- 1Research Center of Biotechnology of the Russian Academy of Sciences, Winogradsky Institute of Microbiology, Moscow, Russia
- 2Network of Researchers on the Chemical Evolution of Life, Leeds, United Kingdom
- 3Yugra State University, Khanty-Mansiysk, Moscow, Russia
- 4Mendeleyev University of Chemical Technology, Moscow, Russia
A new nanobiotechnological approach for the detection of extraterrestrial Earth-like biological forms is proposed. The approach is based on the ability of microbial cells to reduce artificially added cations with the generation of crystalline nanoparticles (NPs) from zero-valent atoms. The method is named DBNG (Detection of Biogenic Nanoparticles Generation). The subglacial low-temperature oligotrophic Lake Untersee in Antarctica was used as a model of putative extraterrestrial water environments inhabited by Earth-like type microorganisms. The DBNG protocol for the comparative study of microbial communities of low-temperature oligotrophic environments was optimized on the base of experiments with the pure culture of psychroactive bacterium Cryobacterium sp. 1639 isolated earlier from Lake Untersee. The formation of silver nanoparticles (Ag°NPs) has been conducted in natural water samples of three horizons at low temperature (+5°C), which was in the temperature range registered in the Lake Untersee. The generation of biogenic Ag°NPs was detected only at the presence of indigenous microorganisms in all studied samples. No Ag°NPs generation was observed in the lake water samples artificially free of cells or exposed to pasteurization (two types of controls). The miniature microfluidic chip for an automated version of the device, based on using different analytical methods for recording in situ-formed biogenic nanoparticles, is proposed. The device allows the detection of the biological objects directly at the sampling site.
Introduction
Nowadays, the topical challenge of astrobiology is the development and testing of new methods for searching for Earth-like biological forms in Space. It is assumed that metabolically active microorganisms are present mainly in a state of aqueous suspensions in terrestrial environments. Many cells of such suspensions can remain viable for a very long time, especially in oligotrophic environments (Gordon et al., 2000; Vishnivetskaya et al., 2000; Ponder et al., 2008). Accordingly, searching for extraterrestrial biosignatures or biological Earth-like objects in most studies is focused on cosmic bodies containing water and ice (Andersen et al., 1995; Priscu et al., 1998; Storrie-Lombardi and Sattler, 2009; Haendel et al., 2011; Filippova et al., 2013; Westall et al., 2013; Koo et al., 2017, 2018). Various approaches to detect biosignatures have been suggested (Rivkina et al., 2000; Gilichinsky et al., 2007; Rogers et al., 2010; Andersen et al., 2011; Zhang et al., 2014; Bedrossian et al., 2017; Managadze et al., 2017). Nevertheless, the development of new effective techniques for the detection of putative life beyond Earth is still an important problem to be solved (Nadeau et al., 2008; Garcia-Descalzo et al., 2012; Judge, 2017; Nelis et al., 2018). The basic astrobiological methods are mostly related to search for biosignatures of a geological, chemical, and biomorphological nature (Cockell, 2010; Westall et al., 2015; Hays et al., 2017; Mickol et al., 2018). Here, we propose a novel nanobiotechnological approach for the detection of microbiological objects in water or melted ice samples from extraterrestrial low-temperature environments. The approach is named the Detection of the Biogenic Nanoparticles Generation (DBNG), and it based on the ability of microorganisms, in particular bacteria, to produce biogenic crystalline nanoparticles of reduced Ag° atoms of the artificially added silver salt solution.
It has already been shown that microorganisms are capable of reducing cations and generating nanoparticles from reduced zero-valent atoms of metals (Wang et al., 2012; Wu et al., 2013). This is one of the natural non-specific mechanisms that are probably involved in the detoxification of metals, including the protection from cation entry into the cell (Merroun, 2007; Gadd, 2010). All tested microorganisms had this ability to reduce cations with different valences (Velusamy et al., 2016; Patil and Kim, 2017; Sorokin et al., 2019; Singh et al., 2020). It was also demonstrated that a much greater variety of nanoparticle assemblies could be produced by cells along the “bottom-up” approach (Zhang et al., 2011; Woehl et al., 2014; Sánchez-López et al., 2020) (Figure 1). Observations clearly show that nanocrystals can nucleate from an aqueous solution via a three-step mechanism: spinodal decomposition, clusterization, and nanocrystallization (Luo et al., 2017; Tan et al., 2017; Xie et al., 2017; Liao et al., 2019; Mikhailov and Mikhailova, 2019; Wang et al., 2019). The amorphous clusters of zero-valent atoms (up to 1–2 nm) are direct precursors of the bigger crystalline nanoparticles (Malis et al., 2011; Wan et al., 2013; Loh et al., 2017; Singh et al., 2020). Several studies have reported natural polymers (such as chitosan, proteins and amino acids, sugars, starch, and tannic acid) as reducing agents for the synthesis of metallic nanoparticles (Prakash and Sharma, 2010; Kharissova et al., 2013; Velusamy et al., 2016; Masse et al., 2019; Navarro Gallón et al., 2019). Differences in generated biogenic nanoparticles depend on the physiology of microorganisms as well as on the medium composition and conditions at their formation (Narayanan and Sakthivel, 2010; Zhang et al., 2011; Wang et al., 2012; Sorokin et al., 2013; Wu et al., 2013; Tyupa et al., 2016; Muller, 2018; Siddiqi et al., 2018). However, only metabolically active cells can rapidly (in minutes) generate metal nanoparticles from metal ions of the added salt due to their ability to constantly “efflux” electrons and electron donors into the medium (Zhou et al., 2013; Skladnev et al., 2017a; Wang et al., 2019). Inactive cells or cells with destroyed and non-active biostructures have a much weaker ability to reduce cations and form only the amorphous nanoclusters (up to 1–2 nm) as precursors of nanoparticles (Woehl et al., 2014; Liao et al., 2019). In solution, the organic components of the cytoplasms of destroyed cells can only act as low concentrated and “slow” chemical reducing agents. The generation of nanoparticles, when a source of metal cations is added into a sample, can be considered as an indicator of the presence of biological objects.
