- 1Space Science Division, US Naval Research Laboratory, Washington, DC, United States
- 2Geophysical Institute, University of Alaska Fairbanks, Fairbanks, AK, United States
- 3High Altitude Observatory, UCAR, Boulder, CO, United States
- 4Department of Electrical and Computer Engineering, University of Illinois Urbana-Champaign, Champaign, IL, United States
The Earth’s upper atmosphere (85–550 km) is the nearest region of geospace and is highly dynamic in nature. Neutral winds impact a large portion of the dynamics in this region. They play a critical role in determining the state of the ionosphere-thermosphere system at almost all latitudes and altitudes. Their influences range from wave breaking/dissipation in the mesosphere and lower thermosphere to global redistribution of energy and momentum deposited at high latitudes by the magnetosphere. Despite their known importance, global geospace neutral winds have remained one of the least sampled state parameters of the Earth’s upper atmosphere and are still poorly characterized even after multiple decades of observations. This paper presents an overview of historical neutral wind measurements and the critical need for their global height-resolved measurements. Some satellite missions are still operational and deliver valuable information on the contribution of neutral winds in global atmospheric dynamics. However, many significant gaps remain in their global monitoring, and our current understanding of the drivers of neutral winds is incomplete. We discuss the challenges posed by these measurement gaps in understanding geospace physics and weather. Further, we propose some wind observation solutions, including the simultaneous operations of upcoming NASA DYNAMIC and GDC missions as well as support for the development of ground-based observing methodologies, that will lead to fundamental advances in geospace science and address humanity’s emerging space needs.
1 Introduction and motivation
Earth’s upper atmosphere (here defined as 85–550 km) is the nearest region of geospace (i.e., the near-earth space environment). It surrounds and protects the planet. It is a weakly ionized plasma whose ionized and neutral components are typically considered separately as the ionosphere and thermosphere, respectively. The region is highly dynamic in nature and is driven by solar and magnetospheric forcing from above (often impulsively) (e.g., Lu et al., 2016), and continuously by meteorological forcing from the lower atmosphere (e.g., Liu, 2016; Sassi et al., 2019). The weather of this region directly impacts the performance of ground-based and space-based communication and navigation technologies. In addition, a wide variety of low-Earth orbit weather, communications, global navigation, and other operational satellites on which humanity relies on a daily basis, reside in this region of space. Any changes in this atmospheric region can directly impact the orbital trajectories of these satellites (and of the orbital debris that also permeates this region) via atmospheric drag. Given our increasing dependence on space-based technologies, scientific understanding and prediction of upper atmospheric weather and dynamics is of critical importance.
It is well known that neutral winds impact a large portion of the dynamics within the upper atmosphere and act as a coupling agent between the neutrals and plasma in the ionosphere-thermosphere (I-T) system (e.g., Wang et al., 2021). They are the primary regulators and redistributors of the mass, momentum, and energy within the I-T system and hence play a major role in determining the state of the geospace weather at all latitudes. Neutral winds in the upper atmosphere are driven primarily by a time-varying interplay among heating-induced pressure gradients (caused by absorption of solar ultraviolet irradiance, Joule heating, particle precipitation, and other frictional heating sources), momentum transfer between ion and neutrals, inertial forces (Coriolis and centrifugal), wave/tidal forcing from below, and internal small-scale instabilities. The neutral wind dynamics have a wide spectrum of influences on geospace weather from equatorial to polar latitudes and at all altitudes in mesosphere and thermosphere. Some examples from their wide spectrum of influences are:
• At low latitudes of the E-region, they generate wind dynamo electric fields and push the ionospheric plasma upward and downward along the magnetic field lines, changing the chemical equilibrium of I-T species (e.g., Rishbeth, 1972; Kelly, 1989; Immel et al., 2021).
• In the mesosphere and lower thermosphere (MLT) region, winds impact the energy and momentum deposited by upward propagating waves and tides (e.g., Garcia and Solomon, 1985; Smith, 1996; Forbes, 2007; England, 2012; Yiğit et al., 2016; Sassi et al., 2018).
• At high latitudes, winds feed back into the ionospheric convection and modify Joule heating and other energy deposition processes (e.g., Killeen, 1987; Richmond et al., 2003; Wang et al., 2021).
• Wind circulation cells in the upper atmosphere (forced by waves and tides as well as thermally driven, shallow layered as well as covering wide altitudinal ranges) influence the seasonal variation of chemical species (e.g., Liu, 2007; Qian et al., 2017; Wang et al., 2022).
• Winds influence the evolution of the ionospheric response during a storm and during subsequent relaxation to quiet conditions (e.g., Crowley et al., 1989; Fuller-Rowell et al., 1996; Strickland et al., 1999). The mass, momentum, and energy deposited from the magnetosphere at high latitudes can be transported over the globe by wind-driven transport, which can lead to significant global thermospheric disturbances (e.g., Lu et al., 2016).
• Along with neutral density, neutral winds contribute directly to atmospheric drag and thus play an important role in precision orbit determination and prediction (e.g., Gaposchkin and Coster, 1988; Gaposchkin, 2003). Their accurate determination will also help in accurately predicting the evolution of debris fields.
One of the exciting new paradigms in upper atmospheric science is the recognition that the meteorology of the lower atmosphere continuously imprints on upwardly propagating tidal components and modulates properties of the thermosphere and the ionosphere (e.g., Lieberman et al., 1994; Oberheide and Forbes, 2008; Forbes et al., 2009; Hagan et al., 2009; Häusler and Luhr, 2009; Oberheide et al., 2015). Tides are also perceived as important vertical coupling agents between the MLT region and the ionosphere (e.g., England et al., 2006; Immel et al., 2006; Jin et al., 2012; Pedatella et al., 2012; McDonald et al., 2018). Such driven variability in the entire atmosphere directly impacts winds and can be characterized via analysis of wind observations.
