- 1Department of Civil and Environmental Engineering, Stanford University, Stanford, CA, United States
- 2Center for the Utilization of Biological Engineering in Space, Berkeley, CA, United States
Various microbial systems have been explored for their applicability to in-situ resource utilisation (ISRU) on Mars and suitability to leverage Martian resources and convert them into useful chemical products. Considering only fully bio-based solutions, two approaches can be distinguished, which comes down to the form of carbon that is being utilized: (a) the deployment of specialised species that can directly convert inorganic carbon (atmospheric CO2) into a target compound or (b) a two-step process that relies on independent fixation of carbon and the subsequent conversion of biomass and/or complex substrates into a target compound. Due to the great variety of microbial metabolism, especially in conjunction with chemical support-processes, a definite classification is often difficult. This can be expanded to the forms of nitrogen and energy that are available as input for a biomanufacturing platform. To provide a perspective on microbial cell factories that may be suitable for Space Systems Bioengineering, a high-level comparison of different approaches is conducted, specifically regarding advantages that may help to extend an early human foothold on the red planet.
Introduction
A multitude of different bioregenerative life-support systems, have been proposed for in-situ resource utilization in Space and at destinations across the Solar System, such as Luna and Mars. Approaches draw from all domains of life, employing microbes as well as higher organisms, with applications ranging from production and recovery of resources, (e.g. generation of oxygen, food and materials, biomining, wastewater recycling), to providing shelter and protection, as far as generation of energy and even terraforming (Kalkus et al., 2018; Llorente et al., 2018; Hastings and Nangle, 2019; Lopez et al., 2019; Shunk et al., 2020; Volger et al., 2020). Here, the focus is on the deployment of microorganisms in bioreactors for bioproduction of feedstocks for consumables and durable goods to sustain and extend an initial presence on Mars. Special attention is given to the disruptive impact of Synthetic Biology on advancing these capabilities (Menezes et al., 2015a; Menezes et al., 2015b). Molecular pharming by use of plants has recently been discussed elsewhere (Mcnulty et al., 2020).
Any biological system for application in white biotechnology (the implementation of biotechnology in the industrial sphere) must rely on initial source(s) of carbon and nitrogen, as major constituents of biomass (besides oxygen). While critical for the economic viability of a process on Earth (Clomburg et al., 2017; Averesch and Krömer, 2018), this becomes even more imperative in a scenario where resources are strictly limited: organics are present in the Martian regolith (parts-per-billion) (Eigenbrode et al., 2018), most accessible, however, is inorganic carbon in the form of CO2 and molecular nitrogen (N2) in the atmosphere (Nangle et al., 2020a; Berliner et al., 2020). In addition, Space Technology has the requirements of being light, low maintenance/robust, and safe. Especially when implementing biomanufacturing for contingency mitigation, (i.e., on-demand production as means to reduce up-mass rather than merely a backup and subsidiary function), response time becomes critical. Further requirements strongly depend on the target product. For example, in case of pharmaceuticals needed to treat unexpected medical conditions but which cannot be stocked due to low shelf-life, a rapid response (production rate) can be vital. For other products, like materials, efficiency (production yield) may be the most important characteristic in order to deliver the required quantities within the spatial and resource constraints of the outpost. When using microbial fermentation as means to supplement a healthy diet and enhance palatability, quality (production titer/purity) is of foremost importance. This has implications for the process and choice of microbial cell factory, often on a case-by-case basis.
From Autotrophy to Heterotrophy—Impact on Process Parameters and Complexity
While chemical carbon capture and utilization technologies are rapidly advancing (Ho et al., 2019), biology still offers the highest flexibility to fix inorganic carbon in order to make it available as a means of life-support and for further conversion into chemical products (Lee et al., 2019; Chen et al., 2020b; Löwe and Kremling, 2021). For a differentiation on process-level, it is most meaningful to distinguish autotrophic systems into phototrophs and chemotrophs. The former can be microalgae, cyanobacteria, or purple phototrophic bacteria. On Mars, the reduced intensity of natural sunlight (≈60% of Earth’s maximum solar irradiance) has wide ranging implications for application of these organisms to bio-ISRU. To achieve high productivity, it would be imperative to concentrate photons by focusing natural lighting, or to rely on artificial lighting to achieve sufficient photon flux density at the desired wavelength (blue and red range) (Pattison et al., 2018). Both approaches have trade-offs in terms of equivalent system mass (Bugbee et al., 2020), and either further complicates the already complex design of photobioreactors (Johnson et al., 2018). If footprint and productivity constraints are relaxed (considering semi-open cultivation-systems like domed and pressurized ponds instead of photobioreactors), however, it is a straight forward way to generate initial biomass, which can serve as foundation for further biology (Billi et al., 2021; Verseux et al., 2021). Light-independent, lithoautotrophy can fix carbon by relying on chemically provided reducing power. On Mars, this could either directly be electricity through microbial electrosynthesis in bio-electrochemical systems (Moscoviz et al., 2016; Abel et al., 2020; Chen et al., 2020a), or indirectly by means of hydrogen or (organoautotrophically) formate, both of which can also be generated electrochemically (Kracke et al., 2020; Abel and Clark, 2021). Use of other electron donors like, e.g., sulphide, sulphur and iron (II) is theoretically possible, but technically less feasible (crustal materials from Mars are in principle able to support lithotrophic growth (Milojevic et al., 2021), but mining and purifying these in quantities that could support biotechnological processes is likely not viable). A special case is methylotrophy, where the C1-carbon also serves as source of reducing power (chemoorganotrophy). Several ways to obtain hydrogen and methane from CO2 and H2O are being considered for ISRU on Mars (the primary purpose being propellant for the return journey),1 as methane does exist on Mars (Webster et al., 2018) but concentrations are much too low (parts-per-billion) to be readily utilisable (unless minable reservoirs are found). Processes relying on gas or electricity as input generally require more sophisticated process setup. Further, these processes are often limited by mass transfer of the poorly soluble gases (Geinitz et al., 2020). Some also present an explosion hazard. Integrated setups can, however, avoid explosive gas mixtures and achieve significant production rates (Kracke et al., 2020; Sahoo et al., 2021). To completely avoid these problems, methane could be further converted to methanol (Zakaria and Kamarudin, 2016; Latimer et al., 2018)—albeit poor conversion efficiency is a century old unsolved problem. Not as troubled by the fundamental predicament of high activation energy combined with high activity of the CH-bonds in methane, biological methods could remedy this (Averesch and Kracke, 2018; Bjorck et al., 2018). Alternatively, methanol could be directly synthesized catalytically (Basile and Dalena, 2017)2. Another option and a form of chemoorganoautotrophy is utilization of formate not only as electron donor but also carbon-source (Abel and Clark, 2021). All these approaches, however, represents an additional process-step and therefore require auxiliary infrastructure. A biological equivalent to a liquid intermediate-substrate that can serve as unified feedstock for various heterotrophic microbial cell factories is acetate. As alternative to methanol carbonylation3, numerous bioprocesses have emerged that sequestrate CO2 into acetate and it is considered a next-generation biotech-feedstock (Kiefer et al., 2021). Processes with chemoorganoheterotrophic organisms using soluble substrates can achieve high productivities and allow for streamlined process design. The utilization of a defined feedstock also has the advantage of more consistent and predictable process performance, which can be problematic when using crude biomass like e.g., cyanobacterial lysate. A basic overview of the different options for carbon-flow is presented in Figure 1 and Table 1 gives an indication of the advantages and drawbacks on process-level, depending on the type of metabolism of the deployed microbe.