To confirm the efficiency of the proposed DBNG method, we applied it to natural water samples taken from the Antarctic Lake Untersee—an analog terrestrial system for putative cold extraterrestrial ecosystems (Andersen et al., 1995, 2011; Filippova et al., 2013; Heinz et al., 2018). The lake is an ice-covered reservoir, oligotrophic, and isolated from the Earth's atmosphere for millions of years. In previous studies, microbial communities present in the Lake Untersee have been shown to survive in extreme conditions, such as near-zero temperatures and low content of organic compounds (Fomenkov et al., 2017; Pikuta et al., 2017). The water reservoir with likely similar characteristics, was found under a layer of Martian ice in the southern polar region (Jones et al., 2018; Orosei et al., 2018; Post et al., 2019). The goal of the present work was to demonstrate the efficiency of the proposed nanobiotechnological DBNG method for astrobiology applications. The aim of the investigation was to study the biogenic Ag°NPs formation in situ in water samples from different horizons of subglacial Antarctic Lake Untersee containing indigenous microorganisms under low-temperature conditions.
Materials and Methods
Sampling of Different Horizons of the Lake Untersee, East Antarctica
Three water samples were taken aseptically from the subglacial low-temperature Lake Untersee, in East Antarctica (71°20′ S, 13°45′ E) located in the interior of the Gruber Mountains of central Queen Maud Land. This extremely oligotrophic lake is situated 563 m above the sea level and is the largest by surface area (11.4 km2) lake in East Antarctica. Samples from the lake were taken using 1-liter bathometers at the station of the ice camp, as described in the NASA 2015 Annual Science Report1 The water samples were collected by the depth profile of the column at 20, 40, and 72 m (starting from the smallest depth). Some environmental parameters are shown in Table 1. Water temperature in the horizons was from +0.3 to +4.9°C. The samples were aseptically transferred to sterile laboratory flasks and transported to the laboratory in heat-insulating containers and subsequently stored at +5°C. For the current study, about 4 ml of those water samples were kindly provided by Acad. Gal'chenko. The experiments for the generation of nanoparticles were started in 4 months of sample collection. The aliquots for analysis were also taken aseptically.
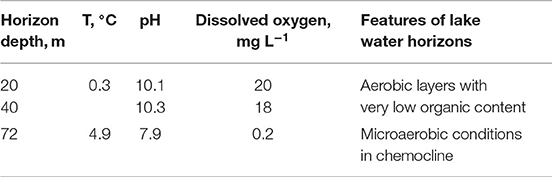
Table 1. Some characteristics of water sampling horizons from the Lake Untersee (Andersen et al., 2011).
The Pure Culture of Psychroactive Bacterium
The psychroactive bacterium Cryobacterium sp. 1639 was isolated in pure culture from the Lake Untersee water sample taken from a depth 72 m with registered in situ temperature of +4.9°C. The 16S rRNA sequence of the isolate was deposited in the NCBI GenBank database (MT364260).
Formation of Biogenic Silver Nanoparticles at DBNG Method
The principal scheme of the DBNG method is presented in Figure 2. The formation of biogenic Ag°NPs was carried out in 50-μL aliquots. The source of silver cations for generation of Ag°NPs was an aqueous solution of Ag(NH3)2NO3 synthesized from AgNO3 and ammonia according to a modified Tollens protocol (Anh-Tuan et al., 2010). Sterile 2 mM Ag(NH3)2NO3 solution was added directly to samples to achieve a final concentration of 0.1 mM. The generation of silver nanoparticles in native water samples was carried out at +5°C. The generation of silver nanoparticles by cells of strain Cryobacterium sp. 1639 was carried out at the exponential growth phase at two cultivation temperatures: +5°C (close to the Lake Untersee horizon temperature) and at +24°C (room temperature). The duration of exposure to silver salt in all experiments was 20 min (Sorokin et al., 2013). The experiments were conducted in triplicate. Sterility of Ag(NH3)2NO3 solution was proved by the absence of microbial growth in the LB medium. The absence of NPs in native water samples and Ag(NH3)2NO3 solution was confirmed by transmission electron microscopy. As cell-free control aliquots, 1.0 mL of the native water samples and the cultural fluid of Cryobacterium sp. 1639 free of microbial cells previously removed by centrifugation (10,000 g, 15 min) were used. In all cell-free controls formation of silver nanoparticles was carried out at two temperatures +5 and +24°C.
Electron Microscopy and X-Ray Microanalysis
Specimens were studied using a JEM-1400 microscope (JEOL, Japan) equipped with an X-ray microanalyzer (Oxford Instruments, United Kingdom) at an accelerating voltage of 80 keV. The samples were prepared using standard copper grids with Formvar film reinforced with carbon. Five microliters aliquots of each variant were applied to the copper grids and dried for 15 h.
A direct cell counting was conducted for the samples taken from different water horizons by the TEM examination of grids with native variants.
Analysis of the linear dimensions of Ag°NPs and their classification was carried out using the “Compass 3D-V14” software and a specially developed algorithm for calculating linear dimensions based on electron microscope images of at least 300 nanoparticles.
Inactivation of Cells of Cryobacterium sp. 1639 Culture and Water Samples From Horizons of the Lake Untersee
Four samples were pasteurized at 70°C for 30 min and used as negative controls (Cebrián et al., 2017).
Results
Formation of Silver Nanoparticles by Cells of Psychroactive Bacterium Cryobacterium sp. 1639
The psychroactive bacterium Cryobacterium sp. 1639 was isolated from horizon depth 72 m of the Lake Untersee. The culture Cryobacterium sp. 1639 grew well at +5°C and was able to reduce silver cations and forming Ag°NPs at +5°C during 20 min (Figure 3A). The size of 50% of these particles did not exceed 20 nm, while larger size appeared rare (Figure 3B). When the temperature was higher than 20°C, the growth of psychroactive bacterium Cryobacterium sp. 1639 was very slow, and the formation of Ag°NPs was not detected (Figure 3C). Cells of Cryobacterium sp. 1639 grown at +5°C lost their ability to reduce Ag+ cations fast and generate nanoparticles under non-optimal temperature conditions (at +24°C).