The ionosphere is only
2 Past and present state of neutral wind observations
Several space-based ITM (Ionosphere-Thermosphere-Mesosphere) missions launched in the past (see Figure 1) have improved our understanding of two-dimensional global horizontal neutral wind flows. The AE-E Neutral Atmosphere Temperature (NATE) instrument (Spencer et al., 1973) measured upper thermospheric in-situ cross track winds at lower latitudes; Wind and Temperature Spectrometer (WATS) and Fabry-Perot Interferometer (FPI) on Dynamic Explorer 2 (DE2 1981–1983) (Spencer et al., 1981) focused on the high latitude I-T interactions using in-situ and remote sensing of the region; WIND Imaging Interferometer (WINDII) and High Resolution Doppler Imager (HRDI) on the Upper Atmosphere Research Satellite (UARS 1991–1997) (Hays et al., 1993; Shepherd et al., 1993; 2012) obtained neutral wind profiles using remote sensing equatorward of polar latitudes from the MLT to upper thermospheric altitudes; TIMED Doppler Interferometer (TIDI) on the Thermosphere Ionosphere Mesosphere Energetics and Dynamics (TIMED 2001-present) (Skinner et al., 2003) remotely sensed MLT winds equatorward of auroral latitudes; CHAllenging Minisatellite Payload (CHAMP 2000–2010) (Reigber et al., 2002) and Gravity Field and Steady-State Ocean Circulation Explorer (GOCE 2009–2013) (Doornbos et al., 2013) measured global in-situ cross-track winds in the upper thermosphere; and recently the Michelson Interferometer for Global High-resolution Thermospheric Imaging (MIGHTI 2019-present) (Englert et al., 2017) on the Ionospheric Connection Explorer (ICON) measured neutral winds from the MLT to the upper thermosphere equatorward of middle latitudes. TIDI/TIMED and MIGHTI/ICON are still operational, providing critical pieces of information on the contribution of neutral winds in global atmospheric dynamics from MLT to the upper thermosphere. However, none of these single satellite missions measured global winds synoptically, all have significant altitudinal, latitudinal and local time coverage gaps, and all only resolved one or two components of the 3D vector wind.
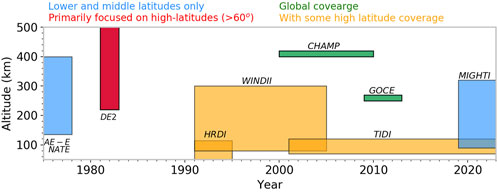
FIGURE 1. The time-line of past ITM missions launched with neutral wind measuring capabilities and their altitudinal coverage. Their latitudinal coverages are indicated with colors on the top.
In addition to the space-based observations which provide complete longitudinal sampling of neutral wind dynamics, ground-based Fabry-Perot Interferometer (FPIs) (Hernandez and Roble, 1976a; Hernandez and Roble, 1976b; Smith et al., 1988; Smith et al., 1989; Makela et al., 2012; Meriwether, 2006) and Scanning Doppler Imaging FPIs (SDIs) (Conde and Smith, 1995; Conde et al., 2018) have contributed extensively to our current understanding of geographically local neutral wind dynamics. They have been operational at multiple sites around the globe since the 1970s. Balloon borne FPI High altitude Interferometer WIND experiment (HIWIND) provided both day and night thermospheric winds in 2011 and 2018 (Wu et al., 2012; 2019). HIWIND daytime capability is significant for understanding thermosphere and ionosphere interaction during the daylight times as most ground-based FPI and SDI techniques are limited to observing during the nighttime. Further, sporadic sounding rocket chemical release wind measurements carried out at different sites around the globe, incoherent scatter radars (ISRs), meteor radars, and lidars have contributed significantly to lower thermospheric wind science.
For more detailed summaries of historical and current neutral wind observations, see, e.g., Dhadly et al. (2017); Dhadly et al. (2018); Dhadly et al. (2019); Drob et al. (2008); Drob et al. (2015); Emmert et al. (2008). Despite the historical space-based and ground-based observational efforts, there still exist wide gaps in the archive of wind measurements, especially in the ignorosphere (see Figure 2) and in the longitudinal coverage at high latitudes, such that the global evolution of neutral wind patterns in response to the solar, magnetospheric, and terrestrial drivers is still poorly understood even after combining four decades of wind measurements.
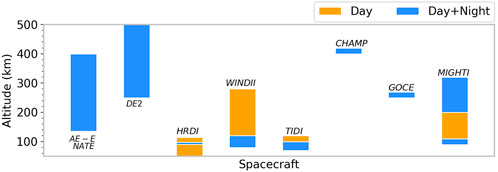
FIGURE 2. Altitudinal and diurnal coverage of the past ITM missions with neutral wind measuring capabilities.
3 Current challenges
Given the important role they play in determining the state of geospace weather, thermospheric winds have remained a subject of interest over the past several decades, but the Heliophysics community still does not have extensive, contemporaneous, and complete databases to close on many fundamental questions. Prior to the UARS and TIMED missions, wind measurements in the thermosphere were obtained primarily from sparsely located ground-based FPIs/SDIs. With exceedingly few exceptions (e.g., Gerrard and Meriwether, 2011), all FPI and SDI ground-based neutral wind measurements are nighttime only because these techniques are overwhelmed during the daytime by Rayleigh-scattered light from the lower atmosphere. In addition, they provide wind measurements at fixed altitudes in E- (∼100–130 km) and F-regions (∼240 km). Thus, the overwhelming majority of daytime MLT and upper thermospheric neutral wind measurements are from space-based instruments, meteor radars, and incoherent scatter radars (ISRs). There have also been rocket-based experiments (e.g., Edwards et al., 1963) that have provided temporally limited measurements of the daytime atmosphere, but by their nature they are unable to provide the continuous global-scale coverage necessary to advance understanding of global dynamics. The ground-based instruments provide limited spatial coverage but at high temporal cadence and some with good local time coverage. These local measurements are significant for investigation of ion/neutral interactions when combined with other observations. Space-based instruments, of course, provide wide geographical coverage, but with limited local time coverage.