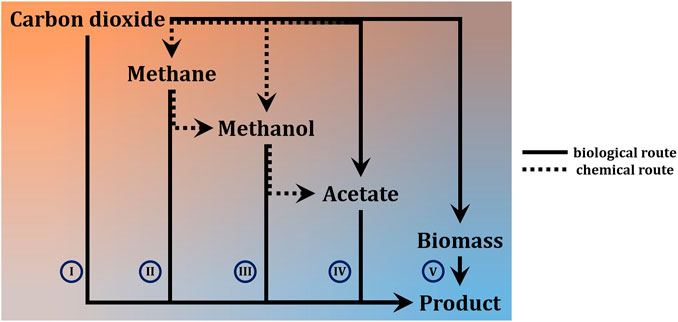
FIGURE 1. High-level differentiation of approaches for conversion of carbon dioxide from Martian atmosphere into carbon-based products via microbial ISRU. Solid lines are bio-based processes, dashed arrows indicate involvement of catalytic (chemical) steps. The fading dashed line indicates a combined chemical-biological approach, rather than alternatives (I) single-step process from inorganic carbon directly to target compound (II)—(IV) processes that rely on significant auxiliary input, (e.g. chemical conversion of CO2 to CH4 or bioconversion of CO2 to CH3COOH) (V) two-step processes via intermediate with broad bio-availability.
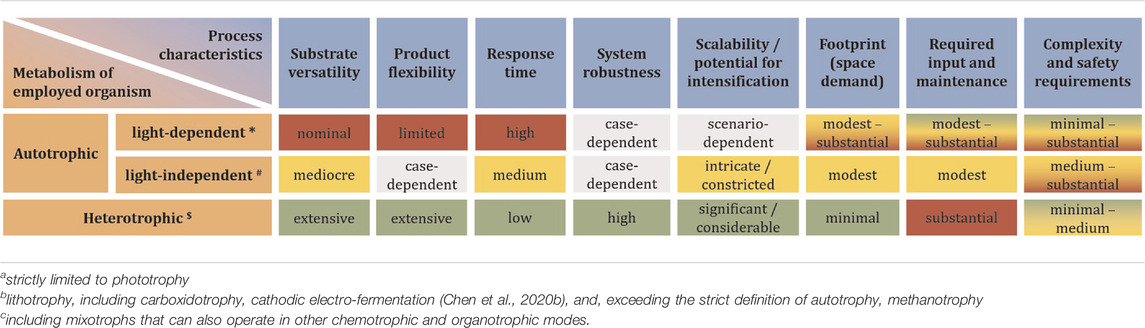
TABLE 1. Qualitative comparison of processes based on the type of metabolism of microbial cell factories to support an initial foothold on Mars in an ISRU scenario. Limited to production of basic compounds (small-, as well as macromolecules but excluding biomanufacturing i.e., physical structures) with pure cultures. Green shading represents an advantage, red shading a disadvantage, yellow a draw. Gray shading indicates non-decision due to great diversity of process factors preventing categorization. It should be noted that the different decision factors do not necessarily have the same weight.
From Substrates to Products—Impact of Synthetic Biology
Genetic tractability is highly desirable for an organism to serve as a versatile chassis for a microbial cell factory that can be tailored toward production of a variety of useful compounds. Hitherto genetic engineering has been adopted more widely with heterotrophic microbes, judging by their dominance in industrial-scale processes and employment as model organisms for genetic studies (Papagianni, 2012). This is likely owed to their more straight forward maintenance, facilitating domestication: their high metabolic rates accelerate cultivation and hence genetic interventions. The requirement for high productivity is likely also an economic “selection-pressure.” In reverse, this may explain the scarcity of genetic tools for autotrophs. Further, especially phototrophs are often more complex organisms with genetic traits like polyploidy and cellular differentiation, (e.g. filamentous, heterocysts, etc.) and/or exotic defense systems (they cannot just “outgrow”), complicating the modification of their genotype (Riley and Guss, 2021). This applies in particular to cyanobacteria and algae (Hitchcock et al., 2020). Nevertheless, recent developments in Synthetic Biology, like CRISPR-technology, have narrowed that gap (Behler et al., 2018). Genetic engineering of chemolithotrophs can be more straightforward as many of them are mixotrophic and can also be cultivated on complex carbon-sources. A wide substrate-range also makes organisms attractive for bioproduction in an ISRU scenario, in particular when a mixed feedstock, derived from waste-biomass or cyanobacterial lysate, is intended to support growth and production (Verseux et al., 2016; Billi et al., 2021). The borders are blurry, as some autotrophs, like, e.g., Cupriavidus necator, can also function heterotrophically or may not even satisfy the strict definition of autotrophy (utilization of inorganic carbon-sources), like methanotrophs. Recently there has also been rapid development in engineering heterotrophic hosts toward artificial autotrophy and methylotrophy, based on natural as well as synthetic pathways for fixation of carbon (Gleizer et al., 2019; Liang et al., 2020; Liu et al., 2020; Satanowski et al., 2020; Wang et al., 2020; Scheffen et al., 2021). This may accelerate the adoption of C1-carbons as feedstocks in white biotechnology, which consequently could also warrant more profound applicability of microbial systems to ISRU and vice versa.