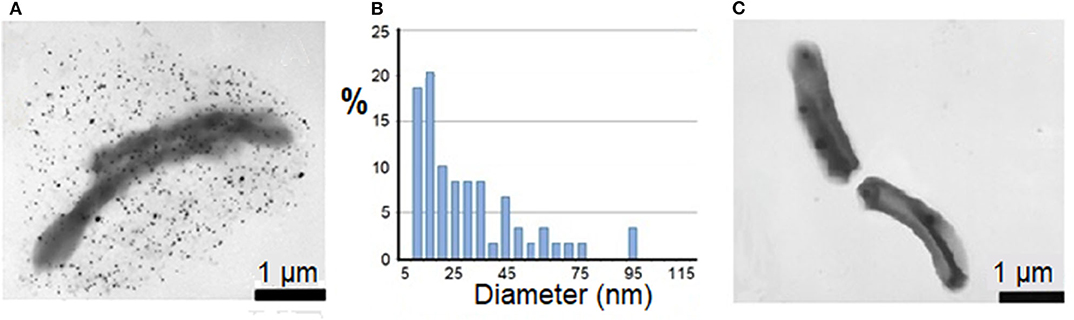
Figure 3. Distribution in sizes (B) of biogenic Ag°NPs generated by psychroactive Cryobacterium sp. 1639 at +5°C in 20 min (A). No Ag°NPs generated by cells Cryobacterium sp. 1639 at +24°C (C).
Formation of Silver Nanoparticles in Native Water Samples From the Lake Untersee
The direct cell counting in water horizons confirmed the presence of numerous indigenous microorganisms (from 3·104 to 2·106 mL-1). In all three studied water samples, the formation of biogenic Ag°NPs of 15–150 nm in diameter at +5°C in 20 min has been observed (Figure 4). In cell-free controls no Ag°NPs have been detected.
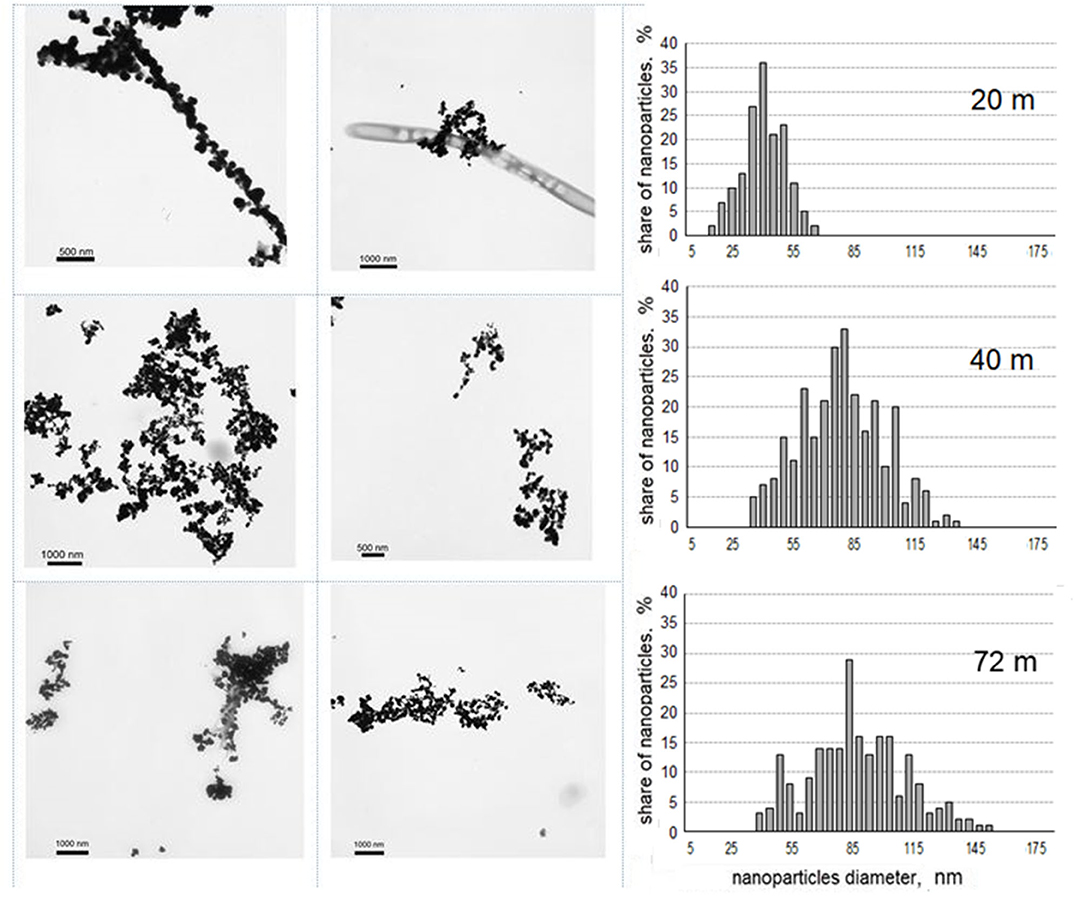
Figure 4. Biogenic Ag°NPs, formed at +5°C in 20 min in water samples of the Lake Untersee and their distribution in size (in percentage to the total amount).
The difference in the size distribution of Ag°NPs formed in water samples taken from three different horizons of the Lake Untersee was revealed. For these samples, distribution in size could be characterized by the normal law. Biogenic nanoparticles detected in the sample taken from 20 m depth had a maximum size of 40 nm. In the samples from depth 40 m and 72 m, the formed nanoparticles were twice as large. Nanoparticles from deeper samples had a larger size (35–120 nm for depth 40 m and 65–155 nm for depth 72 m), the predominant size was 80 nm.
Pools of biogenic nanoparticles formed in samples were different in both linear sizes and quantity. The same conditions for silver reduction in lake water samples made it possible to compare concentrations of biogenic Ag°NPs formed in these reactions. Amounts of biogenic Ag°NPs detected in water samples were normalized to those detected in the sample from the depth of 40 m (Figure 5).