Satellites have provided measurements of thermospheric winds at a broad range of thermospheric altitudes. However, the remote sensing of nighttime ignorosphere is very limited (Figure 2). In addition, the ignorosphere is too low to be routinely probed in-situ and too high to be probed with radars, lidars, and balloons. Thus, it has remained extremely under-sampled and poorly understood compared to the other regions. As an example, even diurnal variations of neutral winds in this region are largely undocumented. Unfortunately, the ignorosphere strongly influences the upper thermosphere (
Tides and waves propagating upward from the lower atmosphere (modulated by the meteorology of the lower atmospheric weather) add significant short-term variability in winds and the I-T system which is currently unexplained. For example, major components of the tidal spectrum (migrating tides such as DW1 and SW2 and non-migrating tides such as DE3) originate from the lower atmosphere. In order to estimate their short-term variability in winds, ground-based wind sensors equally spread in longitudes are required, which is hard to achieve due to limited landmass. Single spacecraft missions launched in the past provide only limited local time coverage. Thus, neither the space-based nor ground-based sampling required to understand short-term variability propagating up from the lower atmosphere is currently available. Without sampling multiple local times, especially in the ignorosphere, it is extremely difficult to characterize the vertical coupling in the atmosphere and parse out the exact role of terrestrial weather on the upper atmosphere.
Apart from the ignorosphere, even comprehensive space-time sampling in the regions that can be easily monitored was never obtained in the past. This is because of the multidimensional dependence of neutral wind circulation. A survey showing the geographical sparseness of the winds due to their multi-dimensionality even after fusing 4 decades of data is presented in Figure 3. Some major data gaps that currently exist in neutral wind observations impart large uncertainties in wind characterizations and are known to introduce numerical artifacts in fitting techniques. Some geographical regions are more densely sampled by FPIs and SDIs than others, which can skew statistical analyses or statistical fitting algorithms (e.g., Dhadly et al., 2019) when included with sparsely sampled regions. In addition, optical and in-situ wind sensors operate independently of each other, possess different geometries, different spatial and solar coverage, and may contain large mutual biases (e.g., Dhadly et al., 2019; Dhadly et al., 2021). Fusion of these diverse data in a self-consistent manner to characterize winds is a formidable challenge. Although various investigations have pieced together the patchy historical and current neutral wind observations to build a comprehensive picture of neutral wind dynamics (e.g., Drob et al., 2008; Drob et al., 2015; Emmert et al., 2008; Dhadly et al., 2017; Dhadly et al., 2018; Dhadly et al., 2019), it is still not enough to understand the large-scale evolution of neutral winds in shape and magnitude in response to all the solar and magnetospheric drivers, continuous terrestrial forcing, seasons, and interhemispheric magnetic asymmetry.
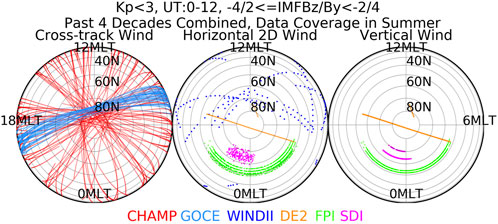
FIGURE 3. A survey of past 4 decades of neutral wind data in the Northern Hemisphere as a function of magnetic latitude and local time (quasi-dipole). Shown are the wind observation locations between 240 and 500 km during summer (May, June, July, and August combined). The geospace conditions are stated on the top and color-coded data sources at the bottom. The most significant coverage is from CHAMP and GOCE which measured only one horizontal wind component (cross-track).
It is worth mentioning that DE-2 was the first ITM mission to measure drivers and impacts of neutral winds simultaneously and provided a tantalizing glimpse of the strongly coupled high latitude I-T system by simultaneously measuring important ionospheric and thermospheric state parameters. Unfortunately, none of the ITM missions after DE-2 measured the drivers and impacts simultaneously especially at high latitudes. The most extreme space weather occurs at these latitudes, and the Heliophysics community does not yet have a complete picture of neutral winds and their drivers.
The basic morphology of horizontal neutral winds and its dominant drivers are known to some extent, thanks to past ITM missions; however, the dynamics of the vertical winds is largely unknown. None of the past ITM missions after DE-2 measured vertical winds. Our current observational understanding of vertical neutral wind dynamics is either based on nighttime local-scale studies by FPIs/SDIs or DE-2. Previous missions such as CHAMP and GOCE measured anomalies in neutral densities in the upper atmosphere. The physical understanding of the thermosphere suggests that they likely caused by neutral wind-driven vertical transport. However, due to a nearly complete lack of vertical wind measurements, their role has yet to be delineated.
Recent advances in physics-based models (e.g., TIEGCM, TIMEGCM, GITM, WACCM-X, CITP-e, etc) and empirical models (e.g., HWM14, HL-TWiM, etc - see reference list) have pushed the boundaries of our current understanding and have shown promising results in capturing the intricacy of the full I-T system. However, due to the lack of appropriate observations, their advances are still to be tested, especially at high latitudes where statistical wind behaviors diverge significantly from physics-based simulations (e.g., Wu et al., 2012; Wu et al., 2015; Liuzzo et al., 2015). If realistic forecasting of space weather is ever to be achieved, the I-T coupling mechanisms need to be understood and validated using observations.
