- 1U.S. Naval Research Laboratory, Washington, DC, United States
- 2Space Science Laboratory, University of Massachusetts Lowell, Lowell, MA, United States
- 3Planetary Science Institute, Tucson, AZ, United States
The Trans-Coronal Radio Array Fleet (T-CRAF) is a mission concept designed to continuously probe the magnetic field and plasma density structure of the corona at heliocentric distances of ≈ 2 − 10 R⊙ (solar radius, R⊙ = 695, 700 km). T-CRAF consists of thirty small satellites orbiting the Sun-Earth Lagrange Point L3 in order to provide thirty lines of sight (LOS) for ground- or space-based radio propagation studies. T-CRAF is divided into three sets of orbits, each with ten satellites: the first group provides LOS at a solar offset, SO (i.e. closest solar approach) of heliocentric distances 2–4 R⊙ to provide continuous coverage in the middle corona, including initial slow solar wind acceleration; the second group of spacecraft probes the corona at SO = 4–7 R⊙ to cover the region including transition to a supersonic slow solar wind; the outer T-CRAF group is positioned to afford coverage for SO > 7 R⊙ as the winds continue to accelerate towards the Alfvén speed threshold. Each satellite is equipped with a multi-frequency (S-band, C-band, and X-band) linearly polarized transmitter. T-CRAF provides the capability to simultaneously measure the mean values and fluctuations of the magnetic field and plasma density within the solar wind, stream interaction regions, and coronal mass ejections (CMEs). Multiple downlink frequencies provide opportunities to use radio ranging (measurement of group time delay) and apparent-Doppler tracking (measurement of frequency shifts) to infer the plasma density and density gradient along each LOS. Linearly polarized signals provide the ability to detect Faraday rotation (FR) and FR fluctuations, used to infer the magnetic field and field fluctuations along each LOS.
1 Introduction
Understanding the coronal plasma, in particular the plasma density and magnetic field strength and structure, is critical to understanding the foundational physics of the solar wind. Viall and Borovsky (2020) outlines several outstanding questions of solar wind physics that require detailed knowledge of solar wind plasma structure at heliocentric distances of
• The solar wind accelerates from subsonic to supersonic speeds, approaching super-Alfvénic speeds (reaching these speeds at the Alfvén critical point, typically ≲ 20 R⊙, e.g. Wexler et al., 2021a);
• The fast solar wind typically reaches terminal velocity (at
• equatorial and near-equatorial slow wind evolves from dense, closed magnetic structures to more tenuous, open magnetic field structures;
• MHD turbulence can contribute to energization of the corona due to dissipation of Alfvén waves;
• coronal mass ejections (CMEs) erupt, generating rapid evolution of the solar wind’s plasma structure.
With the notable exception of Parker Solar Probe (PSP: Fox et al., 2016), no in situ spacecraft can probe this region of the corona; even PSP only visits perihelion
Data for the coronal magnetic field within this region is much more limited. The most reliable method for obtaining this information is radio remote sensing. For decades, radio remote-sensing methods have been used to probe coronal magnetic fields and, in recent years, some radio methods have even been used to return the internal plasma structure and magnetic field morphology of CMEs. These methods include detecting the gyrosynchrotron emission from the nonthermal particle distribution inside a CME and measuring the circular polarization of moving type IV radio bursts (Gopalswamy and Kundu, 1987; Bastian et al., 2001; Sasikumar Raja et al., 2014; Mondal et al., 2020) as well as measuring Faraday rotation using background linearly polarized radio sources (Bird et al., 1985; Kooi et al., 2014; Kooi et al., 2017; Kooi et al., 2021; Howard et al., 2016; Jensen et al., 2018). All of these methods, though, only provide sparse, sporadic measurements of the coronal or CME magnetic field structure. To answer fundamental questions such as:
• How is the solar wind accelerated?
• What is the origin and evolution of the meso-scale plasma and magnetic field structure of the solar wind?
• What are the dynamics of solar wind turbulence; where is the Alvén critical point and how does it evolve?
• How does the internal magnetic structure of CMEs evolve while they are still in their peak acceleration phase?
continuous, consistent measurements of the coronal magnetic field will be necessary.
2 The trans-coronal radio array fleet mission concept
The Trans-Coronal Radio Array Fleet (T-CRAF) is a mission concept designed to address these issues by continuously probing the magnetic field and plasma density structure of the corona at heliocentric distances of
Nearly 30 years later, the core mission concept—providing background transmitters to continuously probe the solar corona using radio propagation techniques—remains solid. However, several aspects require updating for contemporary planning, namely:
1. Number of spacecraft
2. Heliocentric distances range
3. One or more additional radio sensing frequencies
4. Broad helio-latitudinal coverage
SCS was designed for only two satellites, each completing an orbit in
The T-CRAF mission concept requires thirty spacecraft divided into three sets of orbits, each with ten satellites: the first group provides LOS at SO = 2–4 R⊙; the second group probes the corona at SO = 4–7 R⊙; the outer T-CRAF group is positioned to afford coverage for SO > 7 R⊙. Utilizing
Figure 1 provides an illustration of the apparent spacecraft LOS geometry as viewed from the LASCO coronagraphs on board SOHO, which provides a similar perspective to those of ground-based receivers on Earth. The inner (red) and outer (blue) fields of view are provided by LASCO-C2 and C32. These images show the spectacular CME that erupted on 2000 February 27. If a similar eruption occurred during this mission, the thirty spacecraft in this configuration would ensure multiple LOS through multiple regions of this CME, providing detailed information concerning the CME’s plasma structure and its evolution through the corona. These LOS would also provide critical measurements to evaluate restructuring of the corona after the outburst events.
