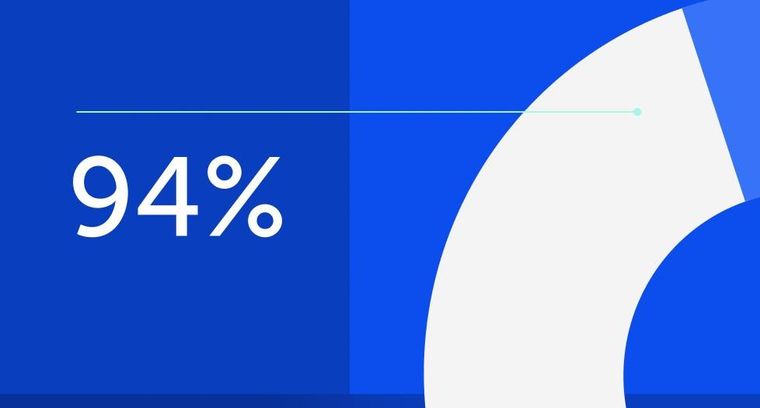
94% of researchers rate our articles as excellent or good
Learn more about the work of our research integrity team to safeguard the quality of each article we publish.
Find out more
OPINION article
Front. Astron. Space Sci., 04 January 2023
Sec. Space Physics
Volume 9 - 2022 | https://doi.org/10.3389/fspas.2022.1051527
This article is part of the Research TopicThe Future of Space Physics 2022View all 66 articles
Difficulties associated with receiving telemetry from satellites severely limit the volume of scientific data that can be downlinked to the ground. Current missions employ techniques such as compressing and pruning datasets to reduce the data volume they transmit. While existing mission designs are already restricted by limited telemetry budgets, future Heliophysics System Observatory missions will produce ever larger data volumes with higher resolution and cadence observations from constellations of satellites spread throughout the heliosphere1. In addition, heliophysics missions often produce data for the operational Space Weather community that requires a low latency between observation and downlink. In light of current limitations, the infrastructure to receive NASA satellite telemetry must be expanded and modernized to support the science needs of future data-rich heliophysics missions.
Communications with NASA science missions are primarily routed through the Deep Space Network (DSN) (Fearey and Renzetti, 1969) and the Near Space Network (NSN) (Monaghan and Dunbar, 2021) using S, X, K, and Ka band radio transmissions. The DSN, primarily used for communicating with missions in or beyond lunar orbit, consists of three ground stations, each with four or six large antennas, separated by ∼120° in longitude. The NSN, used to communicate with satellites near Earth from launch out to cis-Lunar orbits, encompases a number of ground stations with smaller antennas over a wide range of longitudes and latitudes. The NSN also includes the Tracking Data Relay Satellite constellation in geosynchronous orbit, along with its two ground stations.
The different capabilities of these networks lead to unique communication difficulties. A Low Earth Orbit satellite may only have a line of sight connection to an NSN ground station once per day for a 10 min pass, necessitating rapid data transfer. A mission far from the Earth nearly always has a line of sight to at least one DSN station when the Earth is in view, but must compete with other satellites for downlink time2. These communication restrictions limit the volume of data that can be downlinked and delay when those data reach the ground.
It is this relative sparsity of downlink time, utilizing any network, that causes science data to be pruned and compressed before they are transmitted to the ground. Scientists must select for transmission only the data that are most likely to satisfy their science goals. This slows discovery by taking time and resources away from science investigations and creating unintended biases towards preferred phenomena. To further our understanding of underlying physics requires complete in situ and remote sensing data sets to be delivered in larger volumes than ever before. Additionally, to further our capability to predict space weather impacts requires these data to be delivered with low latency.
The Magnetospheric Multiscale (MMS) Mission (Burch et al., 2016) measures the plasma, particle, and electromagnetic field conditions throughout the Earth’s magnetosphere. Due to its relatively close proximity to Earth, MMS communicates through both NASA networks, but only downlinks data using the DSN. Over the life of the mission, only about 4% of the high resolution burst mode data has been downloaded, while the rest are deleted to make room for new data. The selection of which data to downlink is often performed by volunteers and takes valuable time that could be better used studying those same data.
The Parker Solar Probe (PSP) (Fox et al., 2016) studies the young solar wind and inner heliosphere in a highly eccentric heliocentric orbit. Because of its large distance from the Earth, PSP communicates with Earth exclusively through the DSN. Telemetry contacts occur near aphelion, outside of the prime observing windows and when PSP is at maximum elongation from the Sun. Subsets of data are downlinked and used to choose what full-resolution data will be transmitted. For example, the SWEAP instrument onboard PSP records data at ∼0.2 s temporal resolution but only initially downlinks survey-mode data with 7 s resolution. It is then up to operators to determine which high resolution data to downlink. Due to the nature of PSP’s orbit, the decisions about which burst-mode data to downlink from a months long orbit must be made quickly, occasionally within only a few days.