4 Future outlook and recommendations
Given our ever-increasing reliance on space-based technologies, and recognizing the imapcts of neutral winds on I-T dynamics, accurate knowledge of the global neutral wind system, its drivers, and continuous global monitoring is crucial for advancing space weather research and forecasting capabilities needed for space assets. As discussed earlier, the biggest obstacles are current data gaps and observational challenges. The ground-based platforms provide limited geographical coverage (local view) but for extended periods of time and measure the diurnal cycle without any temporal averaging. In contrast, space-based platforms provide wide geographical coverage (synoptic view), but their local time coverage is limited. To overcome the disadvantage of each platform, an effective solution is to use a combination of space-based platforms with the ground-based networks. Thus, investments are needed to develop space-based as well as longitudinally and latitudinally distributed arrays of ground-based sensors. The Super Dual Auroral Radar Network (SuperDARN), which synoptically measures ion velocity fields at high latitudes, has demonstrated the value of ground-based networks to both basic I-T science and space weather research (e.g., Chisham et al., 2007; Nishitani et al., 2019). It’s success derives in large part from international participation by the SuperDARN community.
As discussed earlier, the ignorosphere is the most under-sampled region of the thermosphere. Newer technologies such as THz limb sounder (Wu et al., 2016; Yee et al., 2021) and Doppler Wind and Temperature Sounder (DWTS) (McHugh et al., 2014) have the potential to measure winds along with temperature and density at all local times in the ignorosphere when flown on a space-based mission. For details, please refer to the papers cited above. In addition, optical remote sensing technologies for this region have a strong heritage, at least for daytime conditions. Miniaturized versions of some space-based remote sensing sensors are in development or have been developed and undergoing demonstrations, such as INDI (Interferometer for Neutral-thermosphere Dynamics Imaging (Harlander et al., 2020) and mini-MIGHTI (Harlander et al., 2019; Harlander and Englert, 2020). NASA has recently invested in the ICON/MIGHTI, which is currently monitoring the state of lower latitude neutral winds from MLT to the upper thermosphere from low Earth orbit. However, it is a single-spacecraft mission monitoring only lower latitudes and does not allow us to fully investigate the short-term variability (for example shorter than a month for ICON and 60 days for TIMED) entering the I-T system from the lower atmosphere. A dedicated fleet of multiple identical instruments in polar orbit spread longitudinally would provide an unprecedented sampling of global wind circulation covering a wide range of latitudes/longitudes that is necessary for fundamental advances in our understanding of neutral wind dynamics and its drivers.
The 2013 Decadal Survey on Solar and Space Physics recommended a dedicated platform to address the atmospheric vertical coupling from the MLT to the upper thermosphere, and to study I-T dynamics as a global system using a constellation of satellites in polar orbit spread longitudinally. To fulfill these recommendations, the Decadal Survey endorsed Dynamical Neutral Atmosphere-Ionosphere Coupling (DYNAMIC—a two satellite mission https://soma.larc.nasa.gov/stp/dynamic/index.html) and Geospace Dynamic Constellation (GDC—a six to eight satellite constellation mission—https://lws.larc.nasa.gov/gdc/). The GDC mission, set to launch in 2027 (as per NASA Program Element Appendix on https://lws.larc.nasa.gov/gdc/), will provide a quasi-global coverage of in-situ upper thermospheric winds (∼400 km), initially on local scales and finally on large scales when all the GDC spacecrafts are equally spread in local time and longitudes. DYNAMIC will measure neutral winds at least four local solar times per day (6 h apart if spaced equally in longitude) from the MLT to the upper thermospheric altitudes. DYNAMIC is also being seriously considered by NASA. Flying DYNAMIC and GDC together would amplify the scientific return of each mission - DYNAMIC focusing on the I-T system below 250 km and GDC in-situ above 300 km altitude. These constellations will enable science that goes well beyond what was possible with previous ITM missions. Their combined observations would allow the Heliophysics community to resolve top-down and bottom-up forcing mechanisms which is a difficult observational challenge and is impossible to address without simultaneous multipoint observations by constellation-type missions. In addition to filling data gaps, the data obtained from them would allow the characterization of the multidimensional nature of global neutral winds, which in turn would provide a convenient and reliable reference for the validation and tuning of general circulation models. Figure 4 illustrates the power of having constellation type missions. It shows a survey performed using NASA provided GDC Design Reference Mission (DRM) ephemeris. Assuming the solar cycle 25 after the year 2027 will follow a similar decline as the solar cycle 23 after the year 2004, similar geomagnetic conditions as in 2004–2006 are expected to occur in 2027–2029 (GDC prime mission). For the survey, the geomagnetic conditions of 2004–2006 are utilized as a proxy for 2027–2029. Thus, over the course of the GDC prime mission, it alone will provide much better high latitude coverage of winds than obtained by combining all the available past wind data from all the instruments (as shown in Figure 4).
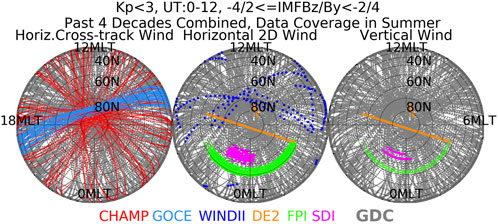
FIGURE 4. Same as Figure 3, but also shows a survey of the projected GDC geographical coverage (grey). For this survey, the geomagnetic conditions of 2004–2006 are utilized as a proxy for 2027–2029 (GDC prime mission). This figure alone illustrates the power of having constellations rather than single spacecraft missions. In data devoid regions, even 3 years of continuous GDC measurements alone will surpass the past 4 decades of wind measurements combined.