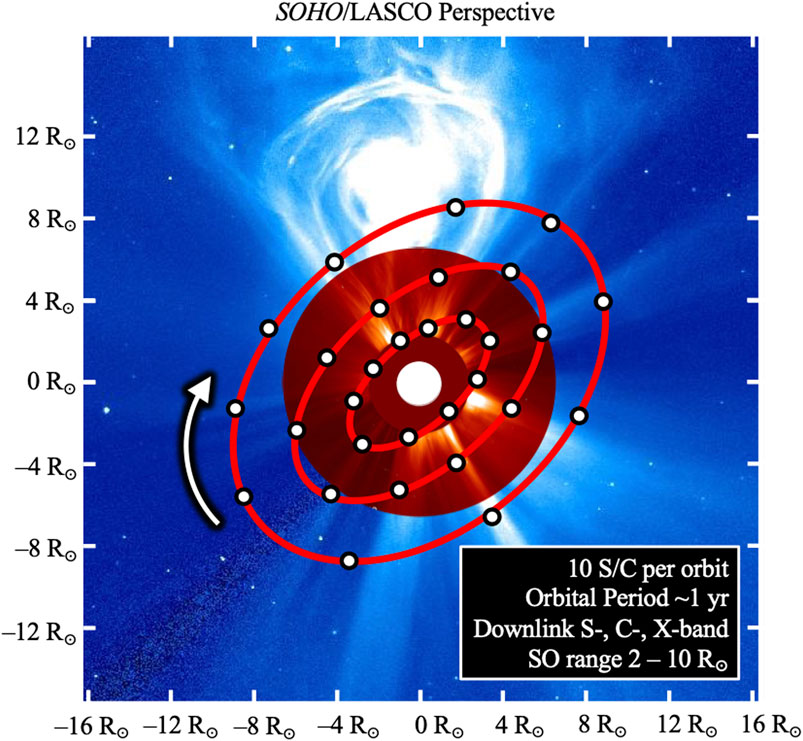
FIGURE 1. Illustration of potential spacecraft (S/C) positions for the Trans-Coronal Radio Array Fleet (T-CRAF) mission concept. There are ten spacecraft (white plotted points) per orbit (red). The inner, middle, and outer orbits have major axis lengths of
3 Radio propagation methods with the trans-coronal radio array fleet
The T-CRAF mission is designed to provide enough background radio transmitters to allow continuous ground- or space-based radio propagation measurements of the corona at distances of

TABLE 1. Radio propagation effects and the corresponding plasma parameters that can be studied using the T-CRAF mission.
3.1 Solar wind speed and acceleration
The solar wind speed, VSW, and acceleration can be calculated using a number of radio propagation methods. Detection of phase (Doppler) shifts using multiple frequencies to infer VSW has been used for decades (see, e.g. Woo, 1978). Observations of radio intensity scintillation can also be used (Imamura et al., 2014). Because T-CRAF uses multiple downlink frequencies, we can use radio frequency fluctuations (FF) to infer VSW. This method, as detailed in Wexler et al. (2019a), Wexler et al. (2020), requires calculating the FF variance,
where λ is the wavelength of the downlink signal, re is the classical electron radius, δne is the RMS electron number density fluctuation, LLOS is the transverse correlation scale of the oscillations, and R is the effective length of the LOS integration path. Calculating the FF variance over a range in heliocentric distances then provides the speed profile necessary to measure acceleration3.
3.2 Solar wind plasma density
T-CRAF’s multiple downlink frequencies provide the opportunity to use radio ranging (measurement of group time delay) and apparent-Doppler tracking (measurement of frequency shift) to infer the plasma density and density gradient along each LOS. These methods provide information for the Total Electron Content, the column density of electrons in the corona:
Radio ranging (also known as Differenced Ranging Versus Integrated Doppler, DRVID) measures the group delay time, Δt, from the spacecraft to the observer. For a signal with angular frequency ω, this is given as
where c is speed of light, qe is electron charge, ϵ0 is permittivity of free space, me is electron mass, and S is the effective length of the LOS. S/c is simply the light travel time in a vacuum; consequently, the effect of the coronal plasma is represented by the second term. T-CRAF will provide multiple downlink frequencies; consequently, the differential time delay, Δτ, between the group delay at one frequency (with ω1) and the group delay at another frequency (with ω2) can give the TEC directly:
For details of this method, see, e.g., Bird et al. (1994). Beyond providing a direct measurement of the TEC, radio ranging can also be used to study fractional electron density fluctuations (see, e.g. Woo et al., 1995).
