- Department of Molecular Evolution, Centro de Astrobiología (Consejo Superior de Investigaciones Científicas-Instituto Nacional de Técnica Aeroespacial Esteban Terradas), Madrid, Spain
Icy moons in our solar system are targets of interest to search for signs of life and habitability beyond Earth. They are the astrobiological aim for several developing space missions (JUICE, Europa Clipper, and Europa Lander). A further understanding of the processes that force a planetary object to develop a biological system and to develop habitability conditions could be obtained by adopting a prebiotic chemistry point of view. In this regard, it is highly interesting to search for organic compounds synthesized from cyanide since it has been proposed that polymerization of NH4CN would be applicable to icy planetary environments. Thus, the effect of water freeze-thaw cycles, UV-radiation and salts on the polymerization of NH4CN was explored as an approach to understand the possible organic chemistry of icy worlds. As a result, insoluble and soluble NH4CN polymers, synthesized under multiple conditions, were analyzed by GC-MS. A diverse set of amino acids, carboxylic acids, and several N-heterocycles were identified. Glyoxylic acid was detected under particular reaction conditions, extending the plausible favorable conditions for the emergence of this molecule, referred to as the “glyoxylate scenario.” In addition, the GC-MS results were studied by multivariate analysis, showing that the ice-water interfaces may be ideal places to develop complex organic chemistry from a carbon source as simple as cyanide.
Introduction
Icy moons in our solar system are some of the places that astrobiologists are studying to search for signs of life beyond Earth. Several of these moons have subsurface oceans that, combined, contain many times the volume of liquid water on Earth. The icy moons of the Solar System and their potential habitability conditions are a target for the scientific community (e.g., Sephton et al., 2018; Sherwood et al., 2018). Furthermore, they are the astrobiological aim for several space missions currently being developed: JUICE (Grasset et al., 2013), Europa Clipper (Campagnola et al., 2019), and Europa Lander (Pappalardo et al., 2013). The processes that cause a planet or a satellite to develop a biological system and habitability conditions could be further understood by adopting a prebiotic chemistry point of view. In this regard, it is highly interesting to search for organic compounds synthesized from HCN because this molecule is considered a key to understanding the origin of a potential protometabolic system (e.g., Eschenmoser, 2007; Marín-Yaseli et al., 2016; Sutherland, 2016), and it is ubiquitous in the universe (e.g., Bacchus-Montabonel, 2017; Rannou and West, 2018), including in terrestrial hydrothermal environments (e.g., Holm and Neubeck, 2009; Huber et al., 2012). The presence of hydrothermal systems on the icy moons of the Solar System has been demonstrated (e.g., Sparks et al., 2016, 2017; Waite et al., 2017), and due to the mechanism of HCN production (e.g., the review Ruiz-Bermejo et al., 2013b), the presence of HCN in the subsurface oceans of these icy moons can be assumed. Additionally, exogenous sources of HCN on these moons may be considered (Levy et al., 2000). The water HCN polymerization reactions are efficient means to obtain purine, pyrimidine, pteridine, amino acid, and carboxylic acid precursors (e.g., Ruiz-Bermejo et al., 2013b; Marín-Yaseli et al., 2015). However, such reactions require high concentrations (>0.01 M) of HCN to overcome the competing hydrolysis processes that lead to the formation of formate and ammonia. One means to obtain high concentrations of HCN is eutectic freezing of dilute solutions of HCN (Sánchez et al., 1966). Thus, since high concentrations of NH4CN can be obtain by freezing, polymerization of NH4CN would be applicable to icy planetary environments (Levy et al., 1999). Additionally, polymerization of HCN (possible at pH 8–10) may be supported by plausible alkaline conditions in these kinds of environments (Russell et al., 2017). In addition, the existence of liquid water plumes with eruptions of CH4, CO, NH3, and H2; simple and complex organic matter on Enceladus (Waite et al., 2017; Postberg et al., 2018), in addition to the observed plumes (Brainard, 2018; Jia et al., 2018); and plausible plate tectonics (Kattenhorn, 2018) on Europa suggest melting and freezing processes and, subsequently, plausible water freeze-melt cycles on these icy moons. During the freeze-thaw cycles, the temperature gradients and changes in the water density induce pressure and potential gradients along the ice canals and changes in the water dielectric constant (e.g., West et al., 2007), and the icy surface presents catalytic properties (Graham and Roberts, 2000). Taking into account all these characteristics of the ices, the ice-water matrix can be considered a plausible prebiotic reactor implicated in the production of building blocks for life. In this sense, it has been shown that the ice-water matrix may have acted as an efficient environment for the production of organic prebiotic molecules (Menor-Salvan and Marín-Yaseli, 2012), monomer condensation (Lui and Orgel, 1998), promotion of the RNA biogenesis (Monnard et al., 2003), and RNA replication (Attwater et al., 2010) due to the concentrator effect of the ice and stabilization of thermolabile compounds.
On the other hand, UV radiation, like HCN, is ubiquitous in the universe, and thus, it can play an important role in chemical evolution. Most works focus on prebiotic chemistry using vacuum UV radiation (10–200 nm) to simulate the conditions of the ISM. UV radiation using Hg lamps has been considered in only a few studies that considered the production of organics from HCN/cyanide as main reactant (Abelson, 1966; Mizutani et al., 1975; Hartig and Getoff, 1980; Ritson and Sutherland, 2012; Ruiz-Bermejo et al., 2013a). The amount and type of UV radiation that arrives at a predetermined planetary zone depend on several factors (the dispersion, transmittance, and albedo of the environment), and it is an important constraint to take in consideration in the astro/exobiological exploration of the icy objects of solar systems (Cessateur et al., 2016). Ice is a highly dispersive environment and reflects UV radiation; thus, the presence of an icy layer notably reduces the UV radiation incident on the liquid water of the ocean, and it has been demonstrated that sea ice acts as a photochemical microreactor, where 85% of the reactions are produced in the upper zone of the ice-water column (King et al., 2005). Thus, in a dual manner, the freeze matrix might protect bioorganics from photodestruction and provide an ideal environment for the diversity of organics synthesized via photochemical reactions (Perovich, 2002). In addition, it has been proposed that salts could protect amino acids (McLaren and Shugar, 1964) and nucleobases (Cleaves and Miller, 1998) from photodegradation by UV radiation. However, to the best of our knowledge, the effect of salts on an irradiated freeze matrix has not yet been studied.
Thus, the main goal of this work is to explore the effect of the water freeze-thaw cycles, UV-radiation and salts on ammonium cyanide polymerization, as a step-by-step approach to understand the possible organic chemistry of the icy moons of the Solar System.
Materials and Methods
Synthesis of Cyanide Polymers
Fifty milliliters of NH4CN solution (1 M, NaCN + NH4Cl in equimolar amounts) in ultra-pure, degassed water (experiment 3, Table 1) or degassed saline solution (experiment 6, Table 1) was frozen at −25°C in a sealed and thermostated glass reactor (Menor-Salván et al., 2008, 2009; Menor-Salvan and Marin-Yaseli, 2013) under an inert atmosphere of argon of the maximum purity available (99.9995%, Praxair SA, Spain). Once the reactor was filled, freeze–thaw cycles were established by varying the temperature between −25 and 4°C (2 h at −25°C, ramping to 4°C at 0.1°C min−1, and 2 h at 4°C) by using a Haake Phoenix II programmable cryostat (Thermo Electron Corporation). The system was irradiated with UV radiation using a Spectroline 11SC-1 Hg vapor discharge lamp (Menor-Salvan and Marin-Yaseli, 2013). The irradiation was performed for 72 h. After this period, the lamp was disconnected, and the reactor was kept sealed, which then underwent active freeze–thaw cycles for 30 days. Three days of irradiation was adopted to compare with previous works (Menor-Salván et al., 2008, 2009; Menor-Salvan and Marin-Yaseli, 2013). For a detailed scheme of the reactor please see the supplementary information of Menor-Salvan and Marin-Yaseli (2013). In the present case Ar was used to fill the reactor. The system was then allowed to warm to room temperature. The resultant reaction mixtures were centrifuged at 12,000 rpm for 10 min and washed with water (4x). The supernatants and the black pellets (insoluble NH4CN polymers) were collected and freeze-dried. The freeze-dried supernatants were fractionated using Nanosep® centrifugal devices (Pall, Life Sciences) to retain molecules above 3 kDa (soluble NH4CN polymers). Finally, brown solids (soluble NH4CN polymers) and black solids (insoluble NH4CN polymers) were obtained. The dried samples were stored at −80°C under an inert atmosphere until analysis. The composition of the saline solutions was as follows: 0.63 M NaCl, 0.18 M MgCl2, 0.015 M CaCl2, 0.015 M KCl, and 0.02 M NaHCO3. This salty composition was chosen for comparison with previous results (Marín-Yaseli et al., 2016, 2017, 2018) since the actual composition of the icy oceans of the Solar System is not yet fully known. Other freeze-thaw cycle experiments (1–2 and 4–5) were performed under the same conditions without UV radiation activation. Experiments 7–14 were performed using the same reactor in analogous manner but without freeze-thaw cycles, using the temperatures reported in Table 1.
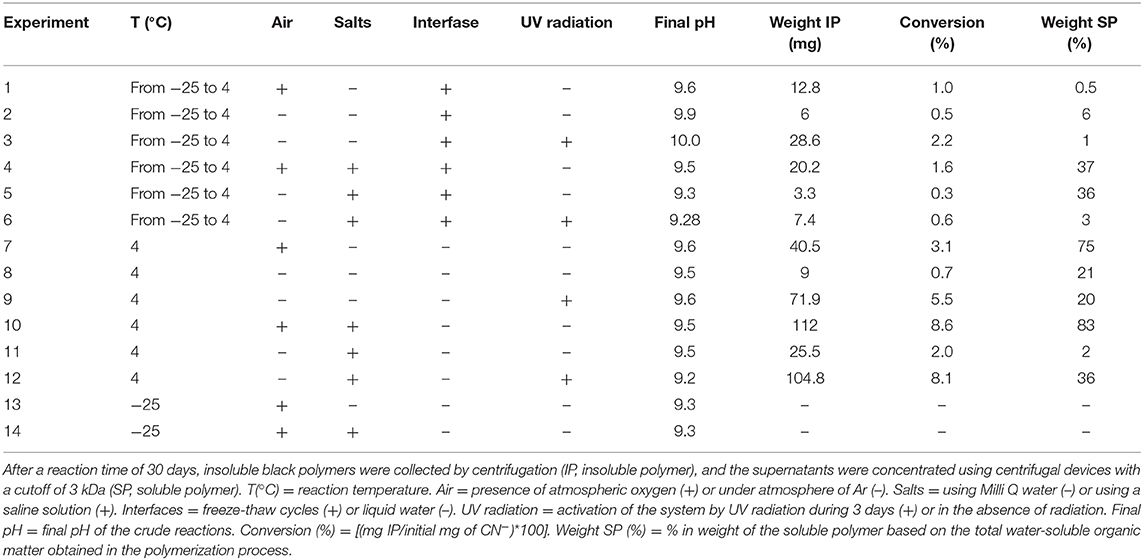
Table 1. Experimental conditions used to study the effects of ice-water interfaces, UV-radiation and salts on ammonium cyanide polymerization.
