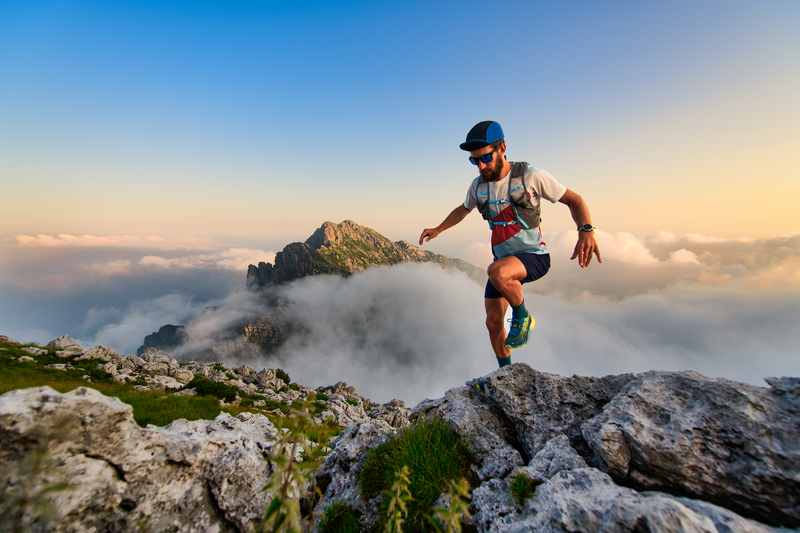
95% of researchers rate our articles as excellent or good
Learn more about the work of our research integrity team to safeguard the quality of each article we publish.
Find out more
MINI REVIEW article
Front. Astron. Space Sci. , 17 September 2021
Sec. Space Physics
Volume 8 - 2021 | https://doi.org/10.3389/fspas.2021.744344
This article is part of the Research Topic Cold-Ion Populations and Cold-Electron Populations in the Earth’s Magnetosphere and Their Impact on the System View all 18 articles
The cold plasmaspheric plasma, the ring current and the radiation belts constitute three important populations of the inner magnetosphere. The overlap region between these populations gives rise to wave-particle interactions between different plasma species and wave modes observed in the magnetosphere, in particular, electromagnetic ion cyclotron (EMIC) waves. These waves can resonantly interact with multiple particle species, being an important loss process for both ring current ions and radiation belt electrons, as well as a cold plasma heating mechanism. This mini-review will focus on the interaction between EMIC waves and cold and thermal plasma, specifically the role of EMIC waves in cold and thermal electron and ion heating. It will discuss early theoretical results in conjunction with numerical modelling and recent satellite observations, and address outstanding problems and controversies in this field.
Electromagnetic ion cyclotron (EMIC) waves are transverse plasma waves generated in the equatorial magnetosphere by ∼10–100 keV ion distributions with temperature anisotropy (Tperp > Tpara, where perpendicular and parallel are defined with respect to the background magnetic field) (Cornwall, 1965). They are typically observed in space and on the ground in the Pc1-2 frequency range between 0.1–5 Hz, though can be observed even at higher frequencies deep in the inner magnetosphere (Sakaguchi et al., 2013). Minor ion species, helium and oxygen, also present in a magnetospheric plasma, produce forbidden band gaps for EMIC wave generation and propagation that split the wave spectrum into multiple branches below the H+, He+, and O+ gyrofrequencies (e.g., Fraser, 1985). For each branch, the wave growth rate and cut-off frequencies are determined by a proton temperature anisotropy, ion composition, and cold plasma density (e.g., Kozyra et al., 1984). Statistical surveys from various satellite missions, covering the whole magnetosphere have reported EMIC wave observations throughout all magnetic local times (MLTs) and L-shells and examined their properties and occurrence distributions depending on geomagnetic conditions and solar wind drivers (Anderson et al., 1992; Denton et al., 2002; Fraser and Nguyen, 2001; Halford et al., 2010; Usanova et al., 2012; Keika et al., 2013; Meredith et al., 2014; Allen et al., 2015; Saikin et al., 2015; Wang et al., 2015; Jun et al., 2019; Grison et al., 2021).
Ions and electrons can resonantly interact with EMIC waves if the Doppler-shifted wave frequency (in the frame of reference of the particle) is equal to an integral multiple of the particle gyrofrequency:
FIGURE 1. Energy exchange between magnetospheric plasma populations via the intermediacy of EMIC waves.