One strategy used to avoid limitations with data retrieval was the construction of a dedicated downlink station for the Solar Dynamics Observatory (SDO) (Pesnell et al., 2012). This design choice enables SDO to collect and downlink well over a terabyte of compressed science data daily from its geosynchronous orbit. The price for this capability was ∼2.5% of the mission cost and ∼5–10% of its annual operating budget. While this may not be feasible for every heliophysics mission, it demonstrates the possibility of designing missions with 100% science data retrieval, even with extreme data volumes, at a modest expense.
While originally built to enable the Pioneer lunar missions and used prominently to communicate with NASA planetary science satellites, the DSN has been an important resource for NASA Heliophysics missions since the 1974 launch of the Helios-1 mission (Goodwin, 1976). Today, a significant fraction of DSN time is allocated to Heliophysics missions. Between 2010 and 2018, ∼35% of the DSN capacity was used to communicate with Heliophysics missions, with the largest users being Voyagers 1 and 2 (∼10% of all DSN time), STEREO A and B (∼6%) and SOHO (∼6%). During this period, ∼58% of the DSN capacity was allocated to planetary science missions while the remaining ∼7% was split between astrophysics missions and other occasional incidental contacts (Omo, 2018).
Accommodating the data requirements of future missions while maintaining the strong science output from current missions will require deliberate attention and support toward improving existing data transfer capabilities (Giovannoni et al., 2021). In particular, as there is an increasing need for heliophysics observatories to move throughout the heliosphere, e.g., PSP, Solar Orbiter (Müller et al., 2020), Vigil (Pulkkinen, 2019), and future 4π-constellation observations of the solar surface, they will increasingly rely on the DSN for their communication needs. The heliophysics community should coordinate with other DSN users to support DSN operations and advocate for the necessary upgrades to expand current downlink capabilities that would accommodate future solar and heliospheric missions.
Heliophysics missions should also take advantage of every opportunity to increase their data downlink capacity within the existing DSN constraints. This could include designing satellites with improved communication systems that use higher-power transmitters and/or larger, higher gain antennas. Another option without significant design changes is to utilize Ka-rather than X-band communications (such as is done by PSP) where the factor of four increase in frequency enables proportional increases in data rates.
The DSN Aperture Enhancement Project proposed in 2009 is nearing completion. This upgrade planned to add six new 34-m receivers and 80 kW high power transmitters, combined with scheduled decommissioning, to yield an increased capacity of at least 30% by 2025. This enhancement was designed with a budget of $362.4 million (NASA Office of Inspector General Office of Audits, 2015), less than the cost of two Medium-Class Explorer missions.
However, this recent upgrade is not enough to meet the current, let alone future deep space communication demands. In a 2021 presentation to the planetary science decadal survey steering committee Brad Arnold, manager of the DSN at the Jet Propulsion Laboratory, warned that “we’re trying to add capacity and more antennas, but we can’t keep up with the demand that’s currently out there, so missions should expect to be getting less availability.” (Foust, 2021) And this will get worse, with oversubscription increasing from 20% today to 40% over the next decade. He also noted that the upcoming return to the Moon with the Artemis program is “the gorilla in the room” since communication supporting human spaceflight rightly has priority over uncrewed satellites. Additionally, a 2020 NASA Office of Inspector General audit of NASA’s planetary science portfolio concluded that “NASA has not adequately funded Deep Space Network (DSN) repair, maintenance, and modernization efforts.” (NASA Office of Inspector Gneral Office of Audits, 2020) But the DSN is not important only for the NASA Planetary Science Division, the future success of the Heliophysics System Observatory requires a fully-funded and expanded DSN.
In the longer term, the DSN must accelerate its capacity growth. Between 1980 and 2010 the DSN capacity increased by about two orders of magnitude, driven largely by the adoption of Ka-band communication and the development of techniques to combine antennas into arrays. But the expectation is that demand willl continue to grow by about an order of magnitude per decade through 2065 (Leslie, 2016). Meeting this exponential growth in demand without commensurate costs will require technological innovation and coordination with stakeholders, including the heliophysics science community.