Note that a 1 M concentration of cyanide is considered a really high concentration in any plausible prebiotic scenario. However, in hydrothermal systems a continuous production of HCN and NH3 can be assumed providing a high disponibility of these prebiotic reagents (Villafañe-Barajas et al., 2020). Herein, as just a approach to the plausible chemistry of the icy moons and in order to check the effect of the ice-water interfaces, and other experimental variables, this apparent high concentration was choosen with comparative purpose respect our own previous reports, knowing that this concentration leads to a successful polymerization of cyanide. The oligomerization/polymerization of cyanide is always carried out at concentrations >0.01 M, because if the concentration is minor the hydrolysis will be dominant against polymerization, while in the eutectic point this effect is not observed (Ferris et al., 1978). Therefore, in spite of 1 M can seem a high concentration, there are mechanisms that may secure the right concentration for the oligomerization/polymerization of cyanide. Other aspect is the influence of the concentration in the kinetic and pathways of formation of the cyanide polymers, but this issue is out the scope of the present paper.
Instrumental Analyses
FT-IR Spectroscopy
Diffuse reflectance spectra were acquired in the spectral region of 4,000–400 cm−1 using an FT-IR spectrometer (Nicolet, model NEXUS 670) configured with a drift reflectance accessory (Harrick, model Praying Mantis DRP) mounted inside the instrument compartment. The spectra were obtained in CsI pellets, and the spectral resolution was 2 cm−1.
Gas Chromatography-Mass Spectrometry (GC-MS)
GC-MS analyses in the full-scan mode were performed using a 6,850 network GC system coupled to a 5,975 VL MSD with a triple-axis detector operating in electronic impact (EI) mode at 70 eV (Agilent) using an HP-5 MS column (crossbond 5% diphenyl-95% dimethyl polysiloxane, 30 m × 0.25 mm i.d. × 0.25 μm film thickness) and He as the carrier gas.
Analytical Procedure
For the identification of polar organic molecules in the NH4CN polymers, the following steps were taken. (i) The samples were hydrolyzed with 6 M HCl at 110°C for 24 h and then freeze-dried to remove water, HCl and any volatile organics. (ii) Two milligrams of each hydrolyzed sample in 75 μL of BSTFA with 1% TMCS [N,O-bis(trimethylsilyl)trifluoroacetamide with trimethylchlorosilane, from Thermo Scientific] was heated at 70°C for 19 h to obtain the respective TMS derivatives. (iii) The derivatized samples were analyzed by GC-MS using the following GC oven program: 60°C (initial temperature) with a hold time of 1.5 min, heated to 130°C at 5°C/min with a hold time of 11 min, heated to 180°C at 10°C/min with a hold time of 10 min and heated to 220°C/min at 20°C/min with a final hold time of 15 min. One microliter of each sample was injected. The temperature of the injector was 275°C, and the injections were performed in splitless mode. The detector temperature was 300°C. The flow rate was 1.1 mL/min. As a rule, the identification of the GC-MS peaks attributed to organic compounds was verified by comparison with the retention times and mass spectra of external standards (purchased from Sigma-Aldrich and Fluka). The detection limit was about 5–10 ppm depending on the analyte.
The multiple point external standard method, using standard solutions from 10 to 100 ppm, was used for the quantification of glyoxylic acid as it is detailed in Mompeán et al. (2019).
Statistical Analysis: Multivariate Analysis
Three principal component analyses (PCAs) of the relative proportion of the number of the diverse amino acids, carboxylic acids, N-heterocycles identified, and the total number of organics identified in every experiment were performed. They were separately calculated based on the soluble and insoluble polymer data and the sets of both polymers for each experiment. Furthermore, for each case, using the variables (proportion of amino acids, carboxylic acids, N-heterocycles, and the total number of organics identified), hierarchical dendrograms based on the Euclidean squared distance method were calculated. PCA was performed using the multivariate data analysis software CANOCO 4.5 (Microcomputer Power, Ithaca, NY, USA) (Braak and Smilauer, 2002). The program CANODRAW 4.0 (in the Canoco package) was used for graphical presentation. The hierarchical dendrograms were obtained using the IBM SPSS Statistics 23 package.
Results
To study the effect of ice-water interfaces on the ammonium cyanide polymerization, a set of reactions under different conditions were performed (Table 1). The experiments at 4 and −25°C are control experiments to determine the effect of the freeze-thaw cycles on the polymerization process. Although previous works showed effective oligomerization-polymerization of ammonium cyanide at temperatures below 0°C (Schwartz et al., 1982; Levy et al., 1999, 2000; Miyakawa et al., 2002), in the cases presented here, at −25°C, no reactions were observed, using a reaction time of 1 month, as confirmed by FT-IR spectroscopy (data not shown; the rest of the FT-IR spectra are included in supplementary information section).
Yields of Insoluble and Soluble Polymers
In all experiments listed in Table 1, water-insoluble solids (insoluble HCN polymers) and brown solutions after the centrifugation of the reaction crudes were collected. The water-soluble brown solids obtained after freeze-drying were concentrated via ultrafiltration by using centrifugal devices with a cutoff of 3 kDa (soluble HCN polymers). The insoluble HCN polymers were considered the final product of the reactions, while the soluble HCN polymers were understood to be intermediate products in the polymerization process (Marín-Yaseli et al., 2017).
In the case of the insoluble polymers collected, generally, the conversion (%) values were greater in the polymerizations performed at 4°C than in the syntheses using freeze-thaw cycles (Table 1). Activation of the system by UV-radiation increased the production of the insoluble polymers compared to the analogous non-irradiated reactions, as expected (Sánchez et al., 1967). The effect of the presence of salts in solution during the polymerization process is clear in the experiment performed at 4°C (experiments 7-12, Table 1). The saline solutions increased the conversions for the insoluble polymers, as expected (Marín-Yaseli et al., 2018). However, this fact was not observed in experiments 1–6 (Table 1).
In the case of soluble polymers, as in the case of insoluble polymers, the freeze-thaw cycles do not favor the polymerization process (Table 1), obtaining a greater percent of soluble polymers in the syntheses performed at 4°C, probably due to the kinetic effect of the temperature (Fernández et al., 2018). However, the effect of UV-radiation was not regular, and it was opposite that observed in the freeze-thaw experiments in terms of the insoluble polymers, i.e., the UV-radiation decreased the yields of soluble polymers, but in the experiments at 4°C, the yield of soluble polymers was similar or greater compared to the non-irradiated reactions. In contrast, the presence of salts increased the production of soluble polymers in most of the experiments (Table 1).
Therefore, for the insoluble polymers, there is an anticorrelation between the conversion value and freeze-thaw cycles, but there is a positive correlation with the presence of salts in the reaction environment and with the UV radiation. In contrast, for the soluble polymers, their relative percentage by weight presented a positive correlation with the presence of salts and air, a negative correlation with the freeze-thaw cycles and a negative correlation with the presence of UV radiation.
Thus, it is shown that the behavior of the system for the production of insoluble NH4CN polymers is different in terms of the manner of formation of the soluble polymers, taking into account the same experimental parameters.
GC-MS Analyses
Due to the differences observed in the previous section, the insoluble and soluble HCN polymers were studied separately, in a previously reported manner (Marín-Yaseli et al., 2016), to explore the relationship between the degree of polymerization and/or cross-linking and the experimental conditions assayed with the monomeric diversity present in the different samples synthetized. Thus, the insoluble and soluble NH4CN polymers were analyzed via GC-MS using BSTFA as a derivatization reagent to obtain the respective TMS derivatives of polar compounds, such as amino acids, carboxylic acids and several N-heterocycles. This derivatization method is not specific for each type of compound mentioned, but for comparative purposes, it provides an excellent general overview of polar molecules with biological interest present in all polymers synthesized. This analytical methodology allows discrimination among the various synthesis conditions tested to determine which are the most favorable from the point of view of the prebiotic production of polar bioorganics (Marín-Yaseli et al., 2016; Mompeán et al., 2019). Another important question is the hydrolysis conditions used previous to the GC-MS analysis. It is well-known that the identification of biorganics from HCN polymers depends directly on the hydrolysis method used (e.g., Miyakawa et al., 2002; Marín-Yaseli et al., 2015). In the present case, acid hydrolysis conditions were used because they are a standard method for screening for a wide variety of polar bioorganics present in complex substances, such as tholins, HCN polymers and carbonaceous materials present in meteorites (Miller and Cleaves, 2006; Burton et al., 2012). Figure 1 shows all of the analytes identified in this work.