The focus of this mini-review is the energy transfer between EMIC waves and cold and warm plasma populations and the role of EMIC waves in electron and ion heating. For other aspects of EMIC wave generation and the role of cold plasma in EMIC wave-particle interactions please refer to the following recently published relevant reviews. The effects of cold plasma density on EMIC wave growth and its role in energetic particle precipitation is highlighted in Usanova and Mann (2016) and Blum and Breneman (2020). The role of the plasmapause and cold plasma gradients for wave growth and ducting is discussed in Usanova et al. (2016); the review also examined wave characteristics in the source region, and considers the effect of plasma composition on the wave spectrum and propagation to the ground. The contribution of ionospheric ions in wave generation, propagation, and interaction with particles and the importance of cold ion composition measurements for new satellite missions to improve understanding of EMIC waves is addressed in Lee et al. (2021). The general questions of energy coupling between EMIC waves and cold plasma are outlined in Delzanno et al. (2021).
Stable auroral red (SAR) arcs are optical emission at predominately 630 nm observed in the mid-latitude ionosphere during periods of increased magnetic activity (Roach and Roach, 1963; Cornwall et al., 1971; Inaba et al., 2020). The red line corresponds to the excitation of atomic oxygen at ∼400 km altitudes. Despite more than 50 years of research, the generation mechanism for SARs is still controversial and may be caused by the three possible processes: Coulomb collisions of plasmaspheric electrons with ring current ions (Cole, 1965; Kozyra et al., 1987; Fok et al., 1993; Inaba et al., 2020); heating the plasmaspheric electrons via Landau resonance with EMIC waves (Cornwall et al., 1971; Thorne and Horne, 1992; Zhou et al., 2013) and acceleration of plasmaspheric electrons into the ionosphere by kinetic Alfvén waves (Hasegawa and Mima, 1978).
Investigation of the role of EMIC waves in SAR arcs generation stared with an early theoretical work by Cornwall et al. (1971) who proposed the following model. It involves energy transport between anisotropic ring current protons that serve as a source of free energy and excite EMIC waves inside the plasmapause and precipitate while the cyclotron waves are absorbed by plasmaspheric (1–10 eV) electrons. This increases the electron temperature in the direction parallel to the ambient field and leads directly to heat conduction into the ionosphere and drives SARs. The associated proton Coulomb dissipation warms plasmaspheric electrons to temperatures at which electron Landau damping becomes efficient and more prominent than Coulomb scattering. The model predicts that SAR arcs are generated in a narrow region just inside the plasmapause (at L-shells from 2 to 4) that corresponds to their observed latitudinal extent in the ionosphere of several hundred kilometers. However, a later observational study by Kozyra et al. (1987) showed that the energetic, <20 keV O+ ring current species can play a more important role than H+ in the Coulomb scattering and can be responsible for heating of plasmaspheric electrons and SAR formation. Motivated by Kozyra et al. (1987), Thorne and Horne (1992) performed a wave ray tracing in a multicomponent plasma and confirmed that EMIC waves can play an important role in both the energy transfer to plasmaspheric electrons and the subsequent downward heat conduction to SAR arc altitudes. They demonstrated that EMIC waves can experience enhanced path integrated amplification along the steep plasmapause density gradient. Subsequently, when the wave propagation vector becomes highly oblique, absorption occurs during Landau resonance with thermal plasmaspheric electrons which requires an electron temperature above 1 eV. Coulomb scattering by energetic O+ was suggested to act as the primer to heat plasmaspheric electrons for efficient ion-cyclotron wave absorption.
Further, Erlandson et al. (1993) presented simultaneous observations of EMIC waves and subauroral electron temperature enhancements on the polar orbiting DE-2 satellite and confirmed that EMIC waves were responsible for Landau heating the low energy electrons which precipitate to the ionosphere and produce ionospheric temperature enhancements. However, their observations could not pinpoint the heating region location and whether the electrons were heated at the ionospheric altitudes or near the equator. Zhou et al. (2013) observed EMIC waves generated by anisotropic 10–25 keV protons together with electron heating in the equatorial magnetosphere on THEMIS satellites. The observations were combined with calculations of the wave Landau damping rates due to the cold electron gyroresonance with EMIC waves. This work corroborated the original idea of Cornwall et al. (1971) as well as supported the possibility of equatorial electron heating by obliquely propagating EMIC waves.