Source of Nitrogen in Light of Carbon-Metabolism
Diazotrophy is ubiquitous among microbes and fixation of nitrogen exists in combination with all modes of carbon and energy metabolism considered in Figure 1. Biological nitrogen-fixation has, however, an excessive energy demand (16 ATP per N2), which translates to low growth-rates and/or high demand for reduced carbon-sources, the latter inevitably accompanied by significant evolution of carbon dioxide. Heterotrophic fixation of nitrogen would thus be at the cost of net carbon-fixation, translating into the demand to significantly increase the dimensions of associated infrastructure and inputs. In addition, it creates a dependency on a supply chain. Therefore, fixation of nitrogen may be best served with a separate, dedicated chemical process, (e.g. Haber-Bosch) or conducted in combination with low-intensity bio-ISRU, like carbon-fixation by photoautotrophs, (e.g., cyanobacteria, Figure 1 route (V)), if availability of power or space permits. The latter would yield a combined (C-N) feedstock, rich in amino sugars like glucosamine, a building block of the peptidoglycan in bacterial biomass (Averesch and Rothschild, 2019).
For production of bulk-compounds that do not contain nitrogen, (e.g. polyesters) N-input may not even be needed, if an efficient circular recycling system is realized. The same is true for food—as long as nitrogen is effectively recovered from the organic waste (Beckinghausen et al., 2020), little supplemental input should be required.
Considerations Beyond Metabolism
In addition to genetic tractability and high metabolic rate, it is desirable that the designated microbial cell factories are robust. Considering the increasing metabolic flexibility on product as well as substrate side, owed to advances in metabolic engineering, the general resilience of the organism may become the more decisive factor for selection of a microbial cell factory for application in Space Technology. Particularly relevant in order to compromise intermittency and unexpected down-time is for example the resistance of spore-forming species to vacuum and desiccation (Horneck, 1993), as well as tolerance of certain cyanobacteria toward high perchlorate concentrations (Billi et al., 2021), or the radiation resistance of certain bacteria (Krisko and Radman, 2013) and fungi (Shunk et al., 2020).
Nevertheless, despite all advances in Synthetic Biology, there may in some cases not be a choice: for complex products, like therapeutic proteins or products derived from plant-pathways, (e.g. natural products, where enzymes require post-translational modifications for activity) (Kayser and Averesch, 2015), almost exclusively only heterotrophs can be used, as these capabilities are limited to eukaryotic organisms, like yeasts (Nielsen, 2013).
Lastly, process intensification is critical in order to conserve precious resources, like water, shifting importance of substrate availability to auxiliary resources and sizing of infrastructure and secondary support processes. Because the same principles and constraints apply on Mars as on Earth, the synergistic integration of metabolic and process engineering can advance this (Woodley, 2017) to successfully integrate biomanufacturing into mission design. The extent to which this is possible of course strongly depends on the microbes and their unique metabolism, but also other factors, like preference to grow in biofilms, to produce by-products that reduce surface tension thus resulting in foaming, or resilience in the face of stressors like shear-force and sudden changes of environmental parameters.
Organisms With High Potential for Bio-ISRU on Mars
For production of biomass, the diazotrophic and spore-forming cyanobacterium species Anabaena, which is tolerant to Mars-like growth-conditions (Verseux et al., 2021), holds great promise. Synechocystis sp. PCC 6803, is also able to grow in high-CO2 atmospheres (Murukesan et al., 2016)—albeit not nitrogen-fixing, it is much more amenable to genetic engineering (Sebesta et al., 2019), can switch between auto- and heterotrophy and naturally produces bio-polyesters. It may therefore be attractive as a system for production of basic resources and/or food supplements.
Hydrogen oxidizing bacteria like Cupriavidus necator and/or acetogens can directly convert CO2 to products. The mixotroph C. necator also utilizes substrates like sugars and organic acids (formate, acetate), and is an excellent producer of bio-polyesters (Raberg et al., 2018). An extensive library of proven genetic tools allows its engineering into microbial cell factories for a wide range of products, including high-performance polymers, as well as expansion of its substrate range (Heinrich et al., 2018; Nangle et al., 2020b). Anaerobic (homo)acetogens (like, e.g. Sporomusa ovata) have a lower metabolic rate and are less genetically tractable, but also have a remarkable substrate range in addition to their unique reductive acetyl-CoA pathway, the latter making them attractive to directly convert CO and/or CO2 to organic acids and alcohols (Schuchmann and Müller, 2016; Su et al., 2020). Certain PPBs are even more flexible in terms of carbon- and energy-source (chemo-, auto-/heterotrophic), capable of anoxygenic photosynthesis, oxidize carbon monoxide, and are in addition also diazotrophic. Some species can be genetically engineered, (e.g. Rhodospirillum rubrum or Rhodopseudomonas palustris), and have previously been used for production of fuels and advanced bio-polyesters (Heinrich et al., 2016; Doud et al., 2017). Many of these species have also been studied in BES for potential to drive metabolism bio-electrochemically and perform MES (Nevin et al., 2011; Bose et al., 2014; Liu et al., 2016), to intensify process operations and increase efficacy.