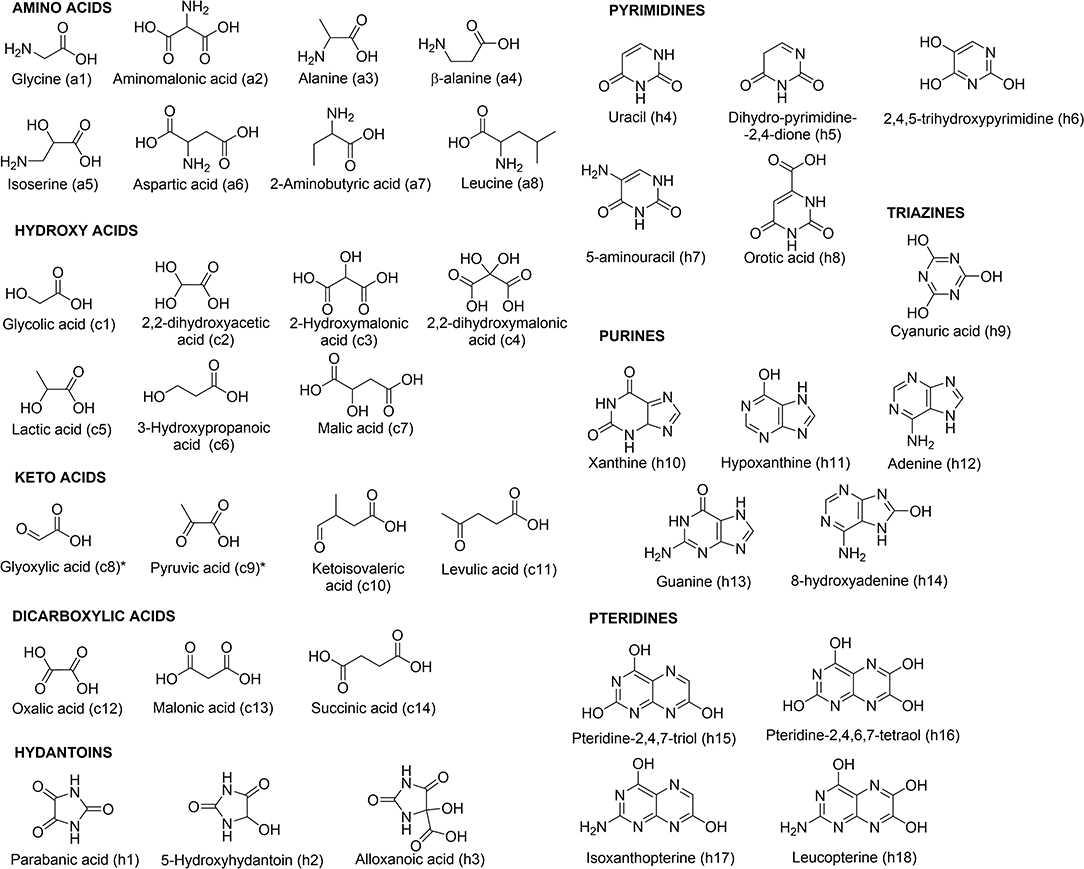
Figure 1. Polar organic compounds identified as their TMS derivatives by GC-MS in insoluble and soluble NH4CN polymers, synthetized as detailed in Table 1. *Glyoxylic acid and pyruvic acid were identified as their oxime-TMS derivatives. The formation of the corresponding oximes is explained in Marín-Yaseli et al. (2016). Some of the compounds represented can be in different tautomeric form. In the particular case of pteridines only the tautomer assigned to the TMS-derivative identified by GC-MS was represented (Marín-Yaseli et al., 2016). For the rest of the analytes the tautomer form provided by ChemDraw Professional 15.0 is represented.
GC-MS Analyses of Insoluble NH4CN Polymers
The results of the qualitative GC-MS analyses of the acid-hydrolyzed insoluble polymers 1–12 are shown in Figure 2. Only the amino acid glycine (a1) was identified in all of the insoluble polymers synthetized. In contrast, isoserine (a5), 3-hydroxypropanoic acid (c6), and 8-hydroxyadenine (h14) were only identified under unique reactions conditions. The carboxylic acids malic acid (c7), pyruvic acid (c9), and succinic acid (c14), all of them forming part of the r-TCA cycle, were simultaneously identified in the insoluble polymers 3, 4, 6, 9, and 12, indicating that the presence of UV radiation favors the formation of these relevant compounds needed for the emergence of a plausible protometabolism (Morowitz et al., 2000). Additionally, the identification of glyoxylic acid (c8) in insoluble polymers 1 and 6 is interesting. These results extend the experimental conditions that can lead to the prebiotic production of glyoxylic acid (c8) (Menor-Salvan and Marin-Yaseli, 2013; Marín-Yaseli et al., 2016; Mohammed et al., 2017; Mompeán et al., 2019) and may be interesting for the further development of the “glyoxylic acid scenario” proposed by Eschenmoser (2007), as will be discussed later. The yields of glyoxylic acid (c8) for the experiments 1 and 6 were 1.1·10−4 % (13 mg/L) and 9.5·10−4 % (169 mg/L), respectively. The yields were calculated from the total amount of the initial carbon input in the system. These yields are comparable with the previous yields obtained for pteridines and purines identified in the insoluble NH4CN polymers from the polymerization of NH4CN using liquid water at 38°C or using aqueous aerosols (Marín-Yaseli et al., 2015) but lower than the yield reported for glyoxylic acid synthetized using spark discharges and alkaline gas-liquid interfases (Mompeán et al., 2019). Indeed, in the insoluble polymer 6, a great diversity in N-heterocycles was observed. These results seem to indicate that polymerization of cyanide in saline water-ice interfaces in the presence of UV-radiation exhibits favorable conditions for the emergence of a plausible prebiotic chemistry.
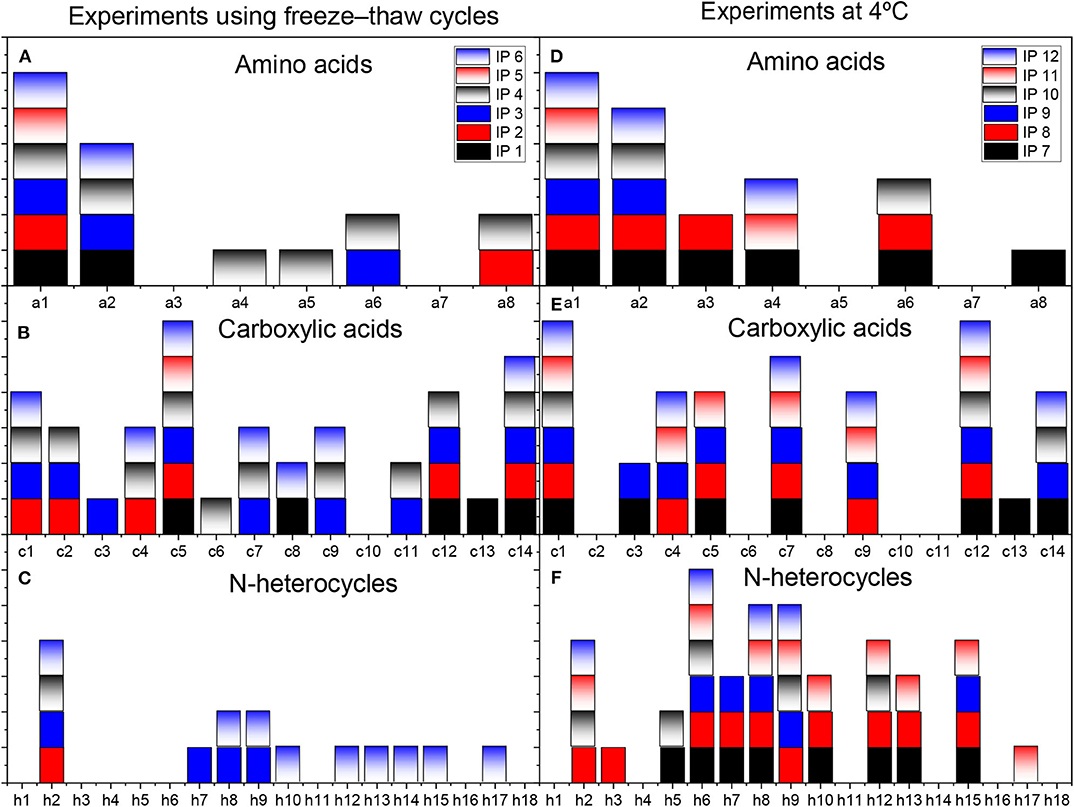
Figure 2. Organic polar compounds identified as their TMS-derivatives by GC-MS in acid-hydrolyzed insoluble NH4CN polymers synthesized under the conditions indicated in Table 1. (A–C) Correspond to the amino acids, carboxylic acids, and N-heterocycles, respectively, found in insoluble NH4CN polymers synthesized in the presence of freeze-thaw cycles; (D–F) correspond to amino acids, carboxylic acids, and N-heterocycles, respectively, found in insoluble NH4CN polymers synthetized at 4°C. The numbers on the abscissa correspond to the enumeration of analytes shown in Figure 1. The colored bars indicate the presence of a concrete analyte in the corresponding sample.
In addition, statistical methods were used for a better comparison of the analytical results shown in Figure 3. PCA, performed on these GC-MS analytical results, found that 99% of the total variance could be explained with three components (Figure 3A). In this case, for the insoluble polymers, hierarchical dendrogram revealed three clusters (Figure 3B). The first cluster corresponds to the insoluble polymers 6, 7, 8, and 11, since they appear grouped. In the case of the PCA plot, this cluster is also clear, but insoluble polymer 7 is slightly ungrouped (Figures 3A,B). In fact, the insoluble polymers 6, 8, and 11 presented the major positive correlation of N-heterocycles, and all of them were obtained in the absence of air. In the hierarchical dendrogram, these four samples were grouped because these insoluble polymers exhibited the highest monomeric diversity obtained, but the N-heterocycles efficiency in insoluble polymer 7 was lower, in strong agreement with the proposal made by Ferris and Edelson (1978) about oligomerization of HCN. The second cluster grouped insoluble polymers 3, 9, and 4. This cluster is not as clear in the PCA plot. The third cluster grouped insoluble polymers 1, 2, 10, and 12. In this case, the PCA analysis (Figure 3A) and the hierarchical dendrogram (Figure 3B) revealed same grouping, where insoluble polymers 1 and 2 and 10 and 12 were more similar to each other. These four polymers exhibited negative correlations with the total number of organics identified (Figure 3A). It is especially remarkable that both insoluble polymers 1 and 2 were synthesized using a water-ice interface without salts and UV radiation. It is interesting that the final pH in both experiments was low. Insoluble polymer 5 was ungrouped in both analyses (Figure 3), showing clearly that it was the polymer that leads to the lowest number of organics identified.