The apparent-Doppler tracking method provides a means to infer plasma density gradients by measuring the frequency shifts, Δf, related to the rate of change in TEC:
The spacecraft Doppler motion contributes equally to all downlink frequencies; consequently, this can be removed via simple subtraction. The remaining frequency-dependent fluctuations are therefore a result of the plasma. For excellent demonstrations of these two methods, see, e.g., Jensen et al. (2016), Jensen et al. (2018).
3.3 Solar wind magnetic field
T-CRAF’s linearly polarized, multi-frequency downlink signals provide another exciting opportunity: continuous measurement of coronal Faraday rotation (FR). FR is the rotation of the plane of polarization of linearly polarized light as it propagates through a magnetized plasma, denoted by Δχ:
where ne is the electron plasma density, B is the vector magnetic field, and ds is the vector spatial increment along the LOS, in the direction of the observer. The physical constants e, ϵ0, me, and c are the fundamental charge, permittivity of free space, mass of an electron, and speed of light, respectively. The terms in brackets are collectively called the Faraday Rotation Measure, RM, reported in rad m−2.
Numerous FR observations have employed spacecraft transmitters, such as Levy et al. (1969), Stelzried et al. (1970), Volland et al. (1977), Bird (1982), Bird (2007), Pätzold et al. (1987), Bird et al. (1992), Efimov et al. (1993), Andreev et al. (1997), Chashei et al. (1999), Efimov et al. (2000), Jensen et al. (2013), Efimov et al. (2015a), Efimov et al. (2015b), Wexler et al. (2017), Jensen et al. (2018), Wexler et al. (2019b), Wexler et al. (2021a). FR observations using T-CRAF would provide significant improvements over these previous observations:
• T-CRAF provides the opportunity to continuously monitor coronal FR, no waiting for suitable spacecraft positioning (e.g. conjunctions);
• T-CRAF provides thirty LOS, removing uncertainties that result if only a single LOS is available;
• T-CRAF provides multiple downlink frequencies and the opportunity for continuous tracking, which can be used to remove nπ ambiguities in Δχ that result from phase wrapping for large RM;
• T-CRAF provides the opportunity to use radio ranging and apparent-Doppler tracking to infer the plasma density contribution to FR; therefore, the magnetic field inferred from FR will be considerably more accurate.
The first two points are crucial for using FR to probe transient events such as CMEs. All previous observations of CME FR using spacecraft transmitters have been serendipitous. Kooi et al. (2021) reported the only multi-LOS FR observations of a CME that were purposefully triggered based on the sudden eruption of said CME and they accomplished this using background radio galaxies. The primary advantage of natural radio sources (e.g. radio galaxies or pulsars) has always been their availability; on any given day, there are typically 50–100 linearly polarized natural radio sources available for FR experiments. The primary disadvantage of natural radio sources is that they produce a much weaker signal compared to spacecraft transmitters. T-CRAF provides the best of both worlds: continuous availability and strong signals. Kooi et al. (2021) was also the first paper to determine the absolute orientation of a CME’s magnetic field using FR alone4, which required FR observations along multiple LOS. T-CRAF has the potential to provide several LOS through CMEs off the solar limb (e.g. in Figure 1) and, critical for space weather applications, up to thirty LOS for Earth-directed CMEs.
Faraday rotation fluctuations (FRF) can also be measured and have been used to infer magnetic field fluctuations. Simultaneous observations of FRF and variations in TEC (e.g. Hollweg et al., 1982) indicate that magnetic field fluctuations dominate the FRF measurements. Consequently, the term that dominates the variance in RM is given by:
where
This can be used to estimate the RMS magnetic field fluctuations,
where VA is the Alfvén wave speed. This provides a direct method for calculating the wave flux density, which is critical for addressing MHD turbulent dissipation models. For reviews of coronal and CME FR observations, as well as detailed discussion concerning the use of FRF to assess Alfvén wave flux densities or differential FR measurements to detect electric currents on the solar wind, see Bird (2007) and Kooi et al. (2022).
4 The trans-coronal radio array fleet spacecraft concept
Because the objective of T-CRAF’s mission design is to provide enough background radio transmitters to provide continuous ground- or space-based radio propagation measurements of the corona and, therefore, do not require in situ or other remote-sensing instruments on board, the spacecraft themselves can be simple, low-cost radio transmitters. Ideally, each spacecraft would transmit a linearly polarized signal across multiple frequency bands. To optimize the signal-to-noise ratio (SNR), the S-, C-, and X-bands are required. There are three reasons for this selection:
1. Radio propagation effects scale according to frequency (as discussed in Section 3); consequently, multiple downlink frequencies provide a more accurate measurement (especially for FR and apparent-Doppler tracking measurements).
2. SO < 6 R⊙ require higher frequencies like C- and X-bands (e.g. Kooi et al., 2014; Jensen et al., 2018; Wexler et al., 2020) because the side lobes of the receiving antenna’s beam response are smaller and, therefore, less susceptible to solar interference (e.g. solar flares, radio bursts, active regions, the Sun itself, etc.) that would dramatically impact the system noise.