Methanotrophs stand apart from most other C1-utilizing microbes and rarely accept other substrates (except for methanol). Of interest for application in bio-ISRU are mostly aerobic species, in particular of type I and II as they exceed rates of anaerobic methanotrophs by orders of magnitude, albeit at reduced efficiency, (i.e. carbon-yield) (Averesch and Kracke, 2018). In particular alkalophilic and halotolerant Methylomicrobium species could simplify axenic cultivation by abolishing the need for strictly sterile conditions, as contamination is unlikely. These methanotrophs and also certain methylotrophs, like Methylobacterium extorquens and Bacillus species can to some extent also be genetically engineered (Kalyuzhnaya et al., 2015).
Many Bacillus species also grow on various sugars and organic acids. In particular B. subtilis, a very versatile model-organism, industrially employed for protein-production, as well as in metabolic engineering (Averesch and Rothschild, 2019), is strongly being considered and studied for application in Space Synthetic Biology, due to the resistance of its spores (Horneck, 1993). It also has a rapid metabolism and growth rate, exceeded by few other organisms. Vibrio natrigens is an emerging workhorse of white biotechnology, able to use a wide range of common organic molecules as its sole source of carbon and energy (Austin et al., 1978); its biggest advantage, however, being a doubling-time just under 10 min (Thoma and Blombach, 2021). It’s exceptionally fast metabolism alone may warrant employment for specialty applications in Space Synthetic Biology, potentially in combination with adaptive evolution, which strongly depends on rate of replication (Dragosits and Mattanovich, 2013). Another organism highly attractive for vital special applications is the yeast Pichia pastoris, a methylotrophic eukaryote most valued for production of recombinant proteins (Schwarzhans et al., 2017).
Topics not closely discussed here are biohydrometallurgy and bioremediation for extraction and recovery of rare Earth elements, precious metals, as well as perchlorate removal, since their primary purpose is not directly related to the conversion of carbon. Nevertheless, they should at least be briefly mentioned as they are important strategies for bio-ISRU. Several microorganisms have been utilized in proof-of-concept experiments for biomining on Earth, as well as the proving-ground of the International Space Station (Johnson, 2014; Schippers et al., 2014; Jerez, 2017; Loudon et al., 2018; Cockell et al., 2020; Volger et al., 2020; Cockell et al., 2021). In particular, Acidithiobacillus ferroxidans, Cupriavidus metallidurans, Shewanella oneidensis and Sphingomonas desiccabilis are promising, most of these species performing chemolithotrophic leaching.
Conclusion
There is likely no single “golden” solution that serves all purposes and covers all needs in any situation. Most likely several complementary systems will have to be advanced toward technological readiness, to cover all applications and for reasons of redundancy. A three-fold approach could make most sense: Diazotrophic cyanobacteria could be utilized to continuously produce biomass as a form of unspecific/universal feedstock from crude Martian resources. Versatile (mixotrophic) natively or artificially chemoautotropic strains could be deployed in parallel to rapidly produce a variety of bulk compounds from flexible and readily available C1-feedstocks. In order to provide for unusual and speciality products needed on short notice, a few highly specialized heterotrophs could be employed. A biofoundry, (i.e. automated infrastructure for engineering and analytics of biological systems) (Holowko et al., 2020), if such can be accommodated in the mission design, may even allow these be constructed at destination as the need arises, based on design-plans transmitted from Earth.
Data Availability Statement
The original contributions presented in the study are included in the article/supplementary material, further inquiries can be directed to the corresponding author.
Author Contributions
NA conceived and composed the manuscript.
Funding
NA was supported by a 2021 Stanford Natural Gas Initiative (NGI) program grant (SPO #139138), as well as NASA grant or cooperative agreement award number NNX17AJ31G. Any opinions, findings, and conclusion or recommendations expressed in this material are those of the author and do not necessarily reflect the views of the National Aeronautics and Space Administration (NASA).
Conflict of Interest
The author declares that the research was conducted in the absence of any commercial or financial relationships that could be construed as a potential conflict of interest.
Abbreviations
BES, bio-electrochemical system; BLSS, bio-regenerative life-support system; ISRU, in-situ resource utilization; ISS, International Space Station; MES, microbial electrosynthesis; PPB, purple phototrophic bacteria.
Footnotes
1Chemical synthesis of methane (I) via coupling of water-electrolysis or sulphur–iodine cycle and Sabatier reaction: “2 H2O+ CO2 → 2 O2 + CH4” (Clark, 1997; Ying et al., 2017) (II) as a by-product of solid oxide electrolysis followed by methanation: “CO2 + H2O → CO + H2 + O2,” “CO + 3 H2 → CH4 + H2O” (Biswas et al., 2020; Hecht et al., 2021) or (III) via methanogenesis in an integrated BES: “2 H2O+ CO2 → 2 O2 + CH4” (Kracke et al., 2020).
2Chemical synthesis of methanol from synthesis gas or carbon dioxide and hydrogen, overall reaction: “CO2 + 3 H2 → CH3OH + H2O.”
3Chemical synthesis of acetate from methanol and carbon monoxide: “CH3OH + CO → CH3COOH.”