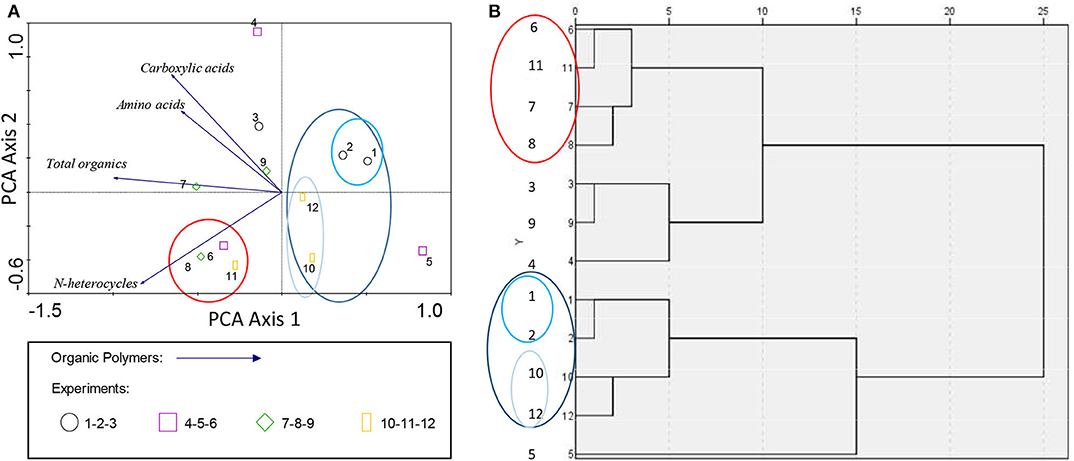
Figure 3. (A) PCA plot based on the relative proportion of amino acids, carboxylic acids, N-heterocycles, and total organics obtained in every experiment for the insoluble polymers. Relative proportions of the different compounds are indicated by arrows. The observed clusters are indicated by circles. (B) Hierarchical dendrograms calculated using PCA components. Experiments are represented by their numbers. The obtained clusters are designated by circles.
GC-MS Analyses of Soluble NH4CN Polymers
The GC-MS analytical results for the soluble polymers were studied in the same manner as the insoluble polymers, as discussed above. Oxalic acid (c12) was identified in all soluble polymers identified, while 2-aminobutyric acid (a7), 2,2-dihydroxyacetic acid (c2), ketoisovaleric acid (c10), alloxanoic acid (h3), uracil (h4), and leucopterine (h18) were identified in only soluble polymers synthesized under particular reaction conditions (Figure 4). Note that some compounds identified in the soluble polymer series were not identified in the insoluble polymers series, and vice versa. In this case, the carboxylic acids malic acid (c7), pyruvic acid (c9), and succinic acid (c14) were simultaneously identified in the soluble polymers 1, 3, 7, and 8. No significant relationship between the experimental conditions used and the identification of these carboxylic acids was found. On the other hand, for the soluble polymers synthesized in the presence of freeze-thaw cycles, the negative influence of the presence of salts and oxygen during the polymerization process on the identification of N-heterocycles in the acid-hydrolyzed residues (Figure 4E), in agreement with previous results (Marín-Yaseli et al., 2017), is notable.
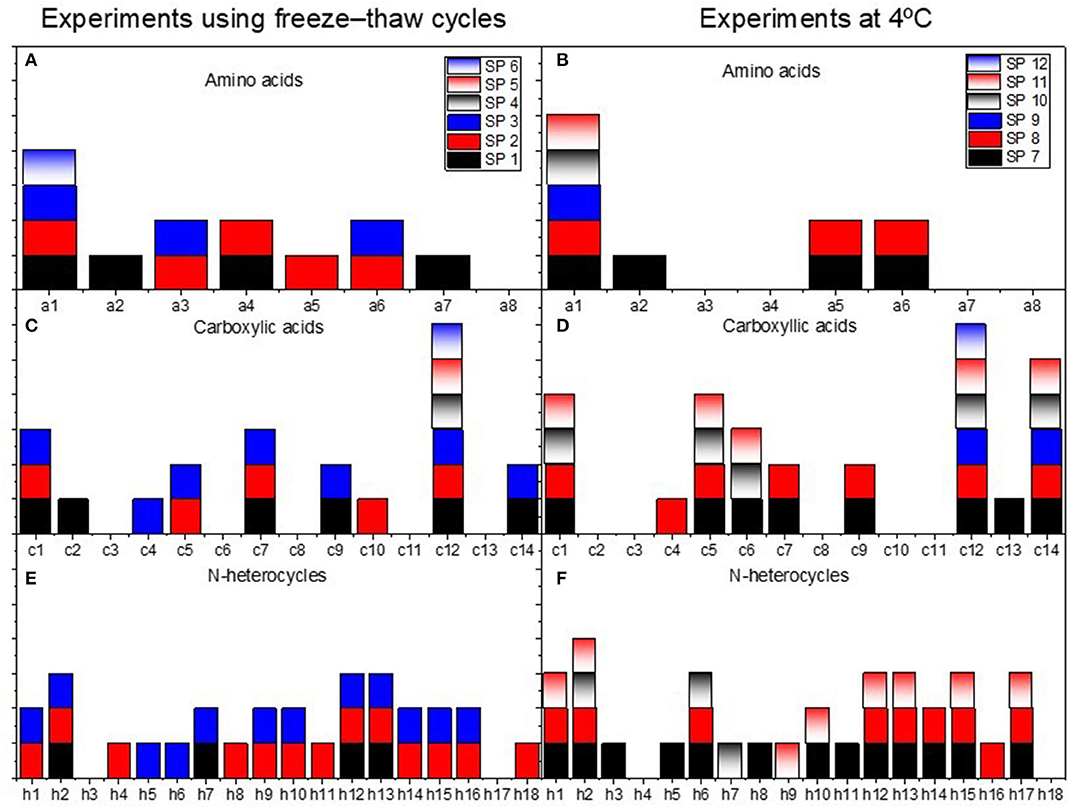
Figure 4. Organic polar compounds identified as their TMS-derivatives via GC-MS in acid-hydrolyzed soluble NH4CN polymers synthesized under the conditions indicated in Table 1. (A–C) Correspond to the amino acids, carboxylic acids, and N-heterocycles, respectively, found in soluble NH4CN polymers synthetized in the presence of freeze-thaw cycles; (D–F) correspond to amino acids, carboxylic acids, and N-heterocycles, respectively, found in soluble NH4CN polymers synthetized at 4°C. The numbers on the abscissa correspond to the enumeration of analytes shown in Figure 1. The colored bars indicate the presence of a concrete analyte in the corresponding sample.
For the soluble polymers, PCA (Figure 5A), in addition to the hierarchical dendrogram (Figure 5B), showed two major clusters among the experiments. One cluster contained the soluble polymers 4, 5, 6, 9, and 12. Furthermore, the PCA plot showed a negative correlation between these experiments and the monomeric diversity found (total number of individual organics identified by GC-MS in each sample) for these soluble polymers, indicating that they were the soluble polymers with fewer amino acids, carboxylic acids and N-heterocycles identified (Figure 5A). In this cluster, soluble polymers 4, 5, and 12 were strongly grouped, and nearby is the soluble polymer 6 (Figure 5A). The factor common to all these was the use of saline solution. The second cluster grouped experiments 3, 7, 8, 10, and 11, with experiments 3 and 7 being more strongly grouped. These two experiments exhibited positive correlations with the total number of organics identified, especially with carboxylic acids and N-heterocycles (Figure 5A). The factor common to both experiments was that they were performed without saline solutions. Soluble polymers 1 and 2 were ungrouped in both analyses (Figure 5). It is interesting to note the strong correlations between soluble polymer 2 and the N-heterocycles and the total number of organics identified in this polymer. This experiment was performed in the absence of saline solution. Therefore, if the analytical results for soluble polymers are considered, the most favorable conditions to obtain greater monomeric diversity are a water-ice scenario free of salts in the presence of UV radiation.
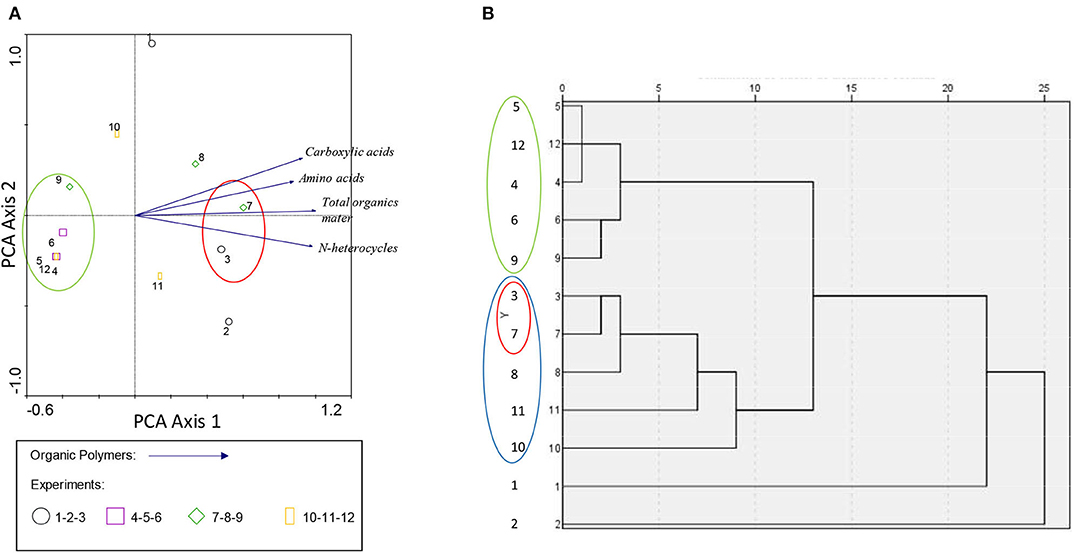
Figure 5. Soluble polymers statistical analysis. (A) PCA plot based on the relative proportions of amino acids, carboxylic acids, N-heterocycles, and total organic obtained in every experiment for the soluble polymers. The relative proportions of the different compounds are indicated by arrows. The observed clusters are indicated by circles. (B) Hierarchical dendrograms calculated using PCA components. Experiments are represented by their numbers. The obtained clusters are designated by circles.
In summary, considering the results shown in Figures 3A, 5A, the experimental conditions of control experiments 7 and 8 lead to greater monomeric diversity. However, the presence of water-ice interfaces and UV radiation (experimental conditions 3 and 6) seems to provide the most favorable conditions for the production of carboxylic acids implicated in the r-TCA cycle and for the production of several N-heterocycles, with the role of saline solutions being truly ambiguous. Thus, a joint study of the organics identified from soluble and insoluble polymers is needed to obtain a good overview of the conditions that lead to greater monomeric diversity as a result of cyanide polymerization under plausible planetary conditions.