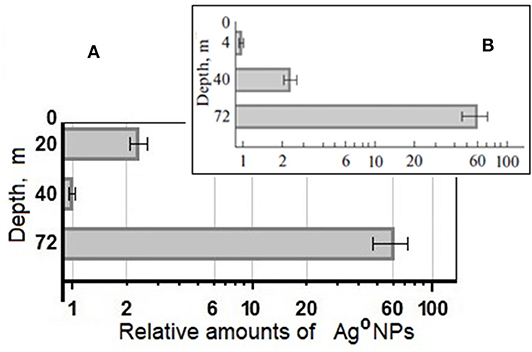
Figure 5. Relative number of Ag°NPs, formed at +5°C in water samples of the Lake Untersee from different depths, normalized to the amount of biogenic nanoparticles in the sample from the depth 40 m (A), (B) relative number of Ag°NPs, formed in the same water samples at +24°C (Skladnev et al., 2017a).
Formation of Nanoparticles by Inactivated Cells of Cryobacterium sp. 1639 and Native Microflora of Water Horizons of the Lake Untersee
The standard protocol of the DBNG method was used for experiments with non-specific inactivation of cell suspensions of psychoactive bacterium Cryobacterium sp. 1639 and three studied water samples with indigenous microorganisms of the Lake Untersee. No silver nanoparticles were observed in these four samples with their previous pasteurization.
Discussion
The Lake Untersee is highly oligotrophic, ice-covered, and has no anthropogenic impact. All these features make it a good terrestrial analog to putative cold extraterrestrial ecosystems and therefore an attractive subject for astrobiologists (Andersen et al., 1995, 2011; Cockell, 2010; Garcia-Lopez and Cid, 2017; Nelis et al., 2018; Post et al., 2019). All these characteristics make water samples from this lake acceptable for the approbation of the new method based on the ability of microbial forms to reduce Ag+ cations followed by the formation of biogenic Ag°NPs from zero-valent silver atoms.
Adaptation of the DBNG Method for the Detection of Microorganisms in Low-Temperature Water Environments
To adapt the DBNG method for the detection of microorganisms in low-temperature water environments (by in situ formation of the biogenic nanoparticles), we used the results of experiments with a pure culture of psychroactive bacteria isolated from the 72 m horizon of the Lake Untersee. It has been demonstrated that the psychroactive bacterium Cryobacterium sp. 1639 has good growth at +5°C (Bajerski et al., 2011), within the temperature range characteristic of its native habitat in the horizon of the Lake Untersee (while at +25°C bacterium Cryobacterium sp. 1639 grew three times slower). For the first time, it has been shown that psychroactive bacteria are capable of biogenic Ag°NPs formation at +5°C during 20 min. It is important to note that silver nanoparticles generated at these conditions were relatively small in size (20 nm or smaller in average) in comparison to those formed by indigenous microorganisms in water samples of the Lake Untersee. The possible explanation of the difference in size is the biosynthesis of low-molecular extracellular bioactive compounds of Cryobacterium sp. 1639. These compounds can be absorbed by the “young” nanoparticles (nanoclusters) and prevent their further growth (Zhang et al., 2011; Zhou et al., 2013). There were no biogenic Ag°NPs generated at +24°C for 20 min. Thus, the temperature +5°C is optimal for the DBNG method for detecting microorganisms in a low-temperature water system.
The formation in situ biogenic Ag°NPs in two horizons of the Lake Untersee at +24°C were described earlier (Skladnev et al., 2017a). It was shown that: (i) for the depth of 40 m—the size distribution of nanoparticles had the normal distribution with a distinct peak at 12–13 nm; (ii) for the depth of 72 m—half of Ag°NPs had a very small diameter (about 5 nm), whereas other nanoparticles were larger (from 12 to 50 nm).
In our study, at +5°C Ag°NPs showed different size distribution (Figure 4). In this case, Ag°NPs size distributions for horizons 40 and 72 m had the pick at 80 nm. We emphasize that large nanoparticles were formed over 20 min, indicating the presence of metabolically active microorganisms in these horizons.
The comparison of average amounts of formed Ag°NPs demonstrate that in the water sample from 72 m, the number of biogenic Ag°NPs is larger than in the water sample of the 40 m depth. It was true for both temperature conditions: at +5°C (60: 1), and +24°C (60: 2.5) (Figure 5). The value of relative amount Ag°NPs at +5°C is higher, which means that cells at low temperature demonstrate higher reducing activity. The high sensitivity of the DBNG method directly for native water samples was shown even at low-concentrated cell suspensions (20 m−3·104, 40 m−4.1·104 cells per mL).
In general, based on the data obtained in our experiments, we can conclude that the reduction efficiency for artificially added silver cations to samples is higher at the temperature close to the native environment (+5°C) than at the room temperature (+24°C), and this indicates to the true psychrophilic physiology of inhabitants. Biogenic Ag°NPs with a diameter larger than 10 nm can be detected by a wide range of analytical methods (visible and fluorescent spectrometry, spectroscopy of surface-enhanced Raman scattering, etc.).
Technical Principles of Analytical Methodology of the Proposed DBNG Method for Perspective Astrobiology Missions
For reliable detection of life-forms using the DBNG method, two controls have to be used: (i) an aliquot of the test sample mechanically purified from microbial cells (for example, by centrifugation) and (ii) an aliquot with inactivated cells (for example by pasteurization). The formation of nanoparticles (from artificially added cations) in the native sample would prove the presence of Earth-type active living forms, if there are no nanoparticles (from artificially added cations) in the two controls.