References
Abel, A. J., and Clark, D. S. (2021). A Comprehensive Modeling Analysis of Formate‐Mediated Microbial Electrosynthesis**. ChemSusChem 14, 344–355. doi:10.1002/cssc.202002079
Abel, A. J., Hilzinger, J. M., Arkin, A. P., and Clark, D. S. (2020). Systems-informed Genome Mining for Electroautotrophic Microbial Production. bioRxiv 2012, 414987. doi:10.1101/2020.12.07.414987
Austin, B., Zachary, A., and Colwell, R. R. (1978). Recognition of Beneckea natriegens (Payne et al.) Baumann et al. as a Member of the Genus Vibrio, as Previously Proposed by Webb and Payne. Int. J. Syst. Bacteriol. 28, 315–317. doi:10.1099/00207713-28-2-315
Averesch, N. J. H., and Kracke, F. (2018). Metabolic Network Analysis of Microbial Methane Utilization for Biomass Formation and Upgrading to Bio-Fuels. Front. Energ. Res. 6. doi:10.3389/fenrg.2018.00106
Averesch, N. J. H., and Krömer, J. O. (2018). Metabolic Engineering of the Shikimate Pathway for Production of Aromatics and Derived Compounds—Present and Future Strain Construction Strategies. Front. Bioeng. Biotechnol. 6. doi:10.3389/fbioe.2018.00032
Averesch, N. J. H., and Rothschild, L. J. (2019). Metabolic Engineering ofBacillus Subtilisfor Production Ofpara‐aminobenzoic Acid - Unexpected Importance of Carbon Source Is an Advantage for Space Application. Microb. Biotechnol. 12, 703–714. doi:10.1111/1751-7915.13403
Basile, A., and Dalena, F. (2017). Methanol: Science and Engineering. Amsterdam, Netherlands; Oxford, United Kingdom; Cambridge, United States: Elsevier B.V.
Beckinghausen, A., Odlare, M., Thorin, E., and Schwede, S. (2020). From Removal to Recovery: An Evaluation of Nitrogen Recovery Techniques from Wastewater. Appl. Energ. 263, 114616. doi:10.1016/j.apenergy.2020.114616
Behler, J., Vijay, D., Hess, W. R., and Akhtar, M. K. (2018). CRISPR-based Technologies for Metabolic Engineering in Cyanobacteria. Trends Biotechnol. 36, 996–1010. doi:10.1016/j.tibtech.2018.05.011
Berliner, A., Hilzinger, J. M., Abel, A. J., Mcnulty, M., Makrygiorgos, G., Averesch, N. J., et al. (2020). Towards a Biomanufactory on Mars. 2020120714. doi:10.20944/preprints202012.0714
Billi, D., Gallego Fernandez, B., Fagliarone, C., Chiavarini, S., and Rothschild, L. J. (2021). Exploiting a Perchlorate-Tolerant Desert Cyanobacterium to Support Bacterial Growth for In Situ Resource Utilization on Mars. Int. J. Astrobiology 20, 29–35. doi:10.1017/s1473550420000300
Biswas, S., Kulkarni, A. P., Giddey, S., and Bhattacharya, S. (2020). A Review on Synthesis of Methane as a Pathway for Renewable Energy Storage with a Focus on Solid Oxide Electrolytic Cell-Based Processes. Front. Energ. Res. 8, 229. doi:10.3389/fenrg.2020.570112
Bjorck, C. E., Dobson, P. D., and Pandhal, J. (2018). Biotechnological Conversion of Methane to Methanol: Evaluation of Progress and Potential. AIMS Bioeng. 5 (1), 1–38. doi:10.3934/bioeng.2018.1.1
Bose, A., Gardel, E. J., Vidoudez, C., Parra, E. A., and Girguis, P. R. (2014). Electron Uptake by Iron-Oxidizing Phototrophic Bacteria. Nat. Commun. 5, 3391. doi:10.1038/ncomms4391
Bugbee, B., Hardy, M., Wheeler, R., Ewert, M., and Kusuma, P. (2020). “Providing Photons for Food in Regenerative Life Support: A Comparative Analysis of Solar Fiber Optic and Electric Light Systems,” in 50th International Conference on Environmental Systems.
Chen, H., Dong, F., and Minteer, S. D. (2020a). The Progress and Outlook of Bioelectrocatalysis for the Production of Chemicals, Fuels and Materials. Nat. Catal. 3, 225–244. doi:10.1038/s41929-019-0408-2
Chen, Y., Banerjee, D., Mukhopadhyay, A., and Petzold, C. J. (2020b). Systems and Synthetic Biology Tools for Advanced Bioproduction Hosts. Curr. Opin. Biotechnol. 64, 101–109. doi:10.1016/j.copbio.2019.12.007
Clark, D. L. (1997). “In-situ Propellant Production on Mars - A Sabatier/electrolysis Demonstration Plant,” in 33rd Joint Propulsion Conference and Exhibit, July 6–9, 1997. Seattle, WA: American Institute of Aeronautics and Astronautics. doi:10.2514/6.1997-2764
Clomburg, J. M., Crumbley, A. M., and Gonzalez, R. (2017). Industrial Biomanufacturing: The Future of Chemical Production. Science 355, aag0804. doi:10.1126/science.aag0804
Cockell, C. S., Santomartino, R., Finster, K., Waajen, A. C., Eades, L. J., Moeller, R., et al. (2020). Space Station Biomining experiment Demonstrates Rare Earth Element Extraction in Microgravity and Mars Gravity. Nat. Commun. 11, 5523. doi:10.1038/s41467-020-19276-w
Cockell, C. S., Santomartino, R., Finster, K., Waajen, A. C., Nicholson, N., Loudon, C.-M., et al. (2021). Microbially-Enhanced Vanadium Mining and Bioremediation under Micro- and Mars Gravity on the International Space Station. Front. Microbiol. 12, 641387. doi:10.3389/fmicb.2021.641387
Doud, D. F. R., Holmes, E. C., Richter, H., Molitor, B., Jander, G., and Angenent, L. T. (2017). Metabolic Engineering of Rhodopseudomonas Palustris for the Obligate Reduction of N-Butyrate to N-Butanol. Biotechnol. Biofuels 10, 178. doi:10.1186/s13068-017-0864-3
Dragosits, M., and Mattanovich, D. (2013). Adaptive Laboratory Evolution - Principles and Applications for Biotechnology. Microb. Cel Fact 12, 64. doi:10.1186/1475-2859-12-64
Eigenbrode, J. L., Summons, R. E., Steele, A., Freissinet, C., Millan, M., Navarro-González, R., et al. (2018). Organic Matter Preserved in 3-Billion-Year-Old Mudstones at Gale Crater, Mars. Science 360, 1096–1101. doi:10.1126/science.aas9185
Geinitz, B., Hüser, A., Mann, M., and Büchs, J. (2020). Gas Fermentation Expands the Scope of a Process Network for Material Conversion. Chem. Ingenieur Technik 92, 1665–1679. doi:10.1002/cite.202000086
Gleizer, S., Ben-Nissan, R., Bar-On, Y. M., Antonovsky, N., Noor, E., Zohar, Y., et al. (2019). Conversion of Escherichia coli to Generate All Biomass Carbon from CO2. Cell 179, 1255–1263. doi:10.1016/j.cell.2019.11.009
Hastings, J. J. A., and Nangle, S. N. (2019). “Biotechnological Strategies for Sustained Human Presence on Mars,” in 70th International Astronautical Congress, October 21–25, 2019. Washington, DC: International Astronautical Federation.