Global Analysis of the Monomeric Diversity of NH4CN Polymers With Respect to the Experimental Synthetic Conditions
Because the global interpretation of the analytical results discussed above is difficult due to the different correlations found between the monomeric diversity and the experimental conditions for the insoluble and soluble polymers, additional statistical analyses were performed. In this case, for each of the 12 experimental conditions studied, the set of organics identified in the soluble polymer and the organics identified in the insoluble polymers were considered. The total data from PCA (Figure 6) and the hierarchical dendrogram revealed two major clusters among the experiments, including one heterogeneous cluster containing experiments 1, 4, 6, 9, 10, 11, and 12, which exhibited a negative correlation with the total number of organics obtained (which is not very clear in experiments 6 and 11). It is interesting to note that experiments 10 and 12 yielded the lower total number of organics identified. Both experiments were performed in the presence of saline solutions at 4°C, and the resulting crude reactions yielded higher final pH values. The second cluster strongly grouped experiments 2, 3, 7, and 8. All of them showed positive correlations with the total number of organics identified (Figure 6A). The common factor between all these was the absence of saline solutions. Therefore, it is now clear that the presence of saline solutions, as here used, is not favorable to identify polar organic compounds from the polymerization of cyanide, although it increases the global yield of NH4CN polymers. This lack of a clear role of salts was also reported previously by Cleaves et al. (2006) in the context of cyanoacetylene polymerization in eutectic solutions. On the other hand, experiment 5 was ungrouped in both analyses (Figure 6), clearly showing that it was the experiment with the lowest number of organics identified. It is interesting to note that three PCA plots (Figures 3A, 5A, 6A) indicated that experiment 5 was, in all cases, the most inefficient. In contrast, when experiment 5 was modified to include irradiation (experiment 6), the number of organics identified was notably greater, and experiment 3 presented one of the greatest amounts of monomeric diversity observed in this work. However, experiments 9 and 12, which also included UV radiation, presented a low diversity of organics (Figure 6A), indicating that water-ice interfaces play an important role of protecting against photodestruction and/or provide better conditions for prebiotic chemistry than only liquid water, when cyanide is used as the main reactant, in agreement with the proposal indicated in the introduction section.
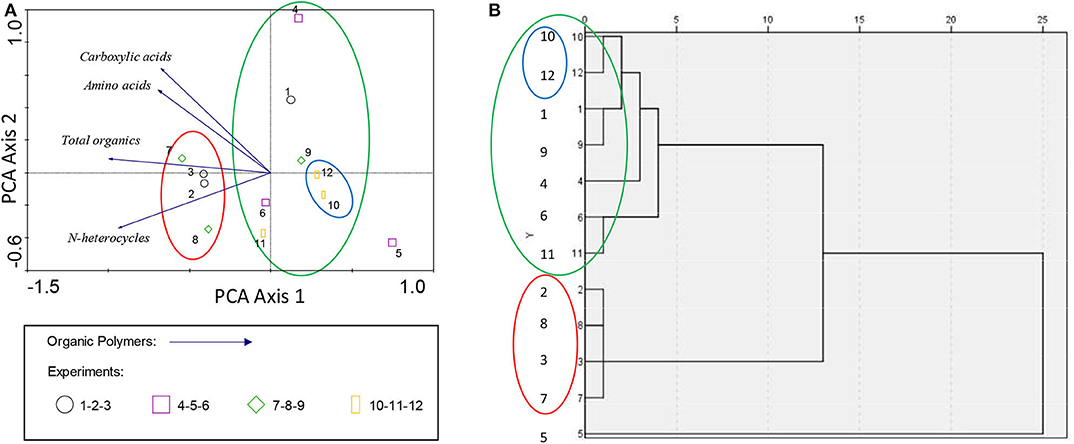
Figure 6. Total polymers statistical analysis. (A) PCA plot based on the relative proportions of amino acids, carboxylic acids, N-heterocycles, and total organic obtained in every experiment for the full polymers. Relative proportions of the different compounds are indicated by arrows. The observed clusters are indicated by circles. (B) Hierarchical dendrograms calculated using PCA components. Experiments are represented by their numbers. The obtained clusters are designated by circles.
To understand the plausible complex organic chemistry of icy systems, these results are truly interesting. Experiments 2 and 3 yielded the greatest monomeric diversity, after the control experiment 7, indicating that water-ice interfaces can serve as suitable chemical reactors in a planetary scenario. Focusing on a more exhaustive analysis of these interfacial experiments, Figure 7 shows in detail the variation in the monomeric diversity present in NH4CN polymers when a new experimental condition is added in each step. Thus, it is easy to see that the presence of UV radiation does not favor the production of amino acids but increases the diversity in carboxylic acids and provides the identification of pyrimidines. These results will be discussed later.
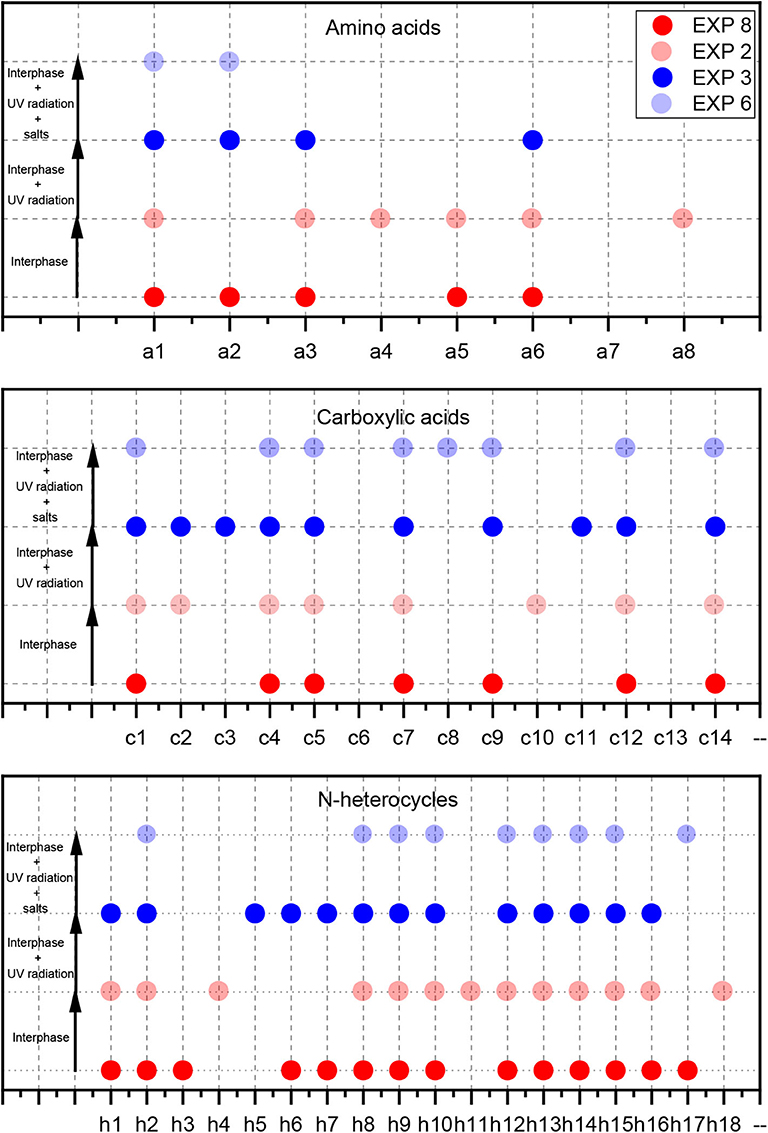
Figure 7. Comparison of the experimental conditions against analytes found by GC-MS for the particular case of the experiments performed in the presence of water-ice interfaces. Note that in each step, a new variable is added. The analytes represented correspond to those identified in both the insoluble and soluble polymers.
Discussion
In most of the previous works reported in the bibliography that performed chromatography analysis of HCN polymers, only the soluble or insoluble fractions of the total material obtained were analyzed (Ruiz-Bermejo et al., 2013b). Here, it is shown that for a qualitative analysis of the monomeric diversity present in NH4CN polymers, it is necessary to explore both the insoluble and soluble polymers because otherwise, important information would be missed. As was observed above, the statistical results are strongly dependent on the part of the NH4CN system analyzed. Therefore, for a comprehensive understanding of the organic complex samples prepared in the lab, as simulations of planetary environments, a first fractionation of the crude reactions is required to obtain better analytical results by chromatographic techniques (e.g., Miyakawa et al., 2002; Marín-Yaseli et al., 2016) using those methods that enable identification of a greater number of analytes in one step. Then, it is necessary to consider the analytical results as a whole for each sample previously subfractionated. In addition, the GC-MS results presented herein represent plausible icy planetary conditions, with this technique being the most widely used analytical method for in situ investigation of organic molecules in space environments; as recently demonstrated, it can be applied to study the space environment of Jupiter's icy moons (Freissinet et al., 2016). Thus, the present analytical results may aid in understanding the possible organic chemistry on the icy moons of the Solar System.