3. At larger solar offsets, solar interference is not necessarily an issue; however, radio propagation effects are reduced due to smaller plasma densities and weaker magnetic fields. Therefore, smaller frequencies (S-band) are required.
5 The trans-coronal radio array fleet’s potential for space weather forecasting
One of the exciting prospects of T-CRAF science is the ability to detect FR through Earth-directed CMEs and, thus, providing detailed measurements of the CME’s magnetic field structure while it is upstream from Earth and well before it interacts with in situ spacecraft orbiting near L1. This poses two significant challenges:
• Earth-directed CMEs may only generate a weak FR signal. Due to the geometry between the CME’s magnetic field and the LOS, and because FR is an integrated measurement, the measured FR may be very weak.
• Modern FR observations of CMEs off the solar limb (which provide a more ideal LOS geometry for FR studies of CMEs, e.g. Kooi et al., 2017; Kooi et al., 2021) typically take weeks to calibrate and analyze; consequently, these observations will not immediately return the CME’s magnetic field structure, which is necessary for early warning space weather applications.
The first issue should be addressed with robust modeling of Earth-directed CME FR. Liu et al. (2007) previously simulated FR for a simple flux rope geometry and Jensen et al. (2010) simulated FR for a flux rope and sheath geometry. These modeling endeavors should be expanded to include modern MHD modeling or geometric reconstructions of CME structures (e.g. Wood et al., 2020), for a variety of CME morphologies and orientations to truly understand the feasibility of these measurements.
The second issue requires development of an advanced CME FR data reduction pipeline. It is only in the last few years that CME FR observations have been able to unabiguously return the CME’s magnetic field orientation (Wood et al., 2020; Kooi et al., 2021). After T-CRAF is launched, it will provide ample opportunities to collect FR observations of Earth-directed CMEs. By building up a database of these measurements and supplementing this with a large sample set of simulated CME FR, machine learning methods can be used to develop this CME FR data reduction pipeline.
Once these issues are addressed, T-CRAF could become a powerful early warning space weather forecasting system using FR tomography. The thirty LOS, together, would provide a low-resolution FR image of the corona: an FR sky map. By taking advantage of simultaneous radio ranging and apparent-Doppler tracking measurements (Section 3.2), the plasma density contribution to the FR will be well known; consequently, each FR sky map could then be used to provide a 2-D representation of the corona’s magnetic field, much in the same way as coronagraph images provide a 2-D representation of the corona’s plasma density. As the Earth rotates around the Sun, the LOS will provide slightly different perspectives of the coronal structures they sample; consequently, an FR sky map time series could be combined using tomographic methods to generate a 3-D representation of the coronal magnetic field. This is similar in principle to using multiple perspectives (e.g. stereoscopic imaging using SOHO/LASCO and STEREO/SECCHI) to reconstruct the 3-D white-light morphology of coronal plasma structures. Because the T-CRAF transmitters would provide a strong downlink signal, they could be used to generate FR sky maps at a high cadence and, therefore, T-CRAF could quickly generate low-resolution FR tomographic scans suitable for studying fast, transient structures like CMEs. This rapid tomography could quickly return the magnetic field orientation of Earth-directed CMEs, which would revolutionize early warning space weather forecasting systems.
Such T-CRAF measurements would be complemented by other future missions, such as the Multiview Observatory for Solar Terrestrial science mission concept (MOST: Gopalswamy et al., 2021). The primary goal of MOST is to understand the magnetic coupling between the solar interior and the heliosphere. The MOST concept includes four spacecraft: one each at L4 and L5, one ahead of L4, and one behind L5. These spacecraft will host a suite of remote-sensing and in situ instruments that will track transient and quiescent 3-D magnetic field structures (e.g. CMEs) within the inner heliosphere. The Faraday Effect Tracker of Coronal and Heliospheric structures (FETCH), one of the proposed instrument concepts for MOST, will perform spacecraft-to-spacecraft FR measurements of the solar wind upstream from Earth at SO < 0.5 AU using the four LOS provided by the four spacecraft (Wexler et al., 2021b; Jensen et al., 2021). FETCH’s LOS will be nearly perpendicular to T-CRAF’s LOS; consequently, the two missions, together, will provide important information about the evolution of solar wind structures upstream from Earth.
6 Recommendations to further develop the the trans-coronal radio array fleet mission concept
The T-CRAF mission concept requires further development. It will be necessary to simulate the T-CRAF orbital dynamics to understand the most cost-effective method to inject each spacecraft into orbit around L3. Pätzold et al. (1996) discuss the orbit design for the two-spacecraft SCS mission concept, noting that their mission concept could be implemented with a tandem launch or with two individual launches directly into the interplanetary trajectory, or via a geocentric transfer (GTO) park orbit. They studied many different transfer orbit options and determined that 2.5- and 3.5-year transfer orbits were most feasible. Similar orbital studies should be made to determine the most efficient launch method for T-CRAF.