Hecht, M., Hoffman, J., Rapp, D., Mcclean, J., Soohoo, J., Schaefer, R., et al. (2021). Mars Oxygen ISRU Experiment (MOXIE). Space Sci. Rev. 217, 9. doi:10.1007/s11214-020-00782-8
Heinrich, D., Raberg, M., Fricke, P., Kenny, S. T., Morales-Gamez, L., Babu, R. P., et al. (2016). Synthesis Gas (Syngas)-Derived Medium-Chain-Length Polyhydroxyalkanoate Synthesis in Engineered Rhodospirillum Rubrum. Appl. Environ. Microbiol. 82, 6132–6140. doi:10.1128/aem.01744-16
Heinrich, D., Raberg, M., and Steinbüchel, A. (2018). Studies on the Aerobic Utilization of Synthesis Gas (Syngas) by Wild Type and Recombinant Strains ofRalstonia eutrophaH16. Microb. Biotechnol. 11, 647–656. doi:10.1111/1751-7915.12873
Hitchcock, A., Hunter, C. N., and Canniffe, D. P. (2020). Progress and Challenges in Engineering Cyanobacteria as Chassis for Light‐driven Biotechnology. Microb. Biotechnol. 13, 363–367. doi:10.1111/1751-7915.13526
Ho, H.-J., Iizuka, A., and Shibata, E. (2019). Carbon Capture and Utilization Technology without Carbon Dioxide Purification and Pressurization: A Review on its Necessity and Available Technologies. Ind. Eng. Chem. Res. 58, 8941–8954. doi:10.1021/acs.iecr.9b01213
Holowko, M. B., Frow, E. K., Reid, J. C., Rourke, M., and Vickers, C. E. (2020). Building a Biofoundry. Synth. Biol. 6, ysaa026. doi:10.1093/synbio/ysaa026
Horneck, G. (1993). Responses ofBacillus Subtilis Spores to Space Environment: Results from Experiments in Space. Origins Life Evol. Biosph. 23, 37–52. doi:10.1007/bf01581989
Jerez, C. A. (2017). Biomining of Metals: How to Access and Exploit Natural Resource Sustainably. Microb. Biotechnol. 10, 1191–1193. doi:10.1111/1751-7915.12792
Johnson, D. B. (2014). Biomining-biotechnologies for Extracting and Recovering Metals from Ores and Waste Materials. Curr. Opin. Biotechnol. 30, 24–31. doi:10.1016/j.copbio.2014.04.008
Johnson, T. J., Katuwal, S., Anderson, G. A., Gu, L., Zhou, R., and Gibbons, W. R. (2018). Photobioreactor Cultivation Strategies for Microalgae and Cyanobacteria. Biotechnol. Prog. 34, 811–827. doi:10.1002/btpr.2628
Kalkus, T. J., Averesch, N. J., and Lehner, B. A. (2018). 69th International Astronautical Congress. Bremen, Germany: International Astronautical Federation.The Power of Life: How Biology Can Help Address the Long-Term Energy Demands of Space Colonization
Kalyuzhnaya, M. G., Puri, A. W., and Lidstrom, M. E. (2015). Metabolic Engineering in Methanotrophic Bacteria. Metab. Eng. 29, 142–152. doi:10.1016/j.ymben.2015.03.010
Kayser, O., and Averesch, N. (2015). Technische Biochemie. Wiesbaden: Springer Spektrum. doi:10.1007/978-3-658-05548-6
Kiefer, D., Merkel, M., Lilge, L., Henkel, M., and Hausmann, R. (2021). From Acetate to Bio-Based Products: Underexploited Potential for Industrial Biotechnology. Trends Biotechnol. 39, 397–411. doi:10.1016/j.tibtech.2020.09.004
Kracke, F., Deutzmann, J. S., Gu, W., and Spormann, A. M. (2020). In Situ electrochemical H2 Production for Efficient and Stable Power-To-Gas Electromethanogenesis. Green. Chem. 22, 6194–6203. doi:10.1039/d0gc01894e
Krisko, A., and Radman, M. (2013). Biology of Extreme Radiation Resistance: The Way of Deinococcus Radiodurans. Cold Spring Harbor Perspect. Biol. 5. doi:10.1101/cshperspect.a012765
Latimer, A. A., Kakekhani, A., Kulkarni, A. R., and Nørskov, J. K. (2018). Direct Methane to Methanol: The Selectivity-Conversion Limit and Design Strategies. ACS Catal. 8, 6894–6907. doi:10.1021/acscatal.8b00220
Lee, S. Y., Kim, H. U., Chae, T. U., Cho, J. S., Kim, J. W., Shin, J. H., et al. (2019). A Comprehensive Metabolic Map for Production of Bio-Based Chemicals. Nat. Catal. 2, 18–33. doi:10.1038/s41929-018-0212-4
Liang, B., Zhao, Y., and Yang, J. (2020). Recent Advances in Developing Artificial Autotrophic Microorganism for Reinforcing CO2 Fixation. Front. Microbiol. 11. doi:10.3389/fmicb.2020.632455
Liu, C., Colón, B. C., Ziesack, M., Silver, P. A., and Nocera, D. G. (2016). Water Splitting-Biosynthetic System with CO2 reduction Efficiencies Exceeding Photosynthesis. Science 352, 1210–1213. doi:10.1126/science.aaf5039
Liu, Z., Wang, K., Chen, Y., Tan, T., and Nielsen, J. (2020). Third-generation Biorefineries as the Means to Produce Fuels and Chemicals from CO2. Nat. Catal. 3, 274–288. doi:10.1038/s41929-019-0421-5
Llorente, B., Williams, T., and Goold, H. (2018). The Multiplanetary Future of Plant Synthetic Biology. Genes 9, 348. doi:10.3390/genes9070348
Lopez, J. V., Peixoto, R. S., and Rosado, A. S. (2019). Inevitable Future: Space Colonization beyond Earth with Microbes First. FEMS Microbiol. Ecol. 95, fiz127. doi:10.1093/femsec/fiz127