To the best of our knowledge, there are few works that explore polymerization of NH4CN below 0°C (Schwartz et al., 1982; Levy et al., 1999, 2000; Miyakawa et al., 2002), and they are generally focused on identification of purines and pyrimidines. Schwartz et al. (1982) described the production of adenine, Levy et al. (1999) discussed the formation of the adenine and guanine, and Miyakawa et al. (2002) identified a set of eleven purines and pyrimidines, the yields of which are dependent on the hydrolysis conditions. Only Levy et al. (2000) jointly described the formation of amino acids and purines. Here, it is shown for the first time that it is possible to obtain amino acids, hydroxy acids, carboxylic acids, hydantoins, pyrimidines, triazines, purines, and pteridines using NH4CN and water-ice interfaces even in the presence of UV radiation. It is certain that a suitable and comprehensive comparison between the results reported here, the results of the commented previous works and the unique effect of the icy matrix is not possible since, on one hand, our control experiments using a reaction time of 1 month using only icy water did not lead to positive polymerization reactions, and in previous works, longer reaction times were used to test the concentrator effect of the eutectic point (from 2 months to 27 years), since it was proved that cyanide oligomers production takes place in preference to hydrolysis when 0.01 M solutions of cyanide are cooled to −23.4°C (Sánchez et al., 1966). On the other hand, there is a bias in the previous chromatographic analyses reported in the bibliography. However, in any case, herein, it is demonstrated that water-ice interfaces provide suitable niches for the prebiotic formation of organics of biological interest even in the presence of UV radiation when NH4CN is used as the main reactant. In addition, it is necessary to note that the presence of UV-vis radiation would favor the formation of radicals during the periods in which the system is in the liquid phase and therefore, the formation of some organic molecules, as previously indicated. During the formation of the ice-liquid water interfaces or at times when only ice is present, these icy layers would protect the organic molecules from photodestruction, because the ice does not let UV penetrate significantly beyond 200 nm (Cruz-Diaz et al., 2014). Therefore, we would only see a beneficial effect of UV-vis radiation in relation to the production of organic molecules, from cyanide, in the presence of freeze-thaw cycles because the effects of photoproduction prevail in the presence of liquid water, and the effects of UV-vis radiation only be evident in the first 200 nm of the frozen layer. Thus, the effect of the UV radiation only would be effective in the surface of the icy moons. However, one might establish a transport of in/organic materials between the liquid subsurface and the frozen surface owing to the presence of the plumes observed on Enceladus and potentially on Europa (e.g., Hansen et al., 2020; Ingersoll et al., 2020; Pasek, 2020; Quick and Hedman, 2020). In this way, one chemistry might be observed in the water-ice interfaces of the subsurfaces and the other one in the water-ice interfaces of the frost layer of the surface. In both cases, the presence of water-ice interfaces would create a richer diversity in polar organic compounds than only liquid water.
Amino Acids
The pathway of production of amino acids from NH4CN is not yet well-established. However, it has been proposed that the trimer of HCN or its tetramer, aminomalononitrile (AMN), and diaminomaleonitrile (DAMN), respectively, might be involved in the synthesis. Studies regarding the formation of amino acids from AMN and DAMN were conducted by Sánchez et al. (1966, 1967), and Moser et al. (1968a,b) showed that DAMN formation is accelerated by lowering the temperature from 25 to −22°C when diluted solutions of HCN were used. The greater diversity in amino acids observed in the NH4CN polymers observed in experiments 2 and 4, in which water-ice interfaces were used, is in agreement with these previous results. The lower temperatures favored the initial formation of DAMN since the eutectic phase of an aqueous HCN solution, which occurs at −23.4°C, contains 74.5% HCN (25 M), and the cyanide concentration is so much greater that oligomer formation proceeds at an appreciable rate (Sánchez et al., 1966) and therefore might improve the formation and diversity of amino acids in these two experiments.
In a pioneering work, Abelson (1966) showed the production of glycine, alanine, aspartic acid and serine using diluted solutions of HCN at pH 8–9, UV (254 nm) radiation and working at room temperature. The relative yields of the amino acids depended on the time of irradiation. With short irradiation times, the yields were low, and glycine was prominent. With longer times, the yields were better, and larger amino acids were more prominent. At very long times, the yields decreased. However, these times of irradiation were not detailed. In the present case, the diversity in amino acids found in experiments 2 and 3 and were lower than those found in experiments 4 through 6, indicating that 3 days is a long time for irradiation and that our results are in agreement with those reported by Abelson (1966).
Independently on the pathway of formation, the identification of amino acids from cyanide polymerization under different plausible prebiotic conditions is astrobiologically relevant since the finding of extraterrestrial biosignatures is a no trivial issue. Thus, it has been discussed that if amino acids are detected at Enceladus, Europa, or other hydro thermally active ocean worlds, they should have been formed recently due to an active production within the ocean (Truong et al., 2019) and the discrination in space mission between abiotic and biotic amino acids demands the development of sensible and specific analytical tools (Klenner et al., 2020). The detection of amino acids under simulated extraterrestrial conditions can help to tune up these techniques.
Carboxylic Acids
The preferential formation of carboxylic acids from NH4CN when UV radiation and water-ice interfaces are present (experiment 3) is in agreement with previously reported results that showed that water-ice interfaces subject to UVC radiation are a source of reactive oxygenated compounds, which favors the syntheses of carboxylic acids (Menor-Salvan and Marin-Yaseli, 2013). The identification of those from NH4CN polymerization is very interesting from the perspective of the emergence of a primordial metabolism. Specifically, it is known that glyoxylic acid (c8) can be directly produced from hydroxyl radicals attacking glycolic acid (c1) (Eugene et al., 2016). Herein, it is shown that glyoxylic acid (c8) was found in the irradiated experiment 6, in which glycolic acid (c1) was also identified. Eschenmoser suggested a relationship between HCN and the constituents of the rTCA cycle (Eschenmoser, 2007), known as the “glyoxylate scenario,” wherein glyoxylate and its formal dimer, dihydroxyfumarate, were proposed to be the central starting materials of a chemical constitution of a possible primordial metabolism. Verification of the “glyoxylate scenario” implicates the experimental revision of the HCN chemistry (Eschenmoser, 2007). In this sense, aqueous, air-water (Marín-Yaseli et al., 2016), or water-ice interfaces favor the production of glyoxylic acid when free radical are formed during the NH4CN polymerizations. On the other hand, glyoxylic acid (c8) was also identified in experiment 1, in which UV radiation was not used. In this case, the production of glyoxylic acid (c8) may be explained from the hydrolysis of the dimer of the cyanide, HN=C-CN, in the water-ice interfase (Eschenmoser, 2007). The yields calculated for glyoxylic acid for the experiments 1 and 6 seems to indicate that the pathway through radicals is more favorable for the production of glyoxylic acid. Indeed, a large variety of dicarboxylic and tricarboxylic acids were identified as radiolytic products from cyanide solutions, being produced by free-radical-initiated chemical reactions (Negrón-Mendoza et al., 1983). All these results bring to light the role of the radicals in the generation of carboxylic acids from cyanide.
N-heterocycles
In general, the N-heterocycles identified in this work are the same as those reported from the polymerization of cyanide in the presence of aqueous aerosol or using liquid water at 38°C by GC-MS analyses using the same analytical procedure herein indicated (Marín-Yaseli et al., 2016). This fact shows the robustness of NH4CN polymerization to produce N-heterocycles, although some of them can be identified only under particular synthesis conditions. By the contrary, the diversity in amino acids seems to have a greater dependence on the experimental synthetic conditions, being higher in diversity, and yield in those cases where higher temperatures were used (Levy et al., 2000; Marín-Yaseli et al., 2016).
Experiments 2, 3, 7, and 8 present the greatest diversity in N-heterocycles (Figure 7). Again, as in the case of the amino acids, the presence of water-ice interfaces improves the diversity in N-heterocycles. This fact can be due to DAMN and its preference production when the temperature is down to eutectic point, as one can see above. DAMN has been proposed to be the main precursor implicated in the formation of pyrimidines and purines from cyanide oligomerization (e.g., Ruiz-Bermejo et al., 2013b and internal references) and AMN and DAMN have also been suggested are main intermediates in a hypothetical pathway for the production of pteridines from HCN (Marín-Yaseli et al., 2015). It is notably, that the UV radiation increases the diversity in pyrimidines (experiment 3) although in this case, uracil (h4) was not identified, as it was expected. Ferris and Joshi (1979) synthesized uracil (h4) from the UV radiation of orotic acid solutions by a decarboxylation process. From this point, Miyakawa et al. (2002) suggested that the yield of uracil from HCN could be much higher if the NH4CN polymers were exposed to UV light. In any case, the polymerization of cyanide is a not-fully resolved problem (e.g., Nandi et al., 2018) and for extension the pathway of its hydrolyzed products.
Potential Organic Chemistry in Icy Ocean Worlds
Icy environments, in particular water-ice interfaces, might be ideal places to develop a complex organic chemistry from a carbon source as simple as cyanide. In addition, this organic chemistry at interfaces is extendable to other simple carbon compounds that are likely also present on the icy moons of the Solar System, such as CH4, urea and acetylene. In the presence of water-ice interfaces, the activation of reduced atmospheres based on CH4 leads to the production of PAHs when pure water is used (Menor-Salván et al., 2008) in addition to a set of triazines, pyrimidines and purines when urea is present in solution (Menor-Salván et al., 2009). Moreover, UV irradiation of an acetylene-based atmosphere and urea solutions yields hidantoins, pyrimidines, and purines (Menor-Salvan and Marin-Yaseli, 2013). Therefore, it seems possible to find a rich organic chemistry in icy worlds and hence favorable habitability conditions for these planetary environments of our Solar System. Russell and coworkers suggested that the conditions for abiogenesis are/were met in any of the oceanic icy moons (Camprubi et al., 2019). By the contrary, Damer and Deamer (2020) indicated that these environments of the Solar System have not the capacity for life to begin. In any case, beyond of the very ambitious goals of the hypotheses about the origin of life, herein it is just showed that a potential wide organic chemistry can be produced from very simple carbon sources where the ice-liquid water interfases play an active role. Thus, more comprehensive and systematic synthetic studies are needed, considering other temperature ranges below 0°C, pressures, doses of radiation, concentrations of reactants and the presence of sulfates (due to the emplacement of endogenic sulfates on Europa's surface, Fox-Powell et al., 2019), that simulate the subsurface water oceans in liquid–solid equilibrium to design the best chromatography tools and finest analytical methods for space exploration with the objective of collecting suitable data about the possible habitability of a particular icy environment. On the other hand, interpolation of the data about the plausible organics found in these icy places together with the statistical studies performed via simulation experiments could help to interpret and understand the planetary conditions under which this plausible organic chemistry has been produced.
Data Availability Statement
The datasets generated for this study are available on request to the corresponding author.
Author Contributions
MR-B conceived and planned the experiments, supervised the data, wrote the manuscript and funding acquisition. EG-T performed the statistical calculations funding acquisition. MM-Y performed the experiments and the GC-MS analysis. All authors contributed to the article and approved the submitted version.
Funding
The authors used the research facilities of the Centro de AstrobiologCAB) and were supported by the Instituto Nacional de Técnica Aeroespacial “Esteban Terradas” (INTA), by the projects ESP2017-89053-C2-2-P and PID2019-104205GB-C22 from the Spanish Ministerio de Ciencia, Innovación y Universidades and by the Spanish State Research Agency (AEI) project MDM-2017-0737 Centro de Astrobiología (CSIC-INTA), Unidad de Excelencia María de Maeztu. MM-Y was supported by a research training grant from INTA.
Conflict of Interest
The authors declare that the research was conducted in the absence of any commercial or financial relationships that could be construed as a potential conflict of interest.