Future heliophysics missions should also explore alternate communication modalities and technologies to increase data return capacity. One way in which this could be accomplished is through networked communications architectures, such as the Mars Relay Network (Edwards, 2007) and the proposed LunaNet (Israel et al., 2020), facilitating indirect ground communication. This could enable heliophysics science from unique perspectives, such as the L3 point behind the Sun from the Earth’s perspective, and with improved reliability, latency, and bandwidth by providing alternate data transmission pathways. These missions should also explore novel technology to increase bandwidths for data transmission. One promising example is optical laser communications. This technology has successfully been demonstrated numerous times for both up- and down-link in near-Earth and cis-lunar environments and will fly in deep space on the upcoming Psyche (Hart, 2018) mission, where it is expected to provide 10 to 100 times increased bandwidth (Leonard, 2017) over traditional radio communications. Within the Heliophysics Systems Observatory, the SETH (Shelton et al., 2019) mission to investigate energetic neutral atoms in the solar wind that was funded for a 9 month concept study in 2019 showed how Heliophysics missions can utilize a commercially available 2U laser communication module to enable 10 Mbps downlinks over distances greater than 0.1 AU.
Every piece of data measured by a satellite has scientific value. Expanding our ability to receive those data increases the scientific output of missions at a marginal cost. To enable continuing and expanding Heliophysics science, it is essential that NASA ensures the accessibility of future science data returned from Heliophysics System Observatory missions by advocating for and investing in the DSN and embracing the communications technologies of the future.
The manuscript was compiled by SJS with content and editorial contributions from all co-authors.
YR acknowledges support from the Future Faculty postdoctoral fellowship at Harvard University. AH was supported by the Space Precipitation Impacts project at Goddard Space Flight Center through the Heliophysics Internal Science Funding Model.
Modified versions of this paper were submitted as unreviewed white papers to the Heliophysics 2050 Workshop (Schonfeld et al., 2021) and the 2024 Solar and Space Physics Decadal Survey.
The authors declare that the research was conducted in the absence of any commercial or financial relationships that could be construed as a potential conflict of interest.
All claims expressed in this article are solely those of the authors and do not necessarily represent those of their affiliated organizations, or those of the publisher, the editors and the reviewers. Any product that may be evaluated in this article, or claim that may be made by its manufacturer, is not guaranteed or endorsed by the publisher.
1See for example the recently selected Medium-Class Explorer missions (Science Office for Mission Assessments, 2022).
2In addition, as the distance to a spacecraft increases the downlink rate decreases, increasing the time necessary to transmit the same data for satellites at larger distances
Burch, J. L., Moore, T. E., Torbert, R. B., and Giles, B. L. (2016). Magnetospheric Multiscale overview and science objectives. Space Sci. Rev. 199, 5–21. doi:10.1007/s11214-015-0164-9
Edwards, D. C. (2007). Relay communications for Mars exploration. Int. J. Satell. Commun. Netw. 25, 111–145. doi:10.1002/sat.871
Fearey, J. P., and Renzetti, N. A. (1969). The deep space network. Space Eng. 15, 599–639. doi:10.1007/978-94-011-7551-7_42
Foust, J. (2021). Increasing demands putting pressure on deep space network. Boulder, CO: Space News. Available at: https://spacenews.com/increasing-demands-putting-pressure-on-deep-space-network
Fox, N. J., Velli, M. C., Bale, S. D., Decker, R., Driesman, A., Howard, R. A., et al. (2016). The solar Probe plus mission: Humanity’s first visit to our star. Space Sci. Rev. 204, 7–48. doi:10.1007/s11214-015-0211-6
Giovannoni, B., Arnold, B., Levesque, M., Berner, J., Smith, A., Lichten, S., et al. (2021). The deep space network: Enabling richer data sets for future planetary science missions. Bull. Am. Astronomical Soc. 53, 410. doi:10.3847/25c2cfeb.8d429eb4
Goodwin, P. S., Traxler, M. R., Meeks, W. G., and Flanagan, F. M. (1976). Report No. NASA-CR-148197, JPL-TM-33-752-VOL-1. Available at: ntrs.nasa.gov/citations/19760019169.
Hart, W., Brown, G. M., Collins, S. M., De Soria-Santacruz Pich, M., Fieseler, P., Goebel, D., et al. (2018). “Overview of the spacecraft design for the Psyche mission concept,” in IEEE Aerospace Conference (Big Sky Montana), 1–20. doi:10.1109/AERO.2018.8396444
Israel, D. J., Mauldin, K. D., Roberts, C. J., Mitchell, J. W., Pulkkinen, A. A., Cooper, L. V. D., et al. (2020). “LunaNet: aFlexible and extensible lunar exploration communications and navigation infrastructure,” in IEEE Aerospace Conference. Big Sky Montana. “The Future Lunar Communications Architecture” – Interagency Operations Advisory Group (IOAG), Lunar Communications Architecture Working Group. Available at: ntrs.nasa.gov/api/citations/20200001555/downloads/20200001555.pdf.