Recently, Inaba et al. (2020) reported conjugate measurements of a SAR arc observed by an all-sky imager in Finland and the Arase satellite. The Arase observation shows that the SAR arc appeared in the overlap region between a plasmaspheric plume and the ring-current ions and that electromagnetic ion cyclotron waves and kinetic Alfven waves were not observed above the SAR arc. These observations suggest that the heating of plasmaspheric electrons via Coulomb collision with ring-current ions is the most plausible mechanism for the SAR-arc generation. There was still a possibility that due to the measurements at ∼30 degrees off the equator the waves might have reflected above the satellite location along the magnetic field line and therefore were not observed. However, this work strongly implies that the SAR generation mechanism is controversial and warrants further investigation.
The relationship between EMIC waves and electron heating in plasmaspheric plumes was investigated by Yuan et al. (2014). Using in situ Cluster observations, they found that the electron heating was much stronger for field-aligned electrons, consistent with Landau damping predictions. Further, using theoretical calculations of the Landau resonant interaction between electrons and observed EMIC waves, they demonstrated that Landau damping of oblique EMIC waves is a reasonable candidate to heat cold electrons in plasmaspheric plumes.
Note that the studies based on the quasi-linear theory assume that EMIC waves are small in amplitude (in relation to the amplitude of the background magnetic field), experiencing multiple random-phase interactions with electrons which makes this process stochastic or diffusive. Since EMIC waves can exhibit nonlinear features, e.g., consisting of discrete elements that may have rising and falling tones, as well as high amplitudes, non-linear interactions are also necessary to consider (e.g., Nakamura et al., 2016; Shoji et al., 2021). The role of non-linear processes in energy exchange between EMIC waves and cold electrons was addresses by Wang et al. (2019) who conducted test-particle simulations and investigated the role of non-linear Landau resonance. They concluded that the nonlinear wave-particle interactions can occur at typical EMIC wave amplitudes (a few nT in magnetic and a few mV/m in electric field) and may play an important role in EMIC wave damping in the equatorial region, being more prominent than linear Landau damping, especially for obliquely propagating waves.
Satellite measurements from different missions have shown that thermal (∼10s to 100s eV) He+ ions can be resonantly heated by EMIC waves in the direction perpendicular to the background magnetic field (e.g., Mauk et al., 1981; Roux et al., 1982; Mouikis et al., 2002). The role of EMIC waves in cold ion heating has been investigated using both quasi-linear theory and electromagnetic hybrid simulations, where ions are treated kinetically and electrons are treated as conducting fluid. The simulations focused on the self-consistent nonlinear evolution of EMIC waves in plasma consisting of electrons, protons, and He+ and showed that the decrease of initial energetic proton temperature anisotropy results in EMIC wave growth and helium ion heating (Omura et al., 1985; Denton et al., 1993; Gary et al., 1994). Ma et al. (2019) presented Van Allen Probes observations of EMIC waves and He+ and O+ ions and explained the nature of EMIC wave interactions with thermal and energetic ions using a quasi-linear analysis. Their diffusion coefficient calculations indicate that H+-band EMIC waves can heat He+ ions, while He+-band waves can energize O+ ions at thermal energies and pitch angles up to ∼80° through multiple harmonic cyclotron resonances. While thermal ions are heated in the transverse direction, the more energetic ring current ions are precipitated into the upper atmosphere through pitch angle scattering.
Anderson and Fuselier (1994) and Fuselier and Anderson (1996) examined H+ and He+ ion measurements in the 1–160 eV range on AMPTE/CCE to investigate the thermal ion response to the waves. They showed that for protons the perpendicular heating was modest, consistent with a non-resonant interaction near the equator. By contrast, He+ energization was ∼20 times stronger and peaked at pitch angles intermediate between field-aligned and perpendicular directions, consistent with a gyroresonant interaction off the equator. Omidi et al. (2010) and Bortnik et al. (2010) further examined the nonlinear evolution of EMIC waves using 2.5D hybrid simulations along with detailed test particle calculations. They found that the nonlinear evolution of EMIC waves involves generation of electrostatic waves with a wavelength half of that of the ion cyclotron waves and also results in parallel heating of cold He+ and H+ ions for substantially long periods.