Further, FPIs/SDIs are well known for their extensive contributions to our current understanding of neutral wind dynamics, but they provide only nighttime wind measurements (with some exceptions–e.g., Gerrard and Meriwether (2011)). Past experiments have suggested that daytime FPI/SDI observations are feasible; however, they require further development and testing. Successful implementation of ground-based wind instruments that can measure winds at all local times and their employment in longitudinally and latitudinally distributed arrays with international cooperation can provide continuous monitoring of E- and F-region neutral winds. Nevertheless, missions like DYNAMIC are needed to measure vertical profile of neutral winds to understand the lower and upper atmospheric vertical coupling. Thus, a combined approach of using ground-based array and space-based sensor wind measurements would be a huge leap forward for the Heliophysics community.
Author contributions
Primary author—MD All other authors contributed by either editing or helping with data analysis.
Acknowledgments
The authors acknowledge support from NASA, NSF, and the Office of Naval Research.
Conflict of interest
The authors declare that the research was conducted in the absence of any commercial or financial relationships that could be construed as a potential conflict of interest.
Publisher’s note
All claims expressed in this article are solely those of the authors and do not necessarily represent those of their affiliated organizations, or those of the publisher, the editors and the reviewers. Any product that may be evaluated in this article, or claim that may be made by its manufacturer, is not guaranteed or endorsed by the publisher.
References
Chisham, G., Lester, M., Milan, S. E., Freeman, M. P., Bristow, W. A., Grocott, A., et al. (2007). A decade of the super dual auroral radar network (SuperDARN): Scientific achievements, new techniques and future directions. Surv. Geophys. 28, 33–109. doi:10.1007/s10712-007-9017-8
Conde, M. G., Bristow, W. A., Hampton, D. L., and Elliott, J. (2018). Multiinstrument studies of thermospheric weather above Alaska. J. Geophys. Res. Space Phys. 123, 9836–9861. doi:10.1029/2018JA025806
Conde, M., and Smith, R. W. (1995). Mapping thermospheric winds in the auroral zone. Geophys. Res. Lett. 22, 3019–3022. doi:10.1029/95GL02437
Crowley, G., Emery, B. A., Roble, R. G., Carlson, H. C., and Knipp, D. J. (1989). Thermospheric dynamics during september 18-19, 1984: 1. Model simulations. J. Geophys. Res. 94, 16925. doi:10.1029/JA094iA12p16925
Dhadly, M., Emmert, J., Drob, D., Conde, M., Doornbos, E., Shepherd, G., et al. (2018). Seasonal dependence of geomagnetic active-time northern high-latitude upper thermospheric winds. J. Geophys. Res. Space Phys. 123, 739–754. doi:10.1002/2017JA024715
Dhadly, M., Emmert, J., Drob, D., Conde, M., Doornbos, E., Shepherd, G., et al. (2017). Seasonal dependence of northern high-latitude upper thermospheric winds: A quiet time climatological study based on ground-based and space-based measurements. J. Geophys. Res. Space Phys. 122, 2619–2644. doi:10.1002/2016JA023688
Dhadly, M., Emmert, J. T., Drob, D. P., Conde, M. G., Aruliah, A., Doornbos, E., et al. (2019). HL-TWiM empirical model of high-latitude upper thermospheric winds. J. Geophys. Res. Space Phys. 124, 10592–10618. doi:10.1029/2019JA027188
Dhadly, M. S., Englert, C. R., Drob, D. P., Emmert, J. T., Niciejewski, R., and Zawdie, K. A. (2021). Comparison of ICON/MIGHTI and TIMED/TIDI neutral wind measurements in the lower thermosphere. J. Geophys. Res. Space Phys. 126, e2021JA029904. doi:10.1029/2021JA029904
Dhadly, M. S., Sassi, F., Emmert, J. T., Drob, D. P., Conde, M. G., Wu, Q., et al. (2022). “Neutral winds from mesosphere to thermosphere – past, present, and future outlook,” in White paper submitted to Heliophysics decadal survey for solar and space physics (Heliophysics) 2024-2033.
Doornbos, E., Bruinsma, S., Fritsche, B., Visser, P., Van Den Ijssel, J., Encarnacao, J. T., et al. (2013). “Air density and wind retrieval using GOCE data,” in ESA Living Planet Symposium, Proceedings of the conference held on 9-13 September 2013 at Edinburgh in United Kingdom, 7. ESA SP-722. 2-13.