After calculating the optimal orbits for T-CRAF, a mission cost analysis must be performed. The primary cost involved for this mission is likely launching the spacecraft fleet and providing each spacecraft with the required propulsion system to enter into orbit, making course corrections as necessary. While other in situ or remote-sensing instruments could, in principle, be added to each spacecraft, the core T-CRAF concept is to only include linearly polarized, multi-frequency radio transmitters. The technology for such downlink transmitters is mature and does not necessarily require additional development; consequently, the spacecraft and radio transmitters themselves may be relatively inexpensive.
It will also be necessary to model the radio propagation effects for both the quiescent solar wind and transient coronal phenomena such as CMEs. The expected values for radio methods (such as those listed in Table 1) should be calculated for different orbital geometries. This is important in order to determine the sensitivity required for receiving systems to effectively use T-CRAF. This would also provide insights into how many spacecraft are actually required to fully reconstruct the three-dimensional magnetic field structure of CMEs (e.g. using tomographic techniques), which is critical if T-CRAF is to be used as part of an early warning geomagnetic storm prediction system. These modeling efforts will also inform the T-CRAF transmitter design. A relatively large transmitter power will be necessary for the downlink signals to be readily detectable by simple ground- or space-based receivers. For transmitters generating a 100 W signal, the SNR is
T-CRAF is designed to provide signals detectable by simple receiving systems; therefore, T-CRAF provides an opportunity to study the corona for a variety of observers, from major research facilities to colleges and universities. However, we also recommend developing a complementary receiving system in tandem with the T-CRAF concept. A dedicated facility with the receivers and resources necessary to continuously detect the downlink signals from all T-CRAF spacecraft will ensure continuous, consistent monitoring of the corona at heliocentric distance of
By making T-CRAF transmitters strong enough to be readily detectable at Earth, though, this mission will be contributing to human-produced radio frequency interference (RFI). In particular, it will place thirty sources of RFI in close proximity to the Sun at frequencies coinciding with the frequency spectrum of some solar radio bursts (SRBs). However, most SRBs emitting at these frequencies are very close to the Sun, typically much closer than the 2 R⊙ solar offsets provided by T-CRAF (e.g. Dulk, 1985). Also, SRBs have well-defined spectral and temporal characteristics, which would be distinguishable from the known downlink signals corresponding to T-CRAF spacecraft. Regardless, it will be important for this mission to engage the solar and radio astronomy communities during the design process to minimize the impact of T-CRAF on other science missions.
7 Summary
The Trans-Coronal Radio Array Fleet’s (T-CRAF) thirty spacecraft provide the opportunity to continuously probe the magnetic field and plasma density structure of the corona at heliocentric distances of
Data availability statement
The original contributions presented in the study are included in the article/supplementary material, further inquiries can be directed to the corresponding author.
Author contributions
JK was responsible for the organization of this article and contributed to all sections. DW was also responsible for the article’s organization and made contributions to Section 2 through Section 4, and Section 6. EJ made contributions to Section 3, Section 5, and Section 6. BW made contributions to Section 5.
Funding
Basic research at the U.S. Naval Research Laboratory (NRL) is supported by 6.1 Base funding.
Acknowledgments
The authors wish to thank M. Bird for making us aware of the original Solar Coronal Sounders mission. The authors are also grateful to Lihua Li for assistance with calculating the T-CRAF antenna transmitter properties. Finally, the authors thank the reviewer for the time and effort put into this article, which improved the overall quality of this work.
Conflict of interest
The authors declare that the research was conducted in the absence of any commercial or financial relationships that could be construed as a potential conflict of interest.
Publisher’s note
All claims expressed in this article are solely those of the authors and do not necessarily represent those of their affiliated organizations, or those of the publisher, the editors and the reviewers. Any product that may be evaluated in this article, or claim that may be made by its manufacturer, is not guaranteed or endorsed by the publisher.
Footnotes
1https://punch.space.swri.edu.
2These images are available at the SOHO public archive http://sohowww.nascom.nasa.gov.
3This requires assumptions for δne. Alternately, if the speed profile is independently known, this can be used to measure δne.
4Wood et al. (2020) previously determined the absolute orientation of a CME by combining radio FR, white-light imaging, and in situ data.
5
References
Andreev, V. E., Efimov, A. I., Samoznaev, L. N., Chashei, I. V., and Bird, M. K. (1997). Characteristics of coronal alfven waves deduced from HELIOS Faraday rotation measurements. Sol. Phys. 176, 387–402. doi:10.1023/A:1004965310604
Bastian, T. S., Pick, M., Kerdraon, A., Maia, D., and Vourlidas, A. (2001). The coronal mass ejection of 1998 april 20: Direct imaging at radio wavelengths. Astrophys. J. 558, L65–L69. doi:10.1086/323421
Bird, M. K., Volland, H., Howard, R. A., Koomen, M. J., Michels, D. J., Sheeley, J., et al. (1985). White light and radio sounding observations of coronal transients. Sol. Phys. 98, 341–368. doi:10.1007/BF00152465
Bird, M. K., Volland, H., Efimov, A. I., Levy, G. S., Seidel, B. L., and Stelzried, C. T. (1992). “Coronal Alfven waves detected by radio sounding during the solar occultations of the HELIOS spacecraft,” in Solar wind seven colloquium. Editors E. Marsch, and R. Schwenn, 147–150.