Loudon, C.-M., Nicholson, N., Finster, K., Leys, N., Byloos, B., Van Houdt, R., et al. (2018). BioRock: New Experiments and Hardware to Investigate Microbe-mineral Interactions in Space. Int. J. Astrobiology 17, 303–313. doi:10.1017/s1473550417000234
Löwe, H., and Kremling, A. (2021). In-depth Computational Analysis of Natural and Artificial Carbon Fixation Pathways. bioRxiv 2001, 425423. doi:10.1101/2021.01.05.425423
Mcnulty, M. J., Xiong, Y., Yates, K., Karuppanan, K., Hilzinger, J. M., Berliner, A. J., et al. (2020). Molecular Pharming to Support Human Life on the Moon, Mars, and beyond. [Epub ahead of print]. doi:10.1080/07388551.2021.1888070
Menezes, A. A., Cumbers, J., Hogan, J. A., and Arkin, A. P. (2015a). Towards Synthetic Biological Approaches to Resource Utilization on Space Missions. J. R. Soc. Interf. 12, 20140715. doi:10.1098/rsif.2014.0715
Menezes, A. A., Montague, M. G., Cumbers, J., Hogan, J. A., and Arkin, A. P. (2015b). Grand Challenges in Space Synthetic Biology. J. R. Soc. Interf. 12, 20150803. doi:10.1098/rsif.2015.0803
Milojevic, T., Albu, M., Kölbl, D., Kothleitner, G., Bruner, R., and Morgan, M. L. (2021). Chemolithotrophy on the Noachian Martian Breccia NWA 7034 via Experimental Microbial Biotransformation. Commun. Earth Environ. 2, 39. doi:10.1038/s43247-021-00105-x
Moscoviz, R., Toledo-Alarcón, J., Trably, E., and Bernet, N. (2016). Electro-Fermentation: How to Drive Fermentation Using Electrochemical Systems. Trends Biotechnol. 34, 856–865. doi:10.1016/j.tibtech.2016.04.009
Murukesan, G., Leino, H., Mäenpää, P., Ståhle, K., Raksajit, W., Lehto, H. J., et al. (2016). Pressurized Martian-like Pure CO2 Atmosphere Supports Strong Growth of Cyanobacteria, and Causes Significant Changes in Their Metabolism. Orig Life Evol. Biosph. 46, 119–131. doi:10.1007/s11084-015-9458-x
Nangle, S. N., Wolfson, M. Y., Hartsough, L., Ma, N. J., Mason, C. E., Merighi, M., et al. (2020a). The Case for Biotech on Mars. Nat. Biotechnol. 38, 401–407. doi:10.1038/s41587-020-0485-4
Nangle, S. N., Ziesack, M., Buckley, S., Trivedi, D., Loh, D. M., Nocera, D. G., et al. (2020b). Valorization of CO2 through Lithoautotrophic Production of Sustainable Chemicals in Cupriavidus Necator. Metab. Eng. 62, 207–220. doi:10.1016/j.ymben.2020.09.002
Nevin, K. P., Hensley, S. A., Franks, A. E., Summers, Z. M., Ou, J., Woodard, T. L., et al. (2011). Electrosynthesis of Organic Compounds from Carbon Dioxide Is Catalyzed by a Diversity of Acetogenic Microorganisms. Appl. Environ. Microbiol. 77, 2882–2886. doi:10.1128/aem.02642-10
Nielsen, J. (2013). Production of Biopharmaceutical Proteins by Yeast. Bioengineered 4, 207–211. doi:10.4161/bioe.22856
Papagianni, M. (2012). Recent Advances in Engineering the central Carbon Metabolism of Industrially Important Bacteria. Microb. Cel Fact 11, 50. doi:10.1186/1475-2859-11-50
Pattison, P. M., Tsao, J. Y., Brainard, G. C., and Bugbee, B. (2018). LEDs for Photons, Physiology and Food. Nature 563, 493–500. doi:10.1038/s41586-018-0706-x
Raberg, M., Volodina, E., Lin, K., and Steinbüchel, A. (2018). Ralstonia eutrophaH16 in Progress: Applications beside PHAs and Establishment as Production Platform by Advanced Genetic Tools. Crit. Rev. Biotechnol. 38, 494–510. doi:10.1080/07388551.2017.1369933
Riley, L. A., and Guss, A. M. (2021). Approaches to Genetic Tool Development for Rapid Domestication of Non-model Microorganisms. Biotechnol. Biofuels 14, 30. doi:10.1186/s13068-020-01872-z
Sahoo, K. K., Goswami, G., and Das, D. (2021). Biotransformation of Methane and Carbon Dioxide into High-Value Products by Methanotrophs: Current State of Art and Future Prospects. Front. Microbiol. 12. doi:10.3389/fmicb.2021.636486
Satanowski, A., Dronsella, B., Noor, E., Vögeli, B., He, H., Wichmann, P., et al. (2020). Awakening a Latent Carbon Fixation Cycle in Escherichia coli. Nat. Commun. 11, 5812. doi:10.1038/s41467-020-19564-5
Scheffen, M., Marchal, D. G., Beneyton, T., Schuller, S. K., Klose, M., Diehl, C., et al. (2021). A New-To-Nature Carboxylation Module to Improve Natural and Synthetic CO2 Fixation. Nat. Catal. 4, 105–115. doi:10.1038/s41929-020-00557-y
Schippers, A., Hedrich, S., Vasters, J., Drobe, M., Sand, W., and Willscher, S. (2014). “Biomining: Metal Recovery from Ores with Microorganisms,” in Geobiotechnology I: Metal-Related Issues. Editors A. Schippers, F. Glombitza, and W. Sand (Berlin, Heidelberg: Springer Berlin Heidelberg), 1–47.