The non-linear wave interactions with ions were recently investigated using high-resolution ion measurements on MMS spacecraft. Kitamura et al. (2018) presented the first observational evidence of energy transfer from energetic ring current protons to cold helium ions via EMIC wave-particle interactions confirming earlier simulation results. The wave-ion phase relations demonstrated that a cyclotron resonance transferred energy from 14–30 keV protons to waves, which in turn non-resonantly accelerated cold He+ to energies up to ∼2 keV. Further, utilizing the same instrumentation and technique as in Kitamura et al. (2018), Abid et al. (2021) showed that 1–100 eV protons can also be non-linearly energized by EMIC waves through phase bunching.
Over the recent several decades of space exploration, significant progress has been made in understanding of energy transfer processes in the magnetosphere and the coupling between different plasma populations within this complex system. EMIC waves have been shown to act as an intermediary that couples energy and momentum between different energy magnetospheric plasma populations via various resonance mechanisms. High-resolution satellite observations, theory and self-consistent simulations have answered a lot of questions regarding the role of EMIC waves in Landau heating of plasmaspheric electrons and heavy ions. Despite the long history of research in this area, there are still open questions, for example, the role of EMIC waves in red auroral arc generation and the location of regions where the energy transfer predominately takes place. Recent studies have also underlined the significance of nonlinear processes in EMIC wave-particle interactions and placed emphasis on the potential to include those in global magnetospheric models which will be a next critical step towards predictive modeling.
MU is the only contributing author for this review.
The work on this review was supported by NASA Awards 80 NSSC19K0265 and NAS5-01072.
The author declares that the research was conducted in the absence of any commercial or financial relationships that could be construed as a potential conflict of interest.
All claims expressed in this article are solely those of the authors and do not necessarily represent those of their affiliated organizations, or those of the publisher, the editors and the reviewers. Any product that may be evaluated in this article, or claim that may be made by its manufacturer, is not guaranteed or endorsed by the publisher.
Abid, A. A., Lu, Q., Gao, X. L., Alotaibi, B. M., Ali, S., Qureshi, M. N. S., et al. (2021). Energization of Cold Ions by Electromagnetic Ion Cyclotron Waves: Magnetospheric Multiscale (MMS) Observations. Phys. Plasmas 28, 072901. doi:10.1063/5.0046764
Allen, R. C., Zhang, J. C., Kistler, L. M., Spence, H. E., Lin, R. L., Klecker, B., et al. (2015). A Statistical Study of EMIC Waves Observed by Cluster: 1. Wave Properties. J. Geophys. Res. Space Phys. 120, 5574–5592. doi:10.1002/2015JA021333
Anderson, B. J., and Fuselier, S. A. (1994). Response of thermal Ions to Electromagnetic Ion Cyclotron Waves. J. Geophys. Res. 99 (A10), 19,413–19,425. doi:10.1029/94JA01235
Anderson, B. J., Erlandson, R. E., and Zanetti, L. J. (1992). A Statistical Study of Pc 1-2 Magnetic Pulsations in the Equatorial Magnetosphere: 1. Equatorial Occurrence Distributions. J. Geophys. Res. 97 (A3), 3075–3088. doi:10.1029/91JA02706