Drob, D. P., Emmert, J. T., Crowley, G., Picone, J. M., Shepherd, G. G., Skinner, W., et al. (2008). An empirical model of the earth’s horizontal wind fields: HWM07. J. Geophys. Res. Space Phys. 113, 1–18. doi:10.1029/2008JA013668
Drob, D. P., Emmert, J. T., Meriwether, J. W., Makela, J. J., Doornbos, E., Conde, M., et al. (2015). An update to the Horizontal Wind Model (HWM): The quiet time thermosphere. Earth Space Sci. 2, 301–319. doi:10.1002/2014EA000089
Edwards, H. D., Cooksey, M. M., Justus, C. G., Fuller, R. N., Albritton, D. L., and Rosenberg, N. W. (1963). Upper-atmosphere wind measurements determined from twelve rocket experiments. J. Geophys. Res. 68, 3021–3032. doi:10.1029/JZ068I010P03021
Emmert, J. T., Dhadly, M. S., and Segerman, A. M. (2021). A globally averaged thermospheric density data set derived from two-line orbital element sets and special perturbations state vectors. J. Geophys. Res. Space Phys. 126, e2021JA029455. doi:10.1029/2021JA029455
Emmert, J. T., Drob, D. P., Shepherd, G. G., Hernandez, G., Jarvis, M. J., Meriwether, J. W., et al. (2008). DWM07 global empirical model of upper thermospheric storm-induced disturbance winds. J. Geophys. Res. 113, A11319. doi:10.1029/2008JA013541
England, S. L. (2012). A review of the effects of non-migrating atmospheric tides on the earth’s low-latitude ionosphere. Space Sci. Rev. 168, 211–236. doi:10.1007/s11214-011-9842-4
England, S. L., Maus, S., Immel, T. J., and Mende, S. B. (2006). Longitudinal variation of the E-region electric fields caused by atmospheric tides. Geophys. Res. Lett. 33, L21105. doi:10.1029/2006GL027465
Englert, C. R., Harlander, J. M., Brown, C. M., Marr, K. D., Miller, I. J., Stump, J. E., et al. (2017). Michelson interferometer for global high-resolution thermospheric imaging (MIGHTI): Instrument Design and calibration. Space Sci. Rev. 212, 553–584. doi:10.1007/s11214-017-0358-4
Forbes, J. M., Bruinsma, S. L., Zhang, X., and Oberheide, J. (2009). Surface-exosphere coupling due to thermal tides. Geophys. Res. Lett. 36, n/a. doi:10.1029/2009gl038748
Forbes, J. M. (2007). Dynamics of the thermosphere. J. Meteorological Soc. Jpn. 85B, 193–213. doi:10.2151/jmsj.85B.193
Fuller-Rowell, T. J., Codrescu, M. V., Rishbeth, H., Moffett, R. J., and Quegan, S. (1996). On the seasonal response of the thermosphere and ionosphere to geomagnetic storms. J. Geophys. Res. 101, 2343–2353. doi:10.1029/95JA01614
Gaposchkin, E. M. (2003). Atmospheric superrotation? Planet. Space Sci. 51, 415–425. doi:10.1016/S0032-0633(03)00021-7
Gaposchkin, E. M., and Coster, A. J. (1988). Analysis of satellite drag. Technical Report. AD-A-203498/1/XAB; JA-6199.
Garcia, R. R., and Solomon, S. (1985). The effect of breaking gravity waves on the dynamics and chemical composition of the mesosphere and lower thermosphere. J. Geophys. Res. 90, 3850–3868. doi:10.1029/JD090ID02P03850
Gerrard, A. J., and Meriwether, J. W. (2011). Initial daytime and nighttime SOFDI observations of thermospheric winds from Fabry-Perot Doppler shift measurements of the 630-nm OI line-shape profile. Ann. Geophys. 29, 1529–1536. doi:10.5194/angeo-29-1529-2011
Hagan, M. E., Maute, A., and Roble, R. G. (2009). Tropospheric tidal effects on the middle and upper atmosphere. J. Geophys. Res. Space Phys. 114, n/a. doi:10.1029/2008JA013637
Harlander, J., Englert, C. R., Harlander, J., and Englert, C. R. (2020). “A small volume sensor for space-based remote sensing of thermospheric neutral winds,” in AGUFM 2020, SA016–0007.
Harlander, J. M., and Englert, C. R. (2020). Laboratory demonstration of mini-MIGHTI: A prototype sensor for thermospheric red-line (630 nm) neutral wind measurements from a 6U CubeSat. J. Atmos. Solar-Terrestrial Phys. 207, 105363. doi:10.1016/J.JASTP.2020.105363
Harlander, J. M., Englert, C. R., and Marr, K. D. (2019). “Mini-mighti: A prototype sensor for thermospheric red-line (630 Nm) neutral wind measurements from A 6u cubesat,” in Optical sensors and sensing congress (ES, FTS, HISE, sensors) (2019), paper FTu4B.3, FTU4B.3. doi:10.1364/fts.2019.ftu4b.3
Häusler, K., and Luhr, H. (2009). Nonmigrating tidal signals in the upper thermospheric zonal wind at equatorial latitudes as observed by CHAMP. Ann. Geophys 27, 2643–2652. doi:10.5194/angeo-27-2643-2009
Hays, P. B., Abreu, V. J., Dobbs, M. E., Gell, D. A., Grassl, H. J., and Skinner, W. R. (1993). The high-resolution Doppler imager on the upper atmosphere research satellite. J. Geophys. Res. 98, 10713. doi:10.1029/93JD00409
Hernandez, G., and Roble, R. G. (1976a). Direct measurements of nighttime thermospheric winds and temperatures, 1. Seasonal variations during geomagnetic quiet periods. J. Geophys. Res. 81, 2065–2074. doi:10.1029/JA081i013p02065
Hernandez, G., and Roble, R. G. (1976b). Direct measurements of nighttime thermospheric winds and temperatures, 2. Geomagnetic storms. J. Geophys. Res. 81, 5173–5181. doi:10.1029/JA081i028p05173
Immel, T. J., Harding, B. J., Heelis, R. A., Maute, A., Forbes, J. M., England, S. L., et al. (2021). Control of ionospheric plasma velocities by thermospheric winds. Nature. doi:10.21203/RS.3.RS-131770/V1
Immel, T. J., Sagawa, E., England, S. L., Henderson, S. B., Hagan, M. E., Mende, S. B., et al. (2006). Control of equatorial ionospheric morphology by atmospheric tides. Geophys. Res. Lett. 33, L15108. doi:10.1029/2006GL026161
Jin, H., Miyoshi, Y., Pancheva, D., Mukhtarov, P., Fujiwara, H., and Shinagawa, H. (2012). Response of migrating tides to the stratospheric sudden warming in 2009 and their effects on the ionosphere studied by a whole atmosphere-ionosphere model GAIA with COSMIC and TIMED/SABER observations. J. Geophys. Res. Space Phys. 117, A10323. doi:10.1029/2012JA017650
M. C. Kelly (Editor) (1989). The Earth’s ionosphere: Plasma physics and electrodynamics (Cambridge, MA, USA: Academic Press), Vol. 43.