UV-visible and fluorescence spectrometry are the most frequently used tools for the detection of metal nanoparticles (Gomez et al., 2014; Loh et al., 2017; Liao et al., 2019; Sánchez-López et al., 2020). In earlier work (Sorokin et al., 2013), it was shown that biogenic Ag°NPs generated under DBNG protocol were confidently registered by visible spectrometry (Figure 6). Several models of miniature spectrometers with high sensitivity are available now. The protocol of the DBNG method permits the use of microliter volumes of examined samples and salt solutions. Devices for the production of nanoparticles by chemical methods based on microfluidic lab-on-a-chip (LOC) technologies have already been demonstrated (Wu et al., 2012; McLeod et al., 2013; Gomez et al., 2014; Buja et al., 2017). So, similar chips for detecting biogenic nanoparticles formed by microorganisms in aqueous samples can be developed (Skladnev et al., 2017b). Such devices can certainly be useful for searching for Earth-like living forms directly on extraterrestrial objects.
Conclusion
The fast in situ formation of the biogenic Ag°NPs in water samples of the Lake Untersee at low temperature was demonstrated. The efficiency of the DBNG method to detect living biological forms directly in native water samples (from 104 cells per mL) was confirmed. For evidence of the biogenic character of formed nanoparticles, we used two controls: water samples after the indigenous cells were artificially removed by centrifugation and water samples exposed to pasteurization. Biogenic nanoparticles formed due to application of the DBNG protocol can be detected by spectrometry and/or other physical methods. A compact chip, based on principles of the DBNG method, can be constructed. In summary, the proposed and tested in this work method can be used for the detection of active microbial Life in native samples and can be considered as a new nanobiotechnological tool for searching for Earthlike living forms elsewhere in Space.
Data Availability Statement
The raw data supporting the conclusions of this article will be made available by the authors, without undue reservation, to any qualified researcher.
Author Contributions
DS: general concept, nanoparticles forming experiments, literature review, and preparing the manuscript. LV and YB: isolation of microbial culture and incubation experiments. OK: general concept, correction of the manuscript, and organizational support. SK: literature review and correction of the manuscript. VS: general concept, electron microscopy investigations, and preparing the manuscript. All authors contributed to the article and approved the submitted version.
Conflict of Interest
The authors declare that the research was conducted in the absence of any commercial or financial relationships that could be construed as a potential conflict of interest.
Acknowledgments
The authors thank Acad. V. F. Gal'chenko, Dr. A. L. Mulyukin, and Jaime A. Cordova Jr. for help and support during our work. The work was carried out within the framework of a State Assignment of the Russian Ministry of Science and Higher Education. All microscopy studies were performed using the instruments in Core Facility Collection of Unique and Extremophilic Microorganisms (UNIQEM) in the Federal Research Center Fundamentals of Biotechnology of the Russian Academy of Sciences.
Footnotes
1. ^https://astrobiology.nasa.gov/nai/annual-reports/2015/seti/lake-sediment-habitats-lake-habitability-and-sediment-biosignatures/
References
Andersen, D. T., Doran, P., Bolshiyanov, D., Rice, J., Gal'chenko, V., Cherych, N., et al. (1995). A preliminary comparison of two perennially ice-covered lakes in Antarctica: analogs of past martian lacustrine environments. Adv. Space Res. 15, 199–202. doi: 10.1016/S0273-1177(99)80084-2
Andersen, D. T., Sumner, D. Y., Hawes, I., Webster-Brown, J. C., and Mckay, P. (2011). Discovery of large conical stromatolites in the Lake Untersee, Antarctica. Geobiology 9, 280–293. doi: 10.1111/j.1472-4669.2011.00279.x
Anh-Tuan, L., Huy, P. T., and Tam, P. D. (2010). Green synthesis of finely-dispersed highly bactericidal silver nanoparticles via modified tollens technique. Cur. Appl. Phys. 10, 910–916. doi: 10.1016/j.cap.2009.10.021
Bajerski, F., Ganzert, L., Mangelsdorf, K., Lipski, A., and Wagner, D. (2011). Cryobacterium arcticum sp. nov., a psychrotolerant bacterium from an Arctic soil. Int. J. System Evolut. Microbiol. 61, 1849–1853. doi: 10.1099/ijs.0.027128-0
Bedrossian, M., Lindensmith, C., and Nadeau, J. L. (2017). Digital holographic microscopy, a method for detection of microorganisms in plume samples from enceladus and other icy worlds. Astrobiology 17, 913–925. doi: 10.1089/ast.2016.1616
Buja, O.-M., Gordan, O. D., Leopold, N., Morschhauser, A., Nestler, J., and Zahn, D. R. T. (2017). Microfluidic setup for on-line SERS monitoring using laser induced nanoparticle spots as SERS active substrate. Beilstein J. Nanotechnol. 8, 237–243. doi: 10.3762/bjnano.8.26
Cebrián, G., Condón, S., and Mañas, P. (2017). Physiology of the inactivation of vegetative bacteria by thermal treatments: mode of action, influence of environmental factors and inactivation kinetics. Foods 6:107. doi: 10.3390/foods6120107
Cockell, C. S. (2010). Geomicrobiology beyond earth: microbe–mineral interactions in space exploration and settlement. Trends Microbiol. 18, 308–314. doi: 10.1016/j.tim.2010.03.005
Filippova, S. N., Surgucheva, N. A., Kulikov, E. E., Sorokin, V. V., Akimov, V. N., Bej, A. K., et al. (2013). Detection of phage infection in the bacterial population of the Lake Untersee (Antarctica). Microbiology 82, 383–386. doi: 10.1134/S0026261713030041
Fomenkov, A., Akimov, V. N., Vasilyeva, L. V., Andersen, D. T., Vincze, T., and Roberts, R. J. (2017). Complete genome and methylome analysis of psychrotrophic bacterial isolates from the Lake Untersee in Antarctica. Genome Announc. 5:e01753-16. doi: 10.1128/genomeA.01753-16
Gadd, G. M. (2010). Metals, minerals and microbes: geomicrobiology and bioremediation. Microbiology 156, 609–643. doi: 10.1099/mic.0.037143-0
Garcia-Descalzo, L., Garcia-Lopez, E., Moreno, A.M., Alcazar, A., Baquero, F., and Cid, C. (2012). Mass spectrometry for direct identification of biosignatures and microorganisms in earth analogs of mars. Planet. Space Sci. 72, 138–145. doi: 10.1016/j.pss.2012.08.009
Garcia-Lopez, E., and Cid, C. (2017). Glaciers and ice sheets as analog environments of potentially habitable icy worlds. Front. Microbiol. 8:1407. doi: 10.3389/fmicb.2017.01407
Gilichinsky, D. A., Wilson, G. S., Friedmann, E. I., Mckay, C. P., Sletten, R. S., Rivkina, E. M., et al. (2007). Microbial populations in Antarctic permafrost: biodiversity, state, age, and implication for astrobiology. Astrobiology 7, 275–311. doi: 10.1089/ast.2006.0012
Gomez, L., Sebastian, V., Irusta, S., Ibarra, A., Arruebo, M., and Santamaria, J. (2014). Scaled-up production of plasmonic nanoparticles using microfluidics: from metal precursors to functionalized and sterilized nanoparticles. Lab. Chip 14, 325–332. doi: 10.1039/C3LC50999K
Gordon, D. A., Priscu, J., and Giovannoni, S. (2000). Origin and phylogeny of microbes living in permanent antarctic Lake ice. Microbiol. Ecol. 39, 197–202.