Blum, L. W., and Breneman, A. W. (2020). “Observations of Radiation belt Losses Due to Cyclotron Wave-Particle Interactions,” in The Dynamic Loss of Earth’s Radiation Belts. Editors A.N. Jaynes, and M.E. Usanova (Elsevier) chap. 3, 49–98. doi:10.1016/B978-0-12-813371-2.00003-2 Available at: https://www.sciencedirect.com/science/article/pii/B9780128133712000032
Blum, L. W., Halford, A., Millan, R., Bonnell, J. W., Goldstein, J., Usanova, M., et al. (2015). Observations of Coincident EMIC Wave Activity and Duskside Energetic Electron Precipitation on 18-19 January 2013. Geophys. Res. Lett. 42, 5727–5735. doi:10.1002/2015GL065245
Blum, L. W., Artemyev, A., Agapitov, O., Mourenas, D., Boardsen, S., and Schiller, Q. (2019). EMIC Wave‐Driven Bounce Resonance Scattering of Energetic Electrons in the Inner Magnetosphere. J. Geophys. Res. Space Phys. 124, 2484–2496. doi:10.1029/2018JA026427
Bortnik, J., Thorne, R. M., and Omidi, N. (2010). Nonlinear Evolution of EMIC Waves in a Uniform Magnetic Field: 2. Test-Particle Scattering. J. Geophys. Res. 115, 741–744. doi:10.1029/2010JA015603
Cao, X., Ni, B., Summers, D., Bortnik, J., Tao, X., Shprits, Y. Y., et al. (2017). Bounce Resonance Scattering of Radiation belt Electrons by H + Band EMIC Waves. J. Geophys. Res. Space Phys. 122, 1702–1713. doi:10.1002/2016JA023607
Capannolo, L., Li, W., Spence, H., Johnson, A. T., Shumko, M., Sample, J., et al. (2021). Energetic Electron Precipitation Observed by FIREBIRD‐II Potentially Driven by EMIC Waves: Location, Extent, and Energy Range from a Multievent Analysis. Geophys. Res. Lett. 48, e2020GL091564. doi:10.1029/2020GL091564
Cole, K. D. (1965). Stable Auroral Red Arcs, Sinks for Energy of Dst main Phase. J. Geophys. Res. 70 (7), 1689–1706. doi:10.1029/JZ070i007p01689
Cornwall, J. M., Coroniti, F. V., and Thorne, R. M. (1971). Unified Theory of SAR Arc Formation at the Plasmapause. J. Geophys. Res. 76 (19), 4428–4445. doi:10.1029/JA076i019p04428
Cornwall, J. M. (1965). Cyclotron Instabilities and Electromagnetic Emission in the Ultra Low Frequency and Very Low Frequency Ranges. J. Geophys. Res. 70 (1), 61–69. doi:10.1029/JZ070i001p00061
Delzanno, G. L., Borovsky, J. E., Henderson, M. G., Resendiz Lira, P. A., Roytershteyn, V., and Welling, D. T. (2021). The Impact of Cold Electrons and Cold Ions in Magnetospheric Physics. J. Atmos. Solar-Terr. Phys. 220, 105599. doi:10.1016/j.jastp.2021.105599
Denton, R. E., Hudson, M. K., Fuselier, S. A., and Anderson, B. J. (1993). Electromagnetic Ion Cyclotron Waves in the Plasma Depletion Layer. J. Geophys. Res. 98, 13,477–13,490. doi:10.1029/93JA00796
Denton, R. E., LaBelle, J., and Zhu, X. (2002). Location of Pc 1-2 Waves Relative to the Magnetopause. Ann. Geophys. 20, 1763–1767. doi:10.5194/angeo‐20‐1763‐2002
Erlandson, R. E., Aggson, T. L., Hogey, W. R., and Slavin, J. A. (1993). Simultaneous Observations of Subauroral Electron Temperature Enhancements and Electromagnetic Ion Cyclotron Waves. Geophys. Res. Lett. 20, 1723–1726. doi:10.1029/93gl01975
Fok, M.-C., Kozyra, J. U., Nagy, A. F., Rasmussen, C. E., and Khazanov, G. V. (1993). Decay of Equatorial Ring Current Ions and Associated Aeronomical Consequences. J. Geophys. Res. 98 (A11), 19381–19393. doi:10.1029/93JA01848
Fraser, B. J., and Nguyen, T. S. (2001). Is the Plasmapause a Preferred Source Region of Electromagnetic Ion Cyclotron Waves in the Magnetosphere? J. Atmos. Solar-Terr. Phys. 63, 1225–1247. doi:10.1016/S1364‐6826(00)00225‐X
Fraser, B. J. (1985). Observations of Ion Cyclotron Waves Near Synchronous Orbit and on the Ground. Space Sci. Rev. 42, 357–374. doi:10.1007/BF0021499310