Acknowledgments
We are grateful to M. T. Fernández for recording the FT-IR spectra.
References
Abelson, P. H. (1966). Chemical events in the primitive earth. Proc. Natl. Acad. Sci. U.S.A. 55, 1365–1372. doi: 10.1073/pnas.55.6.1365
Attwater, J., Wochner, A., Pinheiro, V. B., Coulson, A., and Holliger, P. (2010). Ice as a protocellular medium for RNA replication. Nat. Commun. 1:76. doi: 10.1038/ncomms1076
Bacchus-Montabonel, M.-C. (2017). Theoretical investigation of proton collisions on prebiotic candidates: hydrogen cyanide polymers. Phys. Chem. Chem. Phys. 19:19566–19572. doi: 10.1039/C7CP00826K
Braak, C. J. F., and Smilauer, P. (2002). CANOCO Reference Manual and CanoDrawfor Window'suser's Guide: Software for Canonical Community Ordination (Version 4.5). Ithaca: Microcomputer Power.
Brainard, J. (2018). Europa's plumes gain support. Science 390, 690–690. doi: 10.1126/science.362.6413.390
Burton, A. S., Stern, J. C., Elsila, J. E., Glavin, D. P., and Dworkin, J. P. (2012). Understanding prebiotic chemistry through the analysis of extraterrestrial amino acids and nucleobases in meteorites. Chem. Soc. Rev. 41, 5459–5472. doi: 10.1039/c2cs35109a
Campagnola, S., Buffington, B. B., Lam, T., Petropoulos, A. E., and Pellegrini, E. (2019). Tour design techniques for the Europa Clipper mission. J. Guidance Control Dyn. 42, 2615–2626. doi: 10.2514/1.G004309
Camprubi, E., De Leeuw, J. W., House, C. H., Raulin, F., Russell, M. J., Spang, A., et al. (2019). The emergence of life. Spa. Sci. Rev. 215:56. doi: 10.1007/s11214-019-0624-8
Cessateur, G., Barthelemy, M., and Peinke, I. (2016). Photochemistry-emission coupled model for Europa and Ganymede. J. Space Weather Space Clim. 6:A17. doi: 10.1051/swsc/2016009
Cleaves, H. J., and Miller, S. L. (1998). Oceanic protection of prebiotic organic compounds from UV radiation. Proc. Natl. Acad. Sci. U.S.A. 95, 7260–7263. doi: 10.1073/pnas.95.13.7260
Cleaves, H. J. I. I., Nelson, K., and Miller, S. L. (2006). The prebiotic synthesis of pyrimidines in frozen solution. Naturwissenschaften 93, 228–231. doi: 10.1007/s00114-005-0073-y
Cruz-Diaz, G. A., Muñoz Caro, G. M., and Chen, Y.-J. (2014). Vacuum-UV absorption spectroscopy of interstellar ice analogues. III. Isotopic effects. Monthly Not. R. Astronom. Soc. 439, 2370–2376. doi: 10.1093/mnras/stu084
Damer, B., and Deamer, D. (2020). The hot spring hypothesis for an origin of life. Astrobiology. 20, 429–452. doi: 10.1089/ast.2019.2045
Eschenmoser, A. (2007). On a hypothetical generational relationship between HCN and constituents of the reductive citric acid cycle. Chem. Biodiver. 554–573. doi: 10.1002/cbdv.200790050
Eugene, A. J., Xia, S.-S., and Gúzman, M. I. (2016). Aqueous photochemistry of glyoxylic acid. J. Phys. Chem. A 120:3817–3826. doi: 10.1021/acs.jpca.6b00225
Fernández, A., Ruiz-Bermejo, M., and de la Fuente, J. L. (2018). Modelling the kinetic and structural properties evolution of a versatile reaction: the aqueos HCN polymerization. Phys. Chem. Chem. Phys. 20, 17353–17366. doi: 10.1039/C8CP01662C
Ferris, J. P., and Edelson, E. H. (1978). Chemical evolution. 31. Mechanism of the condensation of cyanide to hydrogen cyanide oligomers. J. Org. Chem. 43, 3989–3995. doi: 10.1021/jo00415a001
Ferris, J. P., and Joshi, P. C. (1979). Chemical evolution 33. Photochemical decarboxylation of orotic acid, orotidine, and orotidine 5'-phosphate. J. Org. Chem. 44, 2133–2137. doi: 10.1021/jo01327a020
Ferris, J. P., Joshi, P. C., Edelson, E. H., and Lawless, J. G. (1978). HCN: A plausible source of purines, pyrimidines and amino acids on the primitive earth. J. Mol. Evol. 11, 293–311. doi: 10.1007/BF01733839
Fox-Powell, M. G., Osinski, G. R., Applin, D., Stromberg, J. M., Gazquez, F., Cloutis, E., et al. (2019). Natural analogue constraints on Europa's non-ice surface material. Geophys. Res. Lett. 46, 5759–5767. doi: 10.1029/2018GL081339
Freissinet, C., Getty, S. A., Trainer, M. G., Glavin, D. P., Mahaffy, P. R., McLain, H. L., et al. (2016). Evaluation of the robustness of chromatographic columns in a simulated highly radiative Jovian environment. Planet Spa. Sci. 122, 38–45. doi: 10.1016/j.pss.2016.01.004
Graham, J. D., and Roberts, J. T. (2000). Chemical reactions of organic molecules adsorbed at ice: 2. Chloride substitution in 2-methyl-2-propanol. Langmuir 16, 3244–3248. doi: 10.1021/la9906166
Grasset, O., Dougherty, M. K., Coustenis, A., Bunce, E. J., Erd, C., Titov, D., et al. (2013). JUpiter ICy moons Explorer (JUICE): an ESA mission to orbit Ganymede and to characterise the Jupiter system. Plan Spa. Sci. 78, 1–21. doi: 10.1016/j.pss.2012.12.002
Hansen, C. J., Esposito, L. W., Colwell, J. E., Hendrix, A. R., Portyankina, G., Stewart, A. I. F., et al. (2020). The composition and structure of Enceladus' plume from the complete set of Cassini UVIS occultation observations. Icarus 344:113461. doi: 10.1016/j.icarus.2019.113461
Hartig, K.-.J., and Getoff, N. (1980). Photolysis of sodium cyanide in aqueous solution. J. Photochem. 13, 207–213. doi: 10.1016/0047-2670(80)85090-8
Holm, N. G., and Neubeck, A. (2009). Reduction of nitrogen compounds in oceanic basement and its implications for HCN formation and abiotic organic synthesis. Geochem. Trans. 10:9. doi: 10.1186/1467-4866-10-9
Huber, C., Kraus, F., Hanzlik, M., Eisenreich, W., and Wachtershauser, G. (2012). Elements of metabolic evolution. Chem. Eur. J. 18, 2063–2080. doi: 10.1002/chem.201102914
Ingersoll, A. P., Ewald, S. P., and Trumbo, S. K. (2020). Time variability of the Enceladus plumes: orbital periods, decadal periods, and aperiodic change. Icarus 344:113345. doi: 10.1016/j.icarus.2019.06.006
Jia, X. Z., Kivelson, M. G., Khurana, K. K., and Kurth, W. S. (2018). Evidence of a plume on Europa from Galileo magnetic and plasma wave signatures. Nat. Astron. 2, 459–464. doi: 10.1038/s41550-018-0450-z
Kattenhorn, S. A. (2018). Commentary: the feasibility of subduction and implications for plate tectonics on Jupiter's Moon Europa. J. Geophys. Res. Plan. 123:684–689. doi: 10.1002/2018JE005524
King, M. D., France, J. L., Fisher, F. N., and Beine, H. J. (2005). Measurement and modelling of UV radiation penetration and photolysis rates of nitrate and hydrogen peroxide in Antarctic sea ice: an estimate of the production rate of hydroxyl radicals in first-year sea ice. J. Photochem. Photobiol. A 176, 39–49. doi: 10.1016/j.jphotochem.2005.08.032
Klenner, F., Postberg, F., Hillier, J., Khawaja, N., Cable, M. L., Abel, B., et al. (2020). Discriminating abiotic and biotic fingerprints of amino acids and fatty acids in ice grains relevant to ocean worlds. Astrobiology. doi: 10.1089/ast.2019.2188. [Epub a head of print].
Levy, M., Miller, S. L., Brinton, K., and Bada, J. L. (2000). Prebiotic synthesis of adenine and amino acids under Europa-like conditions. Icarus 145, 609–123. doi: 10.1006/icar.2000.6365
Levy, M., Miller, S. L., and Oró, J. (1999). Production of guanine from NH4CN polymerizations. J. Mol. Evol. 49, 165–168. doi: 10.1007/PL00006539
Lui, R., and Orgel, L. (1998). Polymerization of β-amino acids in aqueous solution. Orig. Life Evol. Biosph. 28, 47–60. doi: 10.1023/A:1006580918298
Marín-Yaseli, M. R., Cid, C., Yagüe, A. I., and Ruiz-Bermejo, M. (2017). Detection of macromolecular fractions in HCN polymers using electrophoretic and ultrafiltration techniques. Chem. Biodiver. 14:e1600241. doi: 10.1002/cbdv.201600241
Marín-Yaseli, M. R., González-Toril, E., Mompeán, C., and Ruiz-Bermejo, M. (2016). The role of the aqueos aerosols in the “Glyoxylate Scenario”: an experimental approach. Chem. Eur. J. 22, 12785–12799. doi: 10.1002/chem.201602195
Marín-Yaseli, M. R., Mompéan, C., and Ruiz-Bermejo, M. (2015). A prebiotic synthesis of pterins. Chem. Eur. J. 21, 13531–13534. doi: 10.1002/chem.201502345
Marín-Yaseli, M. R., Moreno, M., de la Fuente, J. L., Briones, C., and Ruiz-Bermejo, M. (2018). Experimental conditions affecting the kinetics of aqueous HCN polymerization as revealed by UV–vis spectroscopy. Spectrochim. Acta Part A 191, 389–397. doi: 10.1016/j.saa.2017.10.003
McLaren, A. D., and Shugar, D. (1964). Photochemistry of Proteins and Nucleic Acids. New York, NY: Pargamon, 496–497.
