al. (2020). Relativistic electron microbursts as high energy tail of pulsating Aurora electrons. Geophys. Res. Lett. 47 (21), e2020GL090360. doi:10.1029/2020GL090360
Nakamura, R., Isowa, M., Kamide, Y., Baker, D. N., Blake, J. B., and Looper, M. (2000). SAMPEX observations of precipitation bursts in the outer radiation belt. J. Geophys. Res. 105 (A7), 15875–15885. doi:10.1029/2000JA900018
O'Brien, T. P., Looper, M. D., and Blake, J. B. (2004). Quantification of relativistic electron microburst losses during the GEM storms. Geophys. Res. Lett. 31, L04802. doi:10.1029/2003GL018621
Ripoll, J.-F., Claudepierre, S. G., Ukhorskiy, A. Y., Colpitts, C., Li, X., Fennell, J., et al. (2020). Particle dynamics in the earth's radiation belts: Review of current research and open questions. J. Geophys. Res. Space Phys. 125, e2019JA026735. doi:10.1029/2019JA026735
Seppälä, A., and Clilverd, M. A. (2014). Energetic particle forcing of the northern hemisphere winter stratosphere: Comparison to solar irradiance forcing. Front. Phys. 2, 25. doi:10.3389/fphy.2014.00025
Seppälä, A., Douma, E., Rodger, C., Verronen, P., Clilverd, M. A., and Bortnik, J. (2018). Relativistic electron microburst events: Modeling the atmospheric impact. Geophys. Res. Lett. 45, 1141–1147. doi:10.1002/2017GL075949
Shumko, M., Blum, L. W., and Crew, A. B. (2021b). Duration of individual relativistic electron microbursts: A probe into their scattering mechanism. Geophys. Res. Lett. 48, e2021GL093879. doi:10.1029/2021GL093879
Shumko, M., Gallardo-Lacourt, B., Halford, A. J., Liang, J., Blum, L. W., Donovan, E., et al. (2021a). A strong correlation between relativistic electron microbursts and patchy aurora. Geophys. Res. Lett. 48 (18), e2021GL094696. doi:10.1029/2021GL094696
Shumko, M, Gallardo-Lacourt, B, Halford, A, Blum, L, Liang, J, Miyoshi, Y, et al. (2022). Proton aurora and relativistic electron microbursts scattered by electromagnetic ion cyclotron waves. Front. Astron. Space Sci. 9, 975123. doi:10.3389/fspas.2022.975123
Spence, H. E., Blake, J. B., Crew, A. B., Driscoll, S., Klumpar, D. M., Larsen, B. A., et al. (2012). Focusing on size and energy dependence of electron microbursts from the van allen radiation belts. Space weather. 10. doi:10.1029/2012SW000869
Staples, F. A., Kellerman, A., Murphy, K. R., Rae, I. J., Sandhu, J. K., and Forsyth, C. (2022). Resolving magnetopause shadowing using multimission measurements of phase space density. J. Geophys. Res. Space Phys. 127, e2021JA029298. doi:10.1029/2021JA029298
Thorne, R. M., and Kennel, C. F. (1971). Relativistic electron precipitation during magnetic storm main phase. J. Geophys. Res. 76 (19), 4446–4453. doi:10.1029/ja076i019p04446
Thorne, R. M., O’Brien, T. P., Shprits, Y. Y., Summers, D., and Horne, R. B. (2005). Timescale for MeV electron microburst loss during geomagnetic storms. J. Geophys. Res. 110, A09202. doi:10.1029/2004JA010882
Thorne, R. M. (2010). Radiation belt dynamics: The importance of wave particle interactions. Geophys. Res. Lett. 37 (22), L22107. doi:10.1029/2010GL044990
Tu, W., Xiang, Z., and Morley, S. K. (2019). Modeling the magnetopause shadowing loss during the June 2015 dropout event. Geophys. Res. Lett. 46, 9388–9396. doi:10.1029/2019GL084419
van de Kamp, M., Seppälä, A., Clilverd, M. A., Rodger, C. J., Verronen, P. T., and Whittaker, I. C. (2016). A model providing long‐term data sets of energetic electron precipitation during geomagnetic storms. J. Geophys. Res. Atmos. 121 (520–12), 12, 520–12, 540. doi:10.1002/2015JD024212
Keywords: microbursts, chorus, radiation belts, precipitation, wave-particle interactions
Citation: Elliott SS, Breneman A, Colpitts C, Bortnik J, Jaynes A, Halford A, Shumko M, Blum L, Chen L, Greeley A and Turner D (2022) Understanding the properties, wave drivers, and impacts of electron microburst precipitation: Current understanding and critical knowledge gaps. Front. Astron. Space Sci. 9:1062422. doi: 10.3389/fspas.2022.1062422
Received: 05 October 2022; Accepted: 25 October 2022;
Published: 03 November 2022.
Edited by:
Michael G. Henderson, Los Alamos National Laboratory (DOE), United StatesReviewed by:
Jean-Francois Ripoll, CEA DAM Île-de-France, FranceBrian Larsen, Los Alamos National Laboratory (DOE), United States
Copyright © 2022 Elliott, Breneman, Colpitts, Bortnik, Jaynes, Halford, Shumko, Blum, Chen, Greeley and Turner. This is an open-access article distributed under the terms of the Creative Commons Attribution License (CC BY). The use, distribution or reproduction in other forums is permitted, provided the original author(s) and the copyright owner(s) are credited and that the original publication in this journal is cited, in accordance with accepted academic practice. No use, distribution or reproduction is permitted which does not comply with these terms.
*Correspondence: Sadie S. Elliott, dGV0cmkwMDZAdW1uLmVkdQ==