Bird, M. K., Volland, H., Paetzold, M., Edenhofer, P., Asmar, S. W., and Brenkle, J. P. (1994). The coronal electron density distribution determined from dual-frequency ranging measurements during the 1991 solar conjunction of the ULYSSES spacecraft. Astrophys. J. 426, 373. doi:10.1086/174073
Bird, M. K. (1982). Coronal investigations with occulted spacecraft signals. Space Sci. Rev. 33, 99–126. doi:10.1007/BF00213250
Bird, M. K. (2007). Coronal Faraday rotation of occulted radio signals. Astronomical Astrophysical Trans. 26, 441–453. doi:10.1080/10556790701595236
Brueckner, G. E., Howard, R. A., Koomen, M. J., Korendyke, C. M., Michels, D. J., Moses, J. D., et al. (1995). The large Angle spectroscopic coronagraph (LASCO). Sol. Phys. 162, 357–402. doi:10.1007/BF00733434
Chashei, I. V., Bird, M. K., Efimov, A. I., Andreev, V. E., and Samoznaev, L. N. (1999). Five-minute magnetic field fluctuations in the solar wind acceleration region. Sol. Phys. 189, 399–412. doi:10.1023/A:1005223531849
Domingo, V., Fleck, B., and Poland, A. I. (1995). The SOHO mission: An overview. Sol. Phys. 162, 1–37. doi:10.1007/BF00733425
Dulk, G. A. (1985). Radio emission from the sun and stars. Annu. Rev. Astron. Astrophys. 23, 169–224. doi:10.1146/annurev.aa.23.090185.001125
Efimov, A. I., Chashei, I. V., Shishov, V. I., and Bird, M. K. (1993). Faraday-rotation fluctuations during radio occultation of the circumsolar plasma. Astron. Lett. 19, 57.
Efimov, A. I., Samoznaev, L. N., Andreev, V. E., Chashei, I. V., and Bird, M. K. (2000). Quasi-harmonic faraday-rotation fluctuations of radio waves when sounding the outer solar corona. Astron. Lett. 26, 544–552. doi:10.1134/1.1306991
Efimov, A. I., Lukanina, L. A., Rogashkova, A. I., Samoznaev, L. N., Chashei, I. V., Bird, M. K., et al. (2015a). Coronal radio occultation experiments with the helios solar probes: Correlation/spectral analysis of Faraday rotation fluctuations. Sol. Phys. 290, 2397–2408. doi:10.1007/s11207-015-0687-y
Efimov, A. I., Lukanina, L. A., Rogashkova, A. I., Samoznaev, L. N., Chashei, I. V., Bird, M. K., et al. (2015b). Faraday-rotation fluctuations from radio-sounding measurements of the circumsolar plasma using polarized signals from the HELIOS-1 and HELIOS-2 space probes. Astron. Rep. 59, 313–326. doi:10.1134/S1063772915040022
Fox, N. J., Velli, M. C., Bale, S. D., Decker, R., Driesman, A., Howard, R. A., et al. (2016). The solar probe plus mission: Humanity’s first visit to our star. Space Sci. Rev. 204, 7–48. doi:10.1007/s11214-015-0211-6
Gopalswamy, N., and Kundu, M. R. (1987). Simultaneous radio and white light observations of the 1984 June 27 coronal mass ejection event. Sol. Phys. 114, 347–362. doi:10.1007/BF00167350
Gopalswamy, N., Kucera, T. A., Leake, J. E., MacDowell, R. J., Wilson, L. B., Kanekal, S. G., et al. (2021). “The Multiview observatory for solar terrestrial science (MOST),” in AGU Fall Meeting Conference Abstracts. AGU Fall Meeting Conference Abstracts. SH12A–07.