Schuchmann, K., and Müller, V. (2016). Energetics and Application of Heterotrophy in Acetogenic Bacteria. Appl. Environ. Microbiol. 82, 4056–4069. doi:10.1128/aem.00882-16
Schwarzhans, J.-P., Luttermann, T., Geier, M., Kalinowski, J., and Friehs, K. (2017). Towards Systems Metabolic Engineering in Pichia pastoris. Biotechnol. Adv. 35, 681–710. doi:10.1016/j.biotechadv.2017.07.009
Sebesta, J., Werner, A., and Peebles, C. A. M. (2019). Genetic Engineering of Cyanobacteria: Design, Implementation, and Characterization of Recombinant Synechocystis Sp. PCC 6803. Methods Mol. Biol., 139–154. doi:10.1007/978-1-4939-9142-6_101927
Shunk, G. K., Gomez, X. R., and Averesch, N. J. H. (2020). A Self-Replicating Radiation-Shield for Human Deep-Space Exploration: Radiotrophic Fungi Can Attenuate Ionizing Radiation Aboard the International Space Station. bioRxiv 2007, 205534. doi:10.1101/2020.07.16.205534
Su, Y., Cestellos-Blanco, S., Kim, J. M., Shen, Y.-X., Kong, Q., Lu, D., et al. (2020). Close-Packed Nanowire-Bacteria Hybrids for Efficient Solar-Driven CO2 Fixation. Joule 4, 800–811. doi:10.1016/j.joule.2020.03.001
Thoma, F., and Blombach, B. (2021). Metabolic Engineering of Vibrio Natriegens. Essays Biochem. EBC20200135. doi:10.1042/EBC20200135
Verseux, C., Baqué, M., Lehto, K., De Vera, J.-P. P., Rothschild, L. J., and Billi, D. (2016). Sustainable Life Support on Mars - the Potential Roles of Cyanobacteria. Int. J. Astrobiology 15, 65–92. doi:10.1017/s147355041500021x
Verseux, C., Heinicke, C., Ramalho, T. P., Determann, J., Duckhorn, M., Smagin, M., et al. (2021). A Low-Pressure, N2/CO2 Atmosphere Is Suitable for Cyanobacterium-Based Life-Support Systems on Mars. Front. Microbiol. 12, 611798. doi:10.3389/fmicb.2021.611798
Volger, R., Pettersson, G. M., Brouns, S. J. J., Rothschild, L. J., Cowley, A., and Lehner, B. A. E. (2020). Mining Moon & mars with Microbes: Biological Approaches to Extract Iron from Lunar and Martian Regolith. Planet. Space Sci. 184, 104850. doi:10.1016/j.pss.2020.104850
Wang, Y., Fan, L., Tuyishime, P., Zheng, P., and Sun, J. (2020). Synthetic Methylotrophy: A Practical Solution for Methanol-Based Biomanufacturing. Trends Biotechnol. 38, 650–666. doi:10.1016/j.tibtech.2019.12.013
Webster, C. R., Mahaffy, P. R., Atreya, S. K., Moores, J. E., Flesch, G. J., Malespin, C., et al. (2018). Background Levels of Methane in Mars' Atmosphere Show strong Seasonal Variations. Science 360, 1093–1096. doi:10.1126/science.aaq0131
Woodley, J. M. (2017). Bioprocess Intensification for the Effective Production of Chemical Products. Comput. Chem. Eng. 105, 297–307. doi:10.1016/j.compchemeng.2017.01.015
Ying, Z., Zheng, X., Zhang, Y., and Cui, G. (2017). Development of a Novel Flowsheet for Sulfur-Iodine Cycle Based on the Electrochemical Bunsen Reaction for Hydrogen Production. Int. J. Hydrogen Energ. 42, 26586–26596. doi:10.1016/j.ijhydene.2017.09.035
Keywords: Mars, carbon-fixation, bioproduction, synthetic biology, microbial bioprocess, bio-regenerate life-support system, in-situ resource utilization
Citation: Averesch NJH (2021) Choice of Microbial System for In-Situ Resource Utilization on Mars. Front. Astron. Space Sci. 8:700370. doi: 10.3389/fspas.2021.700370
Received: 26 April 2021; Accepted: 17 June 2021;
Published: 30 June 2021.
Edited by:
Cyprien Verseux, University of Bremen, GermanyReviewed by:
Alex Price, The Open University, United KingdomBaptiste Leroy, University of Mons, Belgium
Copyright © 2021 Averesch. This is an open-access article distributed under the terms of the Creative Commons Attribution License (CC BY). The use, distribution or reproduction in other forums is permitted, provided the original author(s) and the copyright owner(s) are credited and that the original publication in this journal is cited, in accordance with accepted academic practice. No use, distribution or reproduction is permitted which does not comply with these terms.
*Correspondence: Nils Jonathan Helmuth Averesch, bmlscy5hdmVyZXNjaEB1cS5uZXQuYXU=