Fu, S., Ni, B., Lou, Y., Bortnik, J., Ge, Y., Tao, X., et al. (2018). Resonant Scattering of Near‐Equatorially Mirroring Electrons by Landau Resonance with H + Band EMIC Waves. Geophys. Res. Lett. 45, 10,866–10,873. doi:10.1029/2018GL079718
Fuselier, S. A., and Anderson, B. J. (1996). Low-energy He+and H+distributions and Proton Cyclotron Waves in the Afternoon Equatorial Magnetosphere. J. Geophys. Res. 101 (A6), 13255–13265. doi:10.1029/96JA00292
Gary, S. P., McKean, M. E., Winske, D., Anderson, B. J., Denton, R. E., and Fuselier, S. A. (1994). The Proton Cyclotron Instability and the Anisotropy/β Inverse Correlation. J. Geophys. Res. 99 (A4), 5903–5914. doi:10.1029/93JA03583
Grison, B., Santolík, O., Lukačevič, J., and Usanova, M. E. (2021). Occurrence of EMIC Waves in the Magnetosphere According to Their Distance to the Magnetopause. Geophys. Res. Lett. 48, e2020GL090921. doi:10.1029/2020gl090921
Halford, A. J., Fraser, B. J., and Morley, S. K. (2010). EMIC Wave Activity during Geomagnetic Storm and Nonstorm Periods: CRRES Results. J. Geophys. Res. 115, a–n. doi:10.1029/2010JA015716
Hasegawa, A., and Mima, K. (1978). Anomalous Transport Produced by Kinetic Alfvén Wave Turbulence. J. Geophys. Res. 83 (A3), 1117–1123. doi:10.1029/JA083iA03p01117
Horne, R. B., and Thorne, R. M. (1998). Potential Waves for Relativistic Electron Scattering and Stochastic Acceleration during Magnetic Storms. Geophys. Res. Lett. 25, 3011–3014. doi:10.1029/98GL01002
Inaba, Y., Shiokawa, K., Oyama, S. i., Otsuka, Y., Oksanen, A., Shinbori, A., et al. (2020). Plasma and Field Observations in the Magnetospheric Source Region of a Stable Auroral Red (SAR) Arc by the Arase Satellite on 28 March 2017. J. Geophys. Res. Space Phys. 125, e2020JA028068. doi:10.1029/2020JA028068
Jun, C. W., Yue, C., Bortnik, J., Lyons, L. R., Nishimura, Y., Kletzing, C., et al. (2019). A Statistical Study of EMIC Waves Associated with and without Energetic Particle Injection from the Magnetotail. J. Geophys. Res. Space Phys. 124, 433–450. doi:10.1029/2018JA025886
Keika, K., Takahashi, K., Ukhorskiy, A. Y., and Miyoshi, Y. (2013). Global Characteristics of Electromagnetic Ion Cyclotron Waves: Occurrence Rate and its Storm Dependence. J. Geophys. Res. Space Phys. 118, 4135–4150. doi:10.1002/jgra.50385
Kitamura, N., Kitahara, M., Shoji, M., Miyoshi, Y., Hasegawa, H., Nakamura, S., et al. (2018). Direct Measurements of Two-Way Wave-Particle Energy Transfer in a Collisionless Space Plasma. Science 361, 1000–1003. doi:10.1126/science.aap8730
Kozyra, J. U., Cravens, T. E., Nagy, A. F., Fontheim, E. G., and Ong, R. S. B. (1984). Effects of Energetic Heavy Ions on Electromagnetic Ion Cyclotron Wave Generation in the Plasmapause Region. J. Geophys. Res. 89 (A4), 2217–2233. doi:10.1029/JA089iA04p02217
Kozyra, J. U., Shelley, E. G., Comfort, R. H., Brace, L. H., Cravens, T. E., and Nagy, A. F. (1987). The Role of Ring Current O+ in the Formation of Stable Auroral Red Arcs. J. Geophys. Res. 92, 7487. doi:10.1029/JA092iA07p07487
Lee, J. H., Blum, L. W., and Chen, L. (2021). On the Impacts of Cold Plasma and Ion Composition on Magnetospheric EMIC Waves. Front. Astron. Space Sci. 28 July 2021. doi:10.3389/fspas.2021.719715
Ma, Q., Li, W., Yue, C., Thorne, R. M., Bortnik, J., Kletzing, C. A., et al. (2019). Ion Heating by Electromagnetic Ion Cyclotron Waves and Magnetosonic Waves in the Earth's Inner Magnetosphere. Geophys. Res. Lett. 46, 6258–6267. doi:10.1029/2019GL083513
Mauk, B. H., McIlwain, C. E., and McPherron, R. L. (1981). Helium Cyclotron Resonance within the Earth's Magnetosphere. Geophys. Res. Lett. 8 (1), 103–106. doi:10.1029/GL008i001p00103