Haendel, D., Hermichen, W. D., Höfling, R., and Kowski, P. (2011). Hydrology of the lakes in central wohlthat massif, east Antarctica: new results. Isot. Environ. Health Stud. 47, 402–406. doi: 10.1080/10256016.2011.630464
Hays, L. E., Graham, H. V., Des, M. D. J., Hausrath, E. M., Horgan, B., McCollom, T. M., et al. (2017). Biosignature preservation and detection in Mars analog environments. Astrobiology 17, 363–400. doi: 10.1089/ast.2016.1627
Heinz, J., Schirmack, J., Airo, A., Kounaves, S. P., and Schulze-Makuch, D. (2018). Enhanced microbial survivability in subzero brines. Astrobiology 18, 1171–1180. doi: 10.1089/ast.2017.1805
Jones, R. M., Goordial, J. M., and Orcutt, B. N. (2018). Low energy subsurface environments as extraterrestrial analogs. Front. Microbiol. 9:1605. doi: 10.3389/fmicb.2018.01605
Judge, P. A. (2017). Novel strategy to seek biosignatures at enceladus and Europa. Astrobiology 17, 852–861. doi: 10.1089/ast.2017.1667
Kharissova, O. V., Dias, H. V. R., Kharisov, B. I., Pérez, B. O., and Pérez, V. M. J. (2013). The greener synthesis of nanoparticles. Trends Biotechnol. 31, 240–248. doi: 10.1016/j.tibtech.2013.01.003
Koo, H., Hakim, J., Morrow, C., Crowley, M., Andersen, D., and Bej, A. (2018). Metagenomic analysis of microbial community compositions and cold-responsive stress genes in selected Antarctic lacustrine and soil ecosystems. Life 8:29. doi: 10.3390/life8030029
Koo, H., Mojib, N., Hakim, J. A., Hawes, I., Tanabe, Y., Andersen, D. T., et al. (2017). Microbial communities and their predicted metabolic functions in growth laminae of a unique large conical mat from the Lake Untersee, East Antarctica. Front. Microbiol. 8:1347. doi: 10.3389/fmicb.2017.01347
Liao, C., Li, Y., and Tjong, S. C. (2019). Bactericidal and cytotoxic properties of silver nanoparticles. Int. J. Mol. Sci. 20:449. doi: 10.3390/ijms20020449
Loh, N. D., Sen, S., Bosman, M., Tan, S. F., Zhong, J., Nijhuis, C. A., et al. (2017). Multistep nucleation of nanocrystals in aqueous solution. Nat. Chem. 9, 77–82. doi: 10.1038/nchem.2618
Luo, B., Smith, J. W., Ou, Z., and Chen, Q. (2017). Quantifying the self-assembly behavior of anisotropic nanoparticles using liquid-phase transmission electron microscopy. Acc. Chem. Res. 50, 1125–1133. doi: 10.1021/acs.accounts.7b00048
Malis, O., Byard, C., Mott, D., Wanjala, B. N., Loukrakpam, R., Luo, J., et al. (2011). Low-temperature phase and morphology transformations in noble metal nanocatalysts. Nanotechnol 22, 1–8. doi: 10.1088/0957-4484/22/2/025701
Managadze, G. G., Safronova, A. A., Luchnikov, K. A., Vorobyova, E. A., Duxbury, N. S., Wurz, P., et al. (2017). A new method and mass-spectrometric instrument for extraterrestrial microbial life detection using the elemental composition analyses of martian regolith and permafrost ice. Astrobiology 17, 448–458. doi: 10.1089/ast.2016.1511
Masse, F., Desjardins, P., Ouellette, M., Couture, C., Omar, M. M., Pernet, V., et al. (2019). Synthesis of ultrastable gold nanoparticles as a new drug delivery system. Molecules 24:2929. doi: 10.3390/molecules24162929
McLeod, E., Luo, W., Mudanyali, O., Greenbaum, A., and Ozcan, A. (2013). Toward giga-pixel nanoscopy on a chip: a computational wide-field look at the nano-scale without the use of lenses. Lab Chip. 13, 2028–2035. doi: 10.1039/c3lc50222h
Merroun, M. L. (2007). “Interactions between metals and bacteria: fundamental and applied research,” in Communicating Current Research and Educational Topics and Trends in Applied Microbiology, ed A. Méndez-Vilas (Formatex), 108–119. Available online at: https://www.researchgate.net/publication/271196184
Mickol, R. L., Laird, S. K., and Kral, T. A. (2018). Non-psychrophilic methanogens capable of growth following long-term extreme temperature changes with application to mars. Microorganisms 6:34. doi: 10.3390/microorganisms6020034
Mikhailov, O. V., and Mikhailova, E. O. (2019). Elemental silver nanoparticles: biosynthesis and bio applications. Materials 12:3177. doi: 10.3390/ma12193177