Leonard, D. (2017). Deep space communications via faraway photons. Pasadena, CA: Jet Propulsion Laboratory. Available at: https://www.jpl.nasa.gov/news/deep-space-communications-via-faraway-photons
Leslie, D. J., Townes, S. A., Liebrecht, P. E., Vrotsos, P. A., and Cornwell, D. M. (2016). Report No. JPL-CL-16-1781. Available at: ntrs.nasa.gov/citations/20190033531.
Monaghan, H., and Dunbar, B. (2021). Near space network. NASA. Available at: https://www.nasa.gov/directorates/heo/scan/services/networks/near_space_network/
Müller, D., St. Cyr, O. C., Zouganelis, I., Gilbert, H. R., Marsden, R., Nieves-Chinchilla, T., et al. (2020). The solar orbiter mission. Astron. Astrophys. 642, A1. doi:10.1051/0004-6361/202038467
NASA Office of Inspector General Office of Audits (2015). Report No. IG-15-013. Available at: oig.nasa.gov/docs/IG-15-013.pdf.
NASA Office of Inspector Gneral Office of Audits (2020). Report No. IG-20-023. Available at: oig.nasa.gov/docs/IG-20-023.pdf.
Omo, M. (2018). FOIA request 18-JPL-F-00829. Available at: https://archive.org/details/JPLDSNData.
Pesnell, W., Thompson, B., and Chamberlin, P. (2012). The solar Dynamics observatory (SDO). Sol. Phys. 275, 3–15. doi:10.1007/s11207-011-9841-3
Pulkkinen, A. A., Bisi, M. M., Luntama, J. P., Kraft, S., Glover, A., and Heil, M. (2019). ESA Lagrange space weather monitoring mission to L5 point. San Francisco California: AGU Fall Meeting Abstracts. Available at: https://ui.adsabs.harvard.edu/abs/2019AGUFMSH24B.01P/abstract
Schonfeld, S. J., Pesnell, W. D., Verniero, J. L., Rivera, Y. J., Halford, A. J., Vines, S. K., et al. (2021). “Communications enabling science from the 2050 heliophysics system observatory,” in Heliophysics 2050 Workshop. doi:10.5281/zenodo.4025392
Science Office for Mission Assessments (2022). 2019 heliophysics medium-class explorer program announcement of opportunity. Washington, DC: NASA. Available at: https://explorers.larc.nasa.gov/HPMIDEX/announcements.html.
Shelton, M., Pulkkinen, A., Summerlin, E. J., and Storm, M. (2019). “SETH technology demonstration of small satellite deep space optical communications to aid heliophysics science and space weather forecasting,” in 33rd Annual AIAA/USU Conference on Small Satellites Logan Utah. Available at: https://digitalcommons.usu.edu/cgi/viewcontent.cgi?article=4526&context=smallsat
Keywords: deep space network (DSN), heliophysics system observatory, satellite communication, satellite data download, networked communications, laser communication, space weather
Citation: Schonfeld SJ, Pesnell WD, Verniero JL, Rivera YJ, Halford AJ, Vines SK and Spitzer SA (2023) Expanding the deep space network to support the heliophysics system observatory. Front. Astron. Space Sci. 9:1051527. doi: 10.3389/fspas.2022.1051527
Received: 22 September 2022; Accepted: 09 November 2022;
Published: 04 January 2023.
Edited by:
Zoltan Sternovsky, University of Colorado Boulder, United StatesReviewed by:
Thomas Earle Moore, Third Rock Research, United StatesCopyright © 2023 Schonfeld, Pesnell, Verniero, Rivera, Halford, Vines and Spitzer. This is an open-access article distributed under the terms of the Creative Commons Attribution License (CC BY). The use, distribution or reproduction in other forums is permitted, provided the original author(s) and the copyright owner(s) are credited and that the original publication in this journal is cited, in accordance with accepted academic practice. No use, distribution or reproduction is permitted which does not comply with these terms.
*Correspondence: S. J. Schonfeld, c2Nob25mc2pAZ21haWwuY29t
†ORCID:S. J. Schonfeld, orcid.org/0000-0002-5476-2794; W. D. Pesnell, orcid.org/0000-0002-8306-2500; J. L. Verniero, orcid.org/0000-0003-1138-652X; Y. J. Rivera, orcid.org/0000-0002-8748-2123; A. J. Halford, orcid.org/0000-0002-5383-4602; S. K. Vines, orcid.org/0000-0002-7515-3285; S. A. Spitzer, orcid.org/0000-0001-9607-1492
Disclaimer: All claims expressed in this article are solely those of the authors and do not necessarily represent those of their affiliated organizations, or those of the publisher, the editors and the reviewers. Any product that may be evaluated in this article or claim that may be made by its manufacturer is not guaranteed or endorsed by the publisher.
Research integrity at Frontiers
Learn more about the work of our research integrity team to safeguard the quality of each article we publish.