Grall, R. R., Coles, W. A., Klinglesmith, M. T., Breen, A. R., Williams, P. J. S., Markkanen, J., et al. (1996). Rapid acceleration of the polar solar wind. Nature 379, 429–432. doi:10.1038/379429a0
Hollweg, J. V., Bird, M. K., Volland, H., Edenhofer, P., Stelzried, C. T., and Seidel, B. L. (1982). Possible evidence for coronal Alfven waves. J. Geophys. Res. 87, 1. doi:10.1029/JA087iA01p00001
Hollweg, J. V., Cranmer, S. R., and Chandran, B. D. G. (2010). Coronal Faraday rotation fluctuations and a wave/turbulence-driven model of the solar wind. Astrophys. J. 722, 1495–1503. doi:10.1088/0004-637X/722/2/1495
Howard, R. A., Moses, J. D., Vourlidas, A., Newmark, J. S., Socker, D. G., Plunkett, S. P., et al. (2008). Sun Earth connection coronal and heliospheric investigation (SECCHI). Space Sci. Rev. 136, 67–115. doi:10.1007/s11214-008-9341-4
Howard, T. A., Stovall, K., Dowell, J., Taylor, G. B., and White, S. M. (2016). Measuring the magnetic field of coronal mass ejections near the sun using pulsars. Astrophys. J. 831, 208. doi:10.3847/0004-637X/831/2/208
Imamura, T., Tokumaru, M., Isobe, H., Shiota, D., Ando, H., Miyamoto, M., et al. (2014). Outflow structure of the quiet sun corona probed by spacecraft radio scintillations in strong scattering. Astrophys. J. 788, 117. doi:10.1088/0004-637X/788/2/117
Jensen, E. A., Hick, P. P., Bisi, M. M., Jackson, B. V., Clover, J., and Mulligan, T. (2010). Faraday rotation response to coronal mass ejection structure. Sol. Phys. 265, 31–48. doi:10.1007/s11207-010-9543-2
Jensen, E. A., Bisi, M. M., Breen, A. R., Heiles, C., Minter, T., and Vilas, F. (2013). Measurements of Faraday rotation through the solar corona during the 2009 solar minimum with the MESSENGER spacecraft. Sol. Phys. 285, 83–95. doi:10.1007/s11207-012-0213-4
Jensen, E. A., Frazin, R., Heiles, C., Lamy, P., Llebaria, A., Anderson, J. D., et al. (2016). The comparison of total electron content between radio and thompson scattering. Sol. Phys. 291, 465–485. doi:10.1007/s11207-015-0834-5
Jensen, E. A., Heiles, C., Wexler, D., Kepley, A. A., Kuiper, T., Bisi, M. M., et al. (2018). Plasma interactions with the space environment in the acceleration region: Indications of CME-trailing reconnection regions. Astrophys. J. 861, 118. doi:10.3847/1538-4357/aac5dd
Jensen, E. A., Manchester, W., Fung, S. F., Gopalswamy, N., Jian, L., Kenny, M., et al. (2021). “Novel magnetic field and electron density measurements of CMEs (within 1/2 AU) with the proposed Multiview observatory for solar terrestrial science (MOST) mission,” in AGU Fall Meeting Conference Abstracts. AGU Fall Meeting Conference Abstracts. SH33A–08.
Kaiser, M. L., Kucera, T. A., Davila, J. M., Cyr, St.O. C., Guhathakurta, M., and Christian, E. (2008). The STEREO mission: An introduction. Space Sci. Rev. 136, 5–16. doi:10.1007/s11214-007-9277-0
Kooi, J. E., Fischer, P. D., Buffo, J. J., and Spangler, S. R. (2014). Measurements of coronal Faraday rotation at 4.6 R ⊙. Astrophys. J. 784, 68. doi:10.1088/0004-637X/784/1/68
Kooi, J. E., Fischer, P. D., Buffo, J. J., and Spangler, S. R. (2017). VLA measurements of Faraday rotation through coronal mass ejections. Sol. Phys. 292, 56. doi:10.1007/s11207-017-1074-7
Kooi, J. E., Ascione, M. L., Reyes-Rosa, L. V., Rier, S. K., and Ashas, M. (2021). VLA measurements of Faraday rotation through a coronal mass ejection using multiple lines of sight. Sol. Phys. 296, 11. doi:10.1007/s11207-020-01755-4
Kooi, J. E., Wexler, D. B., Jensen, E. A., Kenny, M. N., Nieves-Chinchilla, T., Wilson, I., et al. (2022). Modern Faraday rotation studies to probe the solar wind. Front. Astron. Space Sci. 9, 841866. doi:10.3389/fspas.2022.841866
Levy, G. S., Sato, T., Seidel, B. L., Stelzried, C. T., Ohlson, J. E., and Rusch, W. V. T. (1969). Pioneer 6: Measurement of transient Faraday rotation phenomena observed during solar occultation. Science 166, 596–598. doi:10.1126/science.166.3905.596
Liu, Y., Manchester, W. B., IV, Kasper, J. C., Richardson, J. D., and Belcher, J. W. (2007). Determining the magnetic field orientation of coronal mass ejections from Faraday rotation. Astrophys. J. 665, 1439–1447. doi:10.1086/520038
Mondal, S., Oberoi, D., and Vourlidas, A. (2020). Estimation of the physical parameters of a CME at high coronal heights using low-frequency radio observations. Astrophys. J. 893, 28. doi:10.3847/1538-4357/ab7fab
Pätzold, M., Bird, M. K., Volland, H., Levy, G. S., Seidel, B. L., and Stelzried, C. T. (1987). The mean coronal magnetic field determined from HELIOS Faraday rotation measurements. Sol. Phys. 109, 91–105. doi:10.1007/BF00167401
Pätzold, M., Neubauer, F. M., and Bird, M. K. (1995). Radio occultation studies with solar corona Sounders. Space Sci. Rev. 72, 77–80. doi:10.1007/BF00768757
Pätzold, M., Neubauer, F. M., Häusler, B., Eidel, W., and Bird, M. K. (1996). Solar corona Sounders: A radio-science mission to the sun. Adv. Space Res. 17, 57–60. doi:10.1016/0273-1177(95)00498-4
Sasikumar Raja, K., Ramesh, R., Hariharan, K., Kathiravan, C., and Wang, T. J. (2014). An estimate of the magnetic field strength associated with a solar coronal mass ejection from low frequency radio observations. Astrophys. J. 796, 56. doi:10.1088/0004-637X/796/1/56
Seaton, D. B., West, M. J., Caspi, A., DeForest, C. E., Golub, L., Mason, J., et al. (2020). “A strategy for a coherent and comprehensive basis for understanding the middle corona,” in Heliophysics 2050 Workshop (Zenodo). [Dataset]. doi:10.5281/zenodo.4025420
Stelzried, C. T., Levy, G. S., Sato, T., Rusch, W. V. T., Ohlson, J. E., Schatten, K. H., et al. (1970). The quasi-stationary coronal magnetic field and electron density as determined from a Faraday rotation experiment. Sol. Phys. 14, 440. doi:10.1007/BF00221330
Viall, N. M., and Borovsky, J. E. (2020). Nine outstanding questions of solar wind physics. J. Geophys. Res. Space Phys. 125, e2018JA026005. doi:10.1029/2018JA026005
Volland, H., Bird, M. K., Levy, G. S., Stelzried, C. T., and Seidel, B. L. (1977). Helios-1 Faraday rotation experiment: Results and interpretations of the solar occultations in 1975. J. Geophys. Zeit. Geophys. 42, 659–672.