Muller, M. (2018). Bacterial silver resistance gained by cooperative interspecies redox behavior. Antimicrob. Agents Chemother. 62:e00672-18. doi: 10.1128/AAC.00672-18
Nadeau, J. L., Perreault, N. N., Niederberger, T. D., Whyte, L. G., Sun, H. J., and Leon, R. (2008). Fluorescence microscopy as a tool for in situ life detection. Astrobiology 8, 859–874. doi: 10.1089/ast.2007.0043
Narayanan, K. B., and Sakthivel, N. (2010). Biological synthesis of metal nanoparticles by microbes. Adv. Colloid Interface Sci. 156, 1–13. doi: 10.1016/j.cis.2010.02.001
Navarro Gallón, S. M., Alpaslan, E., Wang, M., Larese-Casanova, P., Londoño, M. E., Atehortúa, L., et al. (2019). Characterization and study of the antibacterial mechanisms of silver nanoparticles prepared with microalgal exopolysaccharides. Mater. Sci. Eng. C Mater. Biol. Appl. 99, 685–695. doi: 10.1016/j.msec.2019.01.134
Nelis, J., Elliott, C., and Campbell, K. (2018). “The smartphone's guide to the galaxy”: in situ analysis in space. Biosensors 8:96. doi: 10.3390/bios8040096
Orosei, R., Lauro, S. E., Pettinelli, E., Cicchetti, A., Coradini, M., Cosciotti, B., et al. (2018). Radar evidence of subglacial liquid water on mars. Science 361, 490–493. doi: 10.1126/science.aar7268
Patil, M. P., and Kim, G. D. (2017). Eco-friendly approach for nanoparticles synthesis and mechanism behind antibacterial activity of silver and anticancer activity of gold nanoparticles. Appl. Microbiol. Biotechnol. 101, 79–92. doi: 10.1007/s00253-016-8012-8
Pikuta, E. V., Lyu, Z., Hoover, R. B., Liu, Y., Patel, N. B., Busse, H. J., et al. (2017). Williamwhitmania taraxaci gen. nov., sp. nov., a proteolytic anaerobe with a novel type of cytology from The Lake Untersee in Antarctica, description of Williamwhitmaniaceae fam. nov., and emendation of the order bacteroidales krieg. Int. J. Syst. Evol. Microbiol. 67, 4132–4145. doi: 10.1099/ijsem.0.002266
Ponder, M. A., Thomashow, M. F., and Tiedje, J. M. (2008). Metabolic activity of siberian permafrost isolates, Psychrobacter arcticus and Exiguobacterium sibiricum, at low water activities. Extremophiles 12, 481–490. doi: 10.1007/s00792-008-0151-0
Post, E., Alley, R. B., Christensen, T. R., Macias-Fauria, M., Forbes, B. C., Gooseff, M. N., et al. (2019). The polar regions in a 2°C warmer world. Sci. Adv. 5:eaaw9883. doi: 10.1126/sciadv.aaw9883
Prakash, A., and Sharma, S. (2010). Bacteria mediated extracellular synthesis of metallic nanoparticles. Int. Res. J. Biotechnol. 1, 71–79.
Priscu, J. C., Fritsen, C. H., Adams, E. E., Giovannoni, S. J., Paerl, H. W., McKay, C. P., et al. (1998). Perennial Antarctic lake ice: an oasis for life in a polar desert. Science 280, 2095–2098. doi: 10.1126/science.280.5372.2095
Rivkina, E. M., Friedmann, E. I., Mckay, C. P., and Gilichinsky, D. A. (2000). Metabolic activity of permafrost bacteria below the freezing point. Appl. Environ. Microbiol. 66, 230–3233. doi: 10.1128/AEM.66.8.3230-3233.2000
Rogers, J. D., Perreault, N. N., Niederberger, T. D., Lichten, C., Whyte, L. G., and Nadeau, J. L. (2010). A life detection problem in a high arctic microbial community. Planet. Space Sci. 58, 623–630. doi: 10.1016/j.pss.2009.06.014
Sánchez-López, E., Gomes, D., Esteruelas, G., Bonilla, L., Lopez-Machado, A. L., Galindo, R., et al. (2020). Metal-based nanoparticles as antimicrobial agents: an overview. Nanomaterials 10:292. doi: 10.3390/nano10020292
Siddiqi, K. S., Husen, A., and Rao, R. A. K. (2018). A review on biosynthesis of silver nanoparticles and their biocidal properties. J. Nanobiotechnol. 16:14. doi: 10.1186/s12951-018-0334-5
Singh, A., Gautam, P. K., Gautam, A., Gautam, V., Shivapriya, P. M., Shivalka, R. S., et al. (2020). Green synthesis of metallic nanoparticles as effective alternatives to treat antibiotics resistant bacterial infections: a review. Biotechnol. Rep. 25:e00427. doi: 10.1016/j.btre.2020.e00427
Skladnev, D. A., Kotsyurbenko, O. R., and Sorokin, V. V. (2017b). “Nanobiotechnological approach for detection of life in extraterrestrial water and ice environment,” in The 10th International Conference European Astrobiology Network Association (EANA2017) (Aarhus), 103.