Menor-Salvan, C., and Marín-Yaseli, M. (2012). Prebiotic chemistry in eutectic solutions at the water-ice matrix. Chem. Soc. Rev. 41, 5404–5415. doi: 10.1039/c2cs35060b
Menor-Salvan, C., and Marin-Yaseli, M. R. (2013). A new route for the prebiotic synthesis of nucleobases and hydantoins in water/ice solutions involving the photochemistry of acetylene. Chem. Eur. J. 19, 6488–6497. doi: 10.1002/chem.201204313
Menor-Salván, C., Ruiz-Bermejo, M., Guzmán, M. I., Osuna-Esteban, S., and Veintemillas-Verdaguer, S. (2009). Synthesis of pyrimidines and triazines in ice: implications for the prebiotic chemistry of nucleobases. Chem. Eur. J. 15, 4411–4418. doi: 10.1002/chem.200802656
Menor-Salván, C., Ruiz-Bermejo, M., Osuna-Esteban, S., Muñoz-Caro, G., and Veintemillas-Verdaguer, S. (2008). Synthesis of polycyclic aromatic hydrocarbons and acetylene polymers in ice: a prebiotic scenario. Chem. Biodiver. 5, 2729–2739. doi: 10.1002/cbdv.200890228
Miller, S. L., and Cleaves, H. J. (2006). “Prebiotic chemistry on the primitive earth,” in Systems Biology, Genomics, Vol. I, eds I. Rigoutsos and G. Stephanopouloss (Oxford: Oxford University Press), 4–56.
Miyakawa, S., Cleaves, H. J., and Miller, S. L. (2002). The cold origin of life: B. Implications based on pyrimidines and purines produced from frozen ammonium cyanide solutions. Orig. Life Evol. Biosph. 32, 209–218. doi: 10.1023/A:1019514022822
Mizutani, H., Mikuni, H., Takahasi, M., and Noda, H. (1975). Study on the photochemical reaction of HCN and its polymer products relating to primary chemical evolution. Orig. Life 6, 513–525. doi: 10.1007/BF00928899
Mohammed, F. S., Chen, K., Mojica, M., Conley, M., Napoline, J. W., Butch, C., et al. (2017). A plausible prebiotic origin of glyoxylate: nonenzymatic transamination reactions of glycine with formaldehyde. Synlett 28, 93–97. doi: 10.1055/s-0036-1588657
Mompeán, C., Marín-Yaseli, M. R., Espigares, P., González-Toril, E., and Ruiz-Bermejo, M. (2019). Prebiotic chemistry in neutral/reduced-alkaline gas-liquid interfaces. Sci. Rep. 9:1916. doi: 10.1038/s41598-018-36579-7
Monnard, P., Kanavarioti, A., and Deamer, D. W. (2003). Eutectic phase polymerization of activated ribonucleotide mixtures yields quasi-equimolar incorporation of purine and pyrimidine nucleobases. J. Am. Chem. Soc. 125, 13734–13740. doi: 10.1021/ja036465h
Morowitz, H. J., Kostelnik, J. D., Yang, J., and Cody, G. D. (2000). The origin of intermediary metabolism. Proc. Natl. Acad. Sci. U.S.A. 97:7704–7708. doi: 10.1073/pnas.110153997
Moser, R. E., Claggett, A. R., and Matthews, C. N. (1968a). Peptide formation from aminomalononitrile (HCN trimer). Tetrahedron. Lett. 9, 1605–1068. doi: 10.1016/S0040-4039(01)99012-4
Moser, R. E., Claggett, A. R., and Matthews, C. N. (1968b). Peptide formation from diaminomaleonitrile (HCN tetramer). Tetrahedron. Lett. 9, 1599–1603. doi: 10.1016/S0040-4039(01)99011-2
Nandi, S., Bhattacharyya, D., and Anoop, A. (2018). Prebiotic chemistry of HCN tetramerization by automated reaction search. Chem. Eur. J. 24, 4885–4894. doi: 10.1002/chem.201705492
Negrón-Mendoza, A., Dragani,ć, Z. D., Navarro-González, R., and Dragani,ć, I. G. (1983). Aldehydes, ketones, and carboxylic-acids formed radiolytically in aqueous-solutions of cyanides and simple nitriles. Radiat. Res. 95, 248–261. doi: 10.2307/3576253
Pappalardo, R. T., Vance, S., Bagenal, F., Bills, B. G., Blaney, D. L., Blankenship, D. D., et al. (2013). Science potential from a Europa Lander. Astrobiology 13, 740–773. doi: 10.1089/ast.2013.1003
Pasek, M. A. (2020). Plume sample modification at icy moons: implications for biosignatures. Front. Astron. Space Sci. 7:14. doi: 10.3389/fspas.2020.00014
Perovich, D. K. (2002). UV Radiation and Artic Ecosystems, ed D. O. Hessen (Berlin; Heidelberg: Springer), 73–89.
Postberg, F., Khawaja, N., Abel, B., Choblet, G., Glein, C. R., Gudipati, M. S., et al. (2018). Macromolecular organic compounds from the depths of Enceladus. Nature 558:564. doi: 10.1038/s41586-018-0246-4
Quick, L. C., and Hedman, M. M. (2020). Characterizing deposits emplaced by cryovolcanic plumes on Europa. Icarus 343:113667. doi: 10.1016/j.icarus.2020.113667
Rannou, P., and West, R. (2018). Supersaturation on Pluto and elsewhere. Icarus 312, 36–44. doi: 10.1016/j.icarus.2018.04.025
Ritson, D., and Sutherland, J. D. (2012). Prebiotic synthesis of simple sugars by photoredox systems chemistry. Nat. Chem. 4, 895–899. doi: 10.1038/nchem.1467
Ruiz-Bermejo, M., Osuna-Esteban, S., and Zorzano, M. P. (2013a). Role of ferrocyanides in the prebiotic synthesis of alpha-amino acids. Orig. Life Evol. Biosph. 43, 191–206. doi: 10.1007/s11084-013-9336-3
Ruiz-Bermejo, M., Zorzano, M.-P., and Osuna-Esteban, S. (2013b). Simple organics and biomonomers identified in HCN polymers: an overview. Life 3, 421–448. doi: 10.3390/life3030421
Russell, M. J., Murray, A. E., and Hand, K. P. (2017). The possible emergence of life and differentiation of a shallow biosphere on irradiated icy worlds: the example of Europa. Astrobiology 17, 1265–1273. doi: 10.1089/ast.2016.1600
Sánchez, R. A., Ferris, J. P., and Orgel, L. E. (1966). Conditions for purine synthesis: did prebiotic synthesis occur at low temperatures? Science 153, 72–73. doi: 10.1126/science.153.3731.72
Sánchez, R. A., Ferris, J. P., and Orgel, L. E. (1967). Studies in prebiotic synthesis II. synthesis of purine precursors and amino acids from aqueous hydrogen cyanide. J. Mol. Biol. 30, 223–252.
Schwartz, A. W., Joosten, H., and Voet, A. B. (1982). Prebiotic adenine synthesis via HCN oligomerization in ice. BioSystems 15, 191–193. doi: 10.1016/0303-2647(82)90003-X
Sephton, M. A., Waite, J. H., and Brockwell, T. G. (2018). How to detect life on icy moons. Astrobiology 18, 843–855. doi: 10.1089/ast.2017.1656
Sherwood, B., Lunine, J., Sotin, C., Cwik, T., and Naderi, F. (2018). Program options to explore ocean worlds. Acta Astron. 143, 285–296. doi: 10.1016/j.actaastro.2017.11.047
Sparks, W. B., Hand, K. P., McGrath, M. A., Bergeron, E., Cracraft, M., and Deustua, S. E. (2016). Probing for evidence of plumes on europa with HST/STIS. Astrophys J. 829:121. doi: 10.3847/0004-637X/829/2/121
Sparks, W. B., Schmidt, B. E., McGrath, M. A., Hand, K. P., Spencer, J. R., Cracraft, M., et al. (2017). Active cryovolcanism on Europa? Astrophys. J. Lett. 839:L18. doi: 10.3847/2041-8213/aa67f8
Sutherland, J. D. (2016). The origin of life—out of the blue. Angew. Chem. Int. Ed. 55, 104–121. doi: 10.1002/anie.201506585
Truong, N., Monroe, A. A., Glein, C. R., Anbar, A. D., and Lunine, J. I. (2019). Decomposition of amino acids in water with application to in-situ measurements of Enceladus, Europa and other hydrothermally active icy ocean worlds. Icarus 329, 140–147. doi: 10.1016/j.icarus.2019.04.009
Villafañe-Barajas, S. A., Colín-García, M., Negrón-Mendoza, A., and Ruiz-Bermejo, M. (2020). An experimental study of the thermolysis of hydrogen cyanide: the role of hydrothermal systems in chemical evolution. Int. J. Astrobiol. 2020, 1–10. doi: 10.1017/S1473550420000142
Waite, J. H., Glein, C. R., Perryman, R. S., Teolis, B. D., Magee, B. A., Miller, G., et al. (2017). Cassini finds molecular hydrogen in the Enceladus plume: evidence for hydrothermal processes. Science 356, 155–159. doi: 10.1126/science.aai8703
Keywords: HCN polymers, prebiotic chemistry, GC-MS, astrobiology, water interfaces, ocean worlds
Citation: Marín-Yaseli MR, González-Toril E and Ruiz-Bermejo M (2020) Reactivity of Cyanide at Water-Ice Interfaces: Implications for the Search for Organics on Icy Worlds. Front. Astron. Space Sci. 7:519017. doi: 10.3389/fspas.2020.519017
Received: 10 December 2019; Accepted: 12 August 2020;
Published: 18 September 2020.
Edited by:
Isik Kanik, NASA Jet Propulsion Laboratory (JPL), United StatesReviewed by:
Rafael Navarro-González, National Autonomous University of Mexico, MexicoSteve D. Vance, NASA Jet Propulsion Laboratory (JPL), United States
Copyright © 2020 Marín-Yaseli, González-Toril and Ruiz-Bermejo. This is an open-access article distributed under the terms of the Creative Commons Attribution License (CC BY). The use, distribution or reproduction in other forums is permitted, provided the original author(s) and the copyright owner(s) are credited and that the original publication in this journal is cited, in accordance with accepted academic practice. No use, distribution or reproduction is permitted which does not comply with these terms.
*Correspondence: Marta Ruiz-Bermejo, cnVpemJtJiN4MDAwNDA7Y2FiLmludGEtY3NpYy5lcw==