Meredith, N. P., Horne, R. B., Kersten, T., Fraser, B. J., and Grew, R. S. (2014). Global Morphology and Spectral Properties of EMIC Waves Derived from CRRES Observations. J. Geophys. Res. Space Phys. 119, 5328–5342. doi:10.1002/2014JA020064
Mouikis, C. G., Kistler, L. M., Baumjohann, W., Lund, E. J., Korth, A., Klecker, B., et al. (2002). Equator-S Observations of He+ energization by EMIC Waves in the Dawnside Equatorial Magnetosphere. Geophys. Res. Lett. 29 (10), 74–81. doi:10.1029/2001GL013899
Nakamura, S., Omura, Y., and Angelopoulos, V. (2016). A Statistical Study of EMIC Rising and Falling Tone Emissions Observed by THEMIS. J. Geophys. Res. Space Phys. 121, 8374–8391. doi:10.1002/2016JA022353
Omidi, N., Thorne, R. M., and Bortnik, J. (2010). Nonlinear Evolution of EMIC Waves in a Uniform Magnetic Field: 1. Hybrid Simulations. J. Geophys. Res. 115, 1–11. doi:10.1029/2010JA015607
Omura, Y., Ashour-Abdalla, M., Gendrin, R., and Quest, K. (1985). Heating of thermal Helium in the Equatorial Magnetosphere: A Simulation Study. J. Geophys. Res. 90, 8281–8292. doi:10.1029/JA090iA09p08281
Roach, F. E., and Roach, J. R. (1963). Stable 6300 Å Auroral Arcs in Mid-latitudes. Planet. Space Sci. 11, 523–540. doi:10.1016/0032-0633(63)90076-X
Roux, A., Perraut, S., Rauch, J. L., de Villedary, C., Kremser, G., Korth, A., et al. (1982). Wave-particle Interactions Near ΩHe+ observed on Board GEOS 1 and 2: 2. Generation of Ion Cyclotron Waves and Heating of He+ ions. J. Geophys. Res. 87 (A10), 8174–8190. doi:10.1029/JA087iA10p08174
Saikin, A. A., Zhang, J. C., Allen, R. C., Smith, C. W., Kistler, L. M., Spence, H. E., et al. (2015). The Occurrence and Wave Properties of H + ‐, He + ‐, and O + ‐band EMIC Waves Observed by the Van Allen Probes. J. Geophys. Res. Space Phys. 120, 7477–7492. doi:10.1002/2015JA021358
Sakaguchi, K., Kasahara, Y., Shoji, M., Omura, Y., Miyoshi, Y., Nagatsuma, T., et al. (2013). Akebono Observations of EMIC Waves in the Slot Region of the Radiation Belts. Geophys. Res. Lett. 40, 5587–5591. doi:10.1002/2013GL058258
Schulz, M., and Lanzerotti, L. J. (1974). Particle Diffusion in the Radiation Belts. New York: Springer. doi:10.1007/978-3-642-65675-0
Shoji, M., Miyoshi, Y., Kistler, L. M., Asamura, K., Matsuoka, A., Kasaba, Y., et al. (2021). Discovery of Proton hill in the Phase Space during Interactions between Ions and Electromagnetic Ion Cyclotron Waves. Sci. Rep. 11, 13480. doi:10.1038/s41598-021-92541-0
Shprits, Y. Y. (2009). Potential Waves for Pitch-Angle Scattering of Near-Equatorially Mirroring Energetic Electrons Due to the Violation of the Second Adiabatic Invariant. Geophys. Res. Lett. 36, L12106. doi:10.1029/2009GL038322
Søraas, F., Laundal, K. M., and Usanova, M. (2013). Coincident Particle and Optical Observations of Nightside Subauroral Proton Precipitation. J. Geophys. Res. Space Phys. 118, 1112–1122. doi:10.1002/jgra.50172
Summers, D., Ni, B., and Meredith, N. P. (2007). Timescales for Radiation belt Electron Acceleration and Loss Due to Resonant Wave-Particle Interactions: 2. Evaluation for VLF Chorus, ELF Hiss, and Electromagnetic Ion Cyclotron Waves. J. Geophys. Res. 112, a–n. doi:10.1029/2006JA011993
Thorne, R. M., and Horne, R. B. (1992). The Contribution of Ion-Cyclotron Waves to Electron Heating and SAR-Arc Excitation Near the Storm-Time Plasmapause. Geophys. Res. Lett. 19, 417–420. doi:10.1029/92GL00089
Usanova, M. E., and Mann, I. R. (2016). “Understanding the Role of EMIC Waves in Radiation Belt and Ring Current Dynamics: Recent Advances,” in Waves, Particles, and Storms in Geospace: A Complex Interplay. Editors G. Balasis, I. A. Daglis, and I. R. Mann (Oxford University Press) chap. 10, 1–13. doi:10.1093/acprof:oso/9780198705246.001.0001