Skladnev, D. A., Sorokin, V. V., and Gal'chenko, V. F. (2017a). Formation of silver nanoparticles in water samples from Antarctic the Lake Untersee. Microbiology 86, 355–362. doi: 10.1134/S002626171703016X
Sorokin, V. V., Pshenichnikova, A. B., Kalenov, S. V., Suyasov, N. A., and Skladnev, D. A. (2019). Comparison of the wild-type obligate methylotrophic bacterium Methylophilus quaylei and its isogenic streptomycin-resistant mutant via metal nanoparticle generation. Biol. Trace Elem. Res. 193, 564–573. doi: 10.1007/s12011-019-01740-4
Sorokin, V. V., Skladnev, D. A., Volkov, V. V., Tereshchenko, E. Y., Mulyukin, A. L., and Gal'chenko, V. F. (2013). The pathways of silver nanoparticles formation by Mycobacterium smegmatis. Dokl. Biol. Sci. 452, 325–328. doi: 10.1134/S0012496613050153
Storrie-Lombardi, M. C., and Sattler, B. (2009). Laser-induced fluorescence emission (LIFE): in situ nondestructive detection of microbial life in the ice covers of Antarctic lakes. Astrobiology 9, 659–672. doi: 10.1089/ast.2009.0351
Tan, S. F., Chee, S. W., Lin, G., and Mirsaidov, U. (2017). Direct observation of interactions between nanoparticles and nanoparticle self-assembly in solution. Acc. Chem. Res. 50, 1303–1312. doi: 10.1021/acs.accounts.7b00063
Tyupa, D. V., Kalenov, S. V., Baurina, M. M., Yakubovich, L. M., Morozov, A. N., Zakalyukin, R. M., et al. (2016). Efficient continuous biosynthesis of silver nanoparticles by activated sludge micromycetes with enhanced tolerance to metal ion toxicity. Enzyme Microb. Technol. 95, 137–145. doi: 10.1016/j.enzmictec.2016.10.008
Velusamy, P., Kumar, G. V., Jeyanthi, V., Das, J., and Pachaiappan, R. (2016). Bio-Inspired green nanoparticles: synthesis, mechanism, and antibacterial application. Toxicol. Res. 32, 95–102. doi: 10.5487/TR.2016.32.2.095
Vishnivetskaya, T., Kathariou, S., McGrath, J., Gilichinsky, D., and Tiedje, J. M. (2000). Low-temperature recovery strategies for the isolation of bacteria from ancient permafrost sediments. Extremophiles 4, 165–173. doi: 10.1007/s007920070031
Wan, Y., Guo, Z., Jiang, X., Fang, K., Lu, X., Zhang, Y., et al. (2013). Quasi-spherical silver nanoparticles: aqueous synthesis and size control by the seed-mediated lee–meisel method. J. Coll. Interface Sci. 394, 263–268. doi: 10.1016/j.jcis.2012.12.037
Wang, J., Lin, X., Shu, T., Su, L., Liang, F., and Zhang, X. (2019). Self-assembly of metal nanoclusters for aggregation-induced emission. Int. J. Mol. Sci. 20:1891. doi: 10.3390/ijms20081891
Wang, L., Xu, L., Kuang, H., Xu, C., and Kotov, N. A. (2012). Dynamic nanoparticles assemblies. Acc. Chem. Res. 45, 1916–26. doi: 10.1021/ar200305f
Westall, F., Foucher, F., Bost, N., Bertrand, M., Loizeau, D., Vago, J., et al. (2015). Biosignatures on mars: what, where, and how? implications for the search for martian life. Astrobiology 15, 998–1029. doi: 10.1089/ast.2015.1374
Westall, F., Loizeau, D., Foucher, F., Bost, N., Betrand, M., Vago, J., et al. (2013). Habitability on Mars from a microbial point of view. Astrobiology 3, 887–897. doi: 10.1089/ast.2013.1000
Woehl, T. J., Park, C., Evans, J. E., Arslan, I., Ristenpart, W. D., and Browning, N. D. (2014). Direct observation of aggregative nanoparticle growth: kinetic modeling of the size distribution and growth rate. Nano Lett. 14, 373–378. doi: 10.1021/nl4043328
Wu, H. F., Gopal, J., and Manikandan, M. (2012). Future perspective of nanoparticle interaction-assisted laser desorption/ionization mass spectrometry for rapid, simple, direct and sensitive detection of microorganisms. J. Mass. Spectrom. 47, 355–63. doi: 10.1002/jms.2962
Wu, R., Cui, L., Chen, L., Wang, C., Cao, C., Sheng, G., et al. (2013). Effects of bio-Au nanoparticles on electrochemical activity of Shewanella oneidensis wild type and DomCA/mtrC mutant. Sci. Rep. 3:3307. doi: 10.1038/srep03307
Xie, Y., Dong, H., Zeng, G., Tang, L., Jiang, Z., Zhang, C., et al. (2017). The interactions between nanoscale zero-valent iron and microbes in the subsurface environment. J. Hazard. Mater. 321, 390–407. doi: 10.1016/j.jhazmat.2016.09.028
Zhang, X., Wang, W., Chen, W., Zhang, N., and Zeng, H. (2014). Comparison of seasonal soil microbial process in snow-covered temperate ecosystems of Northern China. PLoS ONE 9:e92985. doi: 10.1371/journal.pone.0092985
Zhang, X., Yan, S., Tyagi, R. D., and Surampalli, R. Y. (2011). Synthesis of nanoparticles by microorganisms and their application in enhancing microbiological reaction rates. Chemosphere 82, 489–494. doi: 10.1016/j.chemosphere.2010.10.023
Keywords: astrobiology, extraterrestrial life, nanoparticles, psychrophiles, DBNG method
Citation: Skladnev DA, Vasilyeva LV, Berestovskaya YY, Kotsyurbenko OR, Kalenov SV and Sorokin VV (2020) Detection of Microorganisms in Low-Temperature Water Environments by in situ Generation of Biogenic Nanoparticles. Front. Astron. Space Sci. 7:59. doi: 10.3389/fspas.2020.00059
Received: 01 January 2020; Accepted: 31 July 2020;
Published: 23 November 2020.
Edited by:
Tetyana Milojevic, University of Vienna, AustriaReviewed by:
Pauli Erik Laine, University of Jyväskylä, FinlandLena V. Pikuta, Athens State University, United States
Tatiana A. Vishnivetskaya, The University of Tennessee, Knoxville, United States
Iren Kuznetsova, Kotelnikov Institute of Radio Engineering and Electronics of RAS, Russia
Copyright © 2020 Skladnev, Vasilyeva, Berestovskaya, Kotsyurbenko, Kalenov and Sorokin. This is an open-access article distributed under the terms of the Creative Commons Attribution License (CC BY). The use, distribution or reproduction in other forums is permitted, provided the original author(s) and the copyright owner(s) are credited and that the original publication in this journal is cited, in accordance with accepted academic practice. No use, distribution or reproduction is permitted which does not comply with these terms.
*Correspondence: Oleg R. Kotsyurbenko, a290c29yQGhvdG1haWwuY29t