Killeen, T. L. (1987). Energetics and dynamics of the Earth’s thermosphere. Rev. Geophys. 25, 433–454. doi:10.1029/rg025i003p00433
Lieberman, R. S., Hays, P. B., Lieberman, R. S., and Hays, P. B. (1994). An estimate of the momentum deposition in the lower thermosphere by the observed diurnal tide. J. Atmos. Sci. 51, 3094–3105. doi:10.1175/1520-0469(1994)051<3094:AEOTMD>2.0.CO;2
Liu, H. L. (2007). On the large wind shear and fast meridional transport above the mesopause. Geophys. Res. Lett. 34. doi:10.1029/2006GL028789
Liu, H. L. (2016). Variability and predictability of the space environment as related to lower atmosphere forcing. Space weather. 14, 634–658. doi:10.1002/2016SW001450
Liuzzo, L. R., Ridley, A. J., Perlongo, N. J., Mitchell, E. J., Conde, M., Hampton, D. L., et al. (2015). High-latitude ionospheric drivers and their effects on wind patterns in the thermosphere. J. Geophys. Res. Space Phys. 120, 715–735. doi:10.1002/2014JA020553
Lu, G., Richmond, A. D., Lühr, H., and Paxton, L. (2016). High-latitude energy input and its impact on the thermosphere. J. Geophys. Res. Space Phys. 121, 7108–7124. doi:10.1002/2015JA022294
Makela, J. J., Meriwether, J. W., Ridley, A. J., Ciocca, M., and Castellez, M. W. (2012). Large-scale measurements of thermospheric dynamics with a multisite Fabry-Perot interferometer network: Overview of plans and results from midlatitude measurements. Int. J. Geophys. 2012, 1–10. doi:10.1155/2012/872140
McDonald, S. E., Sassi, F., Tate, J., McCormack, J. P., Kuhl, D., Drob, D. P., et al. (2018). Impact of non-migrating tides on the low latitude ionosphere during a sudden stratospheric warming event in January 2010. J. Atmos. Solar-Terrestrial Phys. 171, 188–200. doi:10.1016/J.JASTP.2017.09.012
McHugh, M., Fritts, D., and Gordley, L. (2014). The Doppler wind and temperature sounder. Zenodo 9. doi:10.5281/ZENODO.11111
Meriwether, J. W. (2006). Studies of thermospheric dynamics with a fabry-perot interferometer network: A review. J. Atmos. Sol. Terr. Phys. 68, 1576–1589. doi:10.1016/j.jastp.2005.11.014
Nishitani, N., Ruohoniemi, J. M., Lester, M., Baker, J. B. H., Koustov, A. V., Shepherd, S. G., et al. (2019). Review of the accomplishments of mid-latitude super dual auroral radar network (SuperDARN) HF radars. Prog. Earth Planet. Sci. 6, 27. doi:10.1186/s40645-019-0270-5
Oberheide, J., and Forbes, J. M. (2008). Tidal propagation of deep tropical cloud signatures into the thermosphere from TIMED observations. Geophys. Res. Lett. 35, L04816. doi:10.1029/2007GL032397
Oberheide, J., Shiokawa, K., Gurubaran, S., Ward, W. E., Fujiwara, H., Kosch, M. J., et al. (2015). The geospace response to variable inputs from the lower atmosphere: A review of the progress made by task group 4 of CAWSES-II. Prog. Earth Planet. Sci. 2, 2. doi:10.1186/s40645-014-0031-4
Pedatella, N. M., Hagan, M. E., and Maute, A. (2012). The comparative importance ofDE3, SE2, andSPW4 on the generation of wavenumber-4 longitude structures in the low-latitude ionosphere during September equinox. Geophys. Res. Lett. 39, n/a. doi:10.1029/2012gl053643
Qian, L., Burns, A., and Yue, J. (2017). Evidence of the lower thermospheric winter-to-summer circulation from SABER CO2 observations. Geophys. Res. Lett. 44 (10), 100–107. doi:10.1002/2017GL075643
Reigber, C., Lühr, H., and Schwintzer, P. (2002). CHAMP mission status. Adv. Space Res. 30, 129–134. doi:10.1016/S0273-1177(02)00276-4
Richmond, A. D., Lathuillere, C., and Vennerstroem, S. (2003). Winds in the high-latitude lower thermosphere: Dependence on the interplanetary magnetic field. J. Geophys. Res. 108, 1066. doi:10.1029/2002JA009493
Rishbeth, H. (1972). Thermospheric winds and the F-region: A review. J. Atmos. Terr. Phys. 34, 1–47. doi:10.1016/0021-9169(72)90003-7
Sassi, F., McCormack, J. P., and McDonald, S. E. (2019). Whole atmosphere coupling on intraseasonal and interseasonal time scales: A potential source of increased predictive capability. Radio Sci. 54, 913–933. doi:10.1029/2019RS006847
Sassi, F., Siskind, D. E., Tate, J. L., Liu, H. L., and Randall, C. E. (2018). Simulations of the boreal winter upper mesosphere and lower thermosphere with meteorological specifications in SD-WACCM-X. J. Geophys. Res. Atmos. 123, 3791–3811. doi:10.1002/2017JD027782
Shepherd, G. G., Thuillier, G., Gault, W. A., Solheim, B. H., Hersom, C., Alunni, J. M., et al. (1993). WINDII, the wind imaging interferometer on the upper atmosphere research satellite. J. Geophys. Res. 98, 10725. doi:10.1029/93JD00227
Shepherd, G., Thuillier, G., Cho, Y.-M., Duboin, M.-L., Evans, W. F. J., Gault, W. A., et al. (2012). The wind imaging interferometer (WINDII) on the upper atmosphere research satellite: A 20 year perspective. Rev. Geophys. 50. doi:10.1029/2012RG000390