West, M. J., Seaton, D. B., Alzate, N., Caspi, A., DeForest, C. E., Gilly, C. R., et al. (2022). “A strategy for a coherent and comprehensive basis for understanding the middle corona,” in Heliophysics 2050: Measurements and Technologies Workshop, 4060.
Wexler, D. B., Jensen, E. A., Hollweg, J. V., Heiles, C., Efimov, A. I., Vierinen, J., et al. (2017). Faraday rotation fluctuations of MESSENGER radio signals through the equatorial lower corona near solar minimum. Space weather. 15, 310–324. doi:10.1002/2016SW001558
Wexler, D. B., Hollweg, J. V., Efimov, A. I., Lukanina, L. A., Coster, A. J., Vierinen, J., et al. (2019a). Spacecraft radio frequency fluctuations in the solar corona: A MESSENGER-HELIOS composite study. Astrophys. J. 871, 202. doi:10.3847/1538-4357/aaf6a8
Wexler, D. B., Hollweg, J. V., Efimov, A. I., Song, P., Jensen, E. A., Lionello, R., et al. (2019b). Radio occultation observations of the solar corona over 1.60-1.86 R⊙: Faraday rotation and frequency shift analysis. J. Geophys. Res. Space Phys. 124, 7761–7777. doi:10.1029/2019JA026937
Wexler, D., Imamura, T., Efimov, A., Song, P., Lukanina, L., Ando, H., et al. (2020). Coronal electron density fluctuations inferred from akatsuki spacecraft radio observations. Sol. Phys. 295, 111. doi:10.1007/s11207-020-01677-1
Wexler, D., Jensen, E. A., Gopalswamy, N., Wilson, L. B., Fung, T., Nieves-Chinchilla, S. F., et al. (2021a). “FETCH concept: Investigating quiescent and transient magnetic structures in the inner Heliosphere using Faraday rotation of spacecraft radio signals,” in AGU Fall Meeting Conference Abstracts. AGU Fall Meeting Conference Abstracts. SH31A–05.
Wexler, D., Stevens, M. L., Case, A. W., and Song, P. (2021b). Alfvén speed transition zone in the solar corona. Astrophys. J. Lett. 919, L33. doi:10.3847/2041-8213/ac25fa
Woo, R., Armstrong, J. W., Bird, M. K., and Patzold, M. (1995). Variation of fractional electron density fluctuations inside 40 Ro observed by Ulysses ranging measurements. Geophys. Res. Lett. 22, 329–332. doi:10.1029/94GL03335
Woo, R. (1978). Radial dependence of solar wind properties deduced from Helios 1/2 and Pioneer 10/11 radio scattering observations. Astrophys. J. 219, 727–739. doi:10.1086/155831
Keywords: solar corona, coronal magnetic fields, coronal plasma density, coronal mass ejection, radio astronomy, polarimetry
Citation: Kooi JE, Wexler DB, Jensen EA and Wood BE (2022) Multipoint radio probe of the solar corona: The trans-coronal radio array fleet. Front. Astron. Space Sci. 9:1026422. doi: 10.3389/fspas.2022.1026422
Received: 23 August 2022; Accepted: 10 October 2022;
Published: 03 November 2022.
Edited by:
Joseph E. Borovsky, Space Science Institute, United StatesReviewed by:
Alexey A. Kuznetsov, Institute of Solar-Terrestrial Physics (RAS), RussiaCopyright © 2022 Kooi, Wexler, Jensen and Wood. This is an open-access article distributed under the terms of the Creative Commons Attribution License (CC BY). The use, distribution or reproduction in other forums is permitted, provided the original author(s) and the copyright owner(s) are credited and that the original publication in this journal is cited, in accordance with accepted academic practice. No use, distribution or reproduction is permitted which does not comply with these terms.
*Correspondence: Jason E. Kooi, jason.kooi@nrl.navy.mil