Usanova, M. E., Mann, I. R., Kale, Z. C., Rae, I. J., Sydora, R. D., Sandanger, M., et al. (2010). Conjugate Ground and Multisatellite Observations of Compression-Related EMIC Pc1 Waves and Associated Proton Precipitation. J. Geophys. Res. 115, A07208. doi:10.1029/2009JA014935
Usanova, M. E., Mann, I. R., Bortnik, J., Shao, L., and Angelopoulos, V. (2012). THEMIS Observations of Electromagnetic Ion Cyclotron Wave Occurrence: Dependence on AE, SYMH, and Solar Wind Dynamic Pressure. J. Geophys. Res. 117. doi:10.1029/2012JA018049
Usanova, M. E., Mann, I. R., and Darrouzet, F. (2016). “EMIC Waves in the Inner Magnetosphere,” in Low-Frequency Waves in Space Plasmas. Editors A. Keiling, D.-H. Lee, and V. Nakariakov (Hoboken, NJ: John Wiley & Sons), 65–78. doi:10.1002/9781119055006.ch5
Wang, D., Yuan, Z., Yu, X., Deng, X., Zhou, M., Huang, S., et al. (2015). Statistical Characteristics of EMIC Waves: Van Allen Probe Observations. J. Geophys. Res. Space Phys. 120, 4400–4408. doi:10.1002/2015JA021089
Wang, B., Su, Z., Zhang, Y., Shi, S., and Wang, G. (2016). Nonlinear Landau Resonant Scattering of Near Equatorially Mirroring Radiation belt Electrons by Oblique EMIC Waves. Geophys. Res. Lett. 43, 3628–3636. doi:10.1002/2016GL068467
Wang, B., Li, P., Huang, J., and Zhang, B. (2019). Nonlinear Landau Resonance between EMIC Waves and Cold Electrons in the Inner Magnetosphere. Phys. Plasmas 26, 042903. doi:10.1063/1.5088374
Yahnin, A. G., Popova, T. A., Demekhov, A. G., Lubchich, A. A., Matsuoka, A., Asamura, K., et al. (2021). Evening Side EMIC Waves and Related Proton Precipitation Induced by a Substorm. J. Geophys. Res. Space Phys. 126, e2020JA029091. doi:10.1029/2020JA029091
Yuan, Z., Xiong, Y., Huang, S., Deng, X., Pang, Y., Zhou, M., et al. (2014). Cold Electron Heating by EMIC Waves in the Plasmaspheric Plume with Observations of the Cluster Satellite. Geophys. Res. Lett. 41, 1830–1837. doi:10.1002/2014GL059241
Keywords: EMIC waves, cold plasma, energy exchange, Landau heating, plasmasphere
Citation: Usanova ME (2021) Energy Exchange Between Electromagnetic Ion Cyclotron (EMIC) Waves and Thermal Plasma: From Theory to Observations. Front. Astron. Space Sci. 8:744344. doi: 10.3389/fspas.2021.744344
Received: 20 July 2021; Accepted: 30 August 2021;
Published: 17 September 2021.
Edited by:
Cecilia Norgren, University of Bergen, NorwayReviewed by:
Binbin Ni, Wuhan University, ChinaCopyright © 2021 Usanova. This is an open-access article distributed under the terms of the Creative Commons Attribution License (CC BY). The use, distribution or reproduction in other forums is permitted, provided the original author(s) and the copyright owner(s) are credited and that the original publication in this journal is cited, in accordance with accepted academic practice. No use, distribution or reproduction is permitted which does not comply with these terms.
*Correspondence: M. E. Usanova, bWFyaWEudXNhbm92YUBsYXNwLmNvbG9yYWRvLmVkdQ==
Disclaimer: All claims expressed in this article are solely those of the authors and do not necessarily represent those of their affiliated organizations, or those of the publisher, the editors and the reviewers. Any product that may be evaluated in this article or claim that may be made by its manufacturer is not guaranteed or endorsed by the publisher.
Research integrity at Frontiers
Learn more about the work of our research integrity team to safeguard the quality of each article we publish.