Skinner, W. R., Niciejewski, R. J., Killeen, T. L., Solomon, S. C., Gablehouse, D., Wu, Q., et al. (2003). “Operational performance of the TIMED Doppler interferometer (TIDI),” in Proc. SPIE 5157, optical spectroscopic techniques and instrumentation for atmospheric and space research V (Bellingham, Washington, USA: International Society for Optics and Photonics), Vol. 5157, 47. doi:10.1117/12.503727
Smith, A. K. (1996). Longitudinal variations in mesospheric winds: Evidence for gravity wave filtering by planetary waves. J. Atmos. Sci. 53, 1156–1173. doi:10.1175/1520-0469(1996)053<1156:LVIMWE>2.0.CO;2
Smith, R. W., Meriwether, J. W., Hernandez, G., David, R., Wickwar, V., de la Beaujardiere, O., et al. (1989). Mapping the wind in the polar thermosphere a case study within the CEDAR Program. Eos, Trans. Am. Geophys. Union 70, 161–169. doi:10.1029/89eo00086
Smith, R. W., Rees, D., and Stewart, R. D. (1988). Southern hemisphere thermospheric dynamics: A review. Rev. Geophys. 26, 591. doi:10.1029/RG026i003p00591
Spencer, N. W., Niemann, H. B., and Carignan, G. R. (1973). The neutral-atmosphere temperature instrument. Radio Sci. 8, 287–296. doi:10.1029/RS008I004P00287
Spencer, N. W., Wharton, L. E., Niemann, H. B., Hedin, A. E., Carrignan, G. R., and Maurer, J. C. (1981). The dynamics explorer wind and temperature spectrometer. Space Sci. Instrum. 5, 417–428.
Strickland, D. J., Cox, R. J., Meier, R. R., and Drob, D. P. (1999). Global O/N2 derived from DE 1 FUV dayglow data: Technique and examples from two storm periods. J. Geophys. Res. 104, 4251–4266. doi:10.1029/98JA02817
Wang, N., Qian, L., Yue, J., Wang, W., Mlynczak, M. G., and Russell, J. M. (2022). Climatology of mesosphere and lower thermosphere residual circulations and mesopause height derived from SABER observations. J. Geophys. Res. Atmos. 127, e2021JD035666. doi:10.1029/2021JD035666
Wang, W., Burns, A. G., and Liu, J. (2021). “Upper thermospheric winds,” in Upper atmosphere dynamics and Energetics. chap. Geophysica (Washington, DC, USA: American Geophysical Union), 41–63. doi:10.1002/9781119815631.CH3
Wu, D. L., Yee, J. H., Schlecht, E., Mehdi, I., Siles, J., and Drouin, B. J. (2016). THz limb sounder (TLS) for lower thermospheric wind, oxygen density, and temperature. J. Geophys. Res. Space Phys. 121, 7301–7315. doi:10.1002/2015JA022314
Wu, Q., Emery, B. A., Shepherd, S. G., Ruohoniemi, J. M., Frissell, N. A., and Semeter, J. (2015). High-latitude thermospheric wind observations and simulations with SuperDARN data driven NCAR TIEGCM during the December 2006 magnetic storm. J. Geophys. Res. A Space Phys. 120, 6021–6028. doi:10.1002/2015JA021026
Wu, Q., Knipp, D., Liu, J., Wang, W., Varney, R., Gillies, R., et al. (2019). HIWIND observation of summer season polar cap thermospheric winds. J. Geophys. Res. Space Phys. 124, 9270–9277. doi:10.1029/2019JA027258
Wu, Q., Wang, W., Roble, R. G., Häggström, I., and Strømme, A. (2012). First daytime thermospheric wind observation from a balloon-borne Fabry-Perot interferometer over Kiruna (68N). Geophys. Res. Lett. 39, n/a. doi:10.1029/2012GL052533
Yee, J. H., Mehdi, I., Hayton, D., Siles, J., and Wu, D. (2021). Remote sensing of global lower thermospheric winds. Space Phys. Aeronomy, Up. Atmos. Dyn. Energetics 2021, 469–486. doi:10.1002/9781119815631.CH22
Keywords: neutral wind, upper atmosphere dynamics, thermosphere, ionosphere, ignorosphere, geospace, near earth space, wind dynamics
Citation: Dhadly M, Sassi F, Emmert J, Drob D, Conde M, Wu Q, Makela J, Budzien S and Nicholas A (2023) Neutral winds from mesosphere to thermosphere—past, present, and future outlook. Front. Astron. Space Sci. 9:1050586. doi: 10.3389/fspas.2022.1050586
Received: 21 September 2022; Accepted: 29 December 2022;
Published: 12 January 2023.
Edited by:
Philip J. Erickson, Massachusetts Institute of Technology, United StatesReviewed by:
John Bosco Habarulema, South African National Space Agency, South AfricaLarry Lyons, College of Physical Sciences, University of California, Los Angeles, United States
Ludger Scherliess, Utah State University, United States
Copyright © 2023 Dhadly, Sassi, Emmert, Drob, Conde, Wu, Makela, Budzien and Nicholas. This is an open-access article distributed under the terms of the Creative Commons Attribution License (CC BY). The use, distribution or reproduction in other forums is permitted, provided the original author(s) and the copyright owner(s) are credited and that the original publication in this journal is cited, in accordance with accepted academic practice. No use, distribution or reproduction is permitted which does not comply with these terms.
*Correspondence: Manbharat Dhadly, bWFuYmhhcmF0LmRoYWRseUBucmwubmF2eS5taWw=