- 1Space Biochemistry Group, Department of Biophysical Chemistry, University of Vienna, Vienna, Austria
- 2Institute of Electron Microscopy and Nanoanalysis, Graz Centre for Electron Microscopy, Graz, Austria
- 3Voestalpine BÖHLER Edelstahl GmbH & Co KG, Kapfenberg, Austria
Prokaryotes are among the most versatile organisms on Earth and their ability to adsorb metals for nutrient, energy, or protection purposes can be noted in many different environments on our planet. The extreme thermoacidophilic archaeon Metallosphaera sedula is a metal-mobilizing archaeon capable of redox transformations during chemolithoautotrophic growth on diverse metal-bearing compounds. Examining the interfaces of this extreme metallophilic archaeon with various metal-bearing substrates of terrestrial and extraterrestrial origin, we have detected its selective preservation after desiccation. Cultivated on specific metal-bearing materials, e.g., tungsten-bearing scheelite, tungsten-bearing polyoxometalate, multimetallic waste products, and the NWA 1172 meteorite, cells of M. sedula can be preserved after dehydration, and therefore can potentially serve as a microbial fingerprint of the presence and/or activity of metal-transforming microorganisms. Preservation of desiccated M. sedula cells reported in this study has a discriminatory character, depending on the content and nature of the metal-containing compound used for cultivation of this metallophilic microorganism. The achieved preservation of dehydrated M. sedula cells facilitates our survivability studies with this desiccated microorganism during future space exposure experiments and under simulated space environmental conditions.
Introduction
Liquid water is a vital requirement for life on our planet and is the main research focus for scientists looking for life beyond Earth. Nonetheless, various organisms, including unicellular and multicellular life forms, can be preserved in dehydrated settings for long periods. However, dehydration remains a severe stress that leads to cellular perturbation interfering with survival. It has been widely accepted that intracellular compatible solutes impact microbial tolerance to desiccation. A potential positive influence of compatible solutes, e.g., trehalose or sucrose, on cell membranes during dehydration has been shown by Hincha and Hagemann (2004). The efficient ability of trehalose to preserve the function of dried biological macromolecules is widely used for protecting cell membranes and proteins from extreme temperatures, desiccation, and osmotic shock in a wide range of microorganisms. Trehalose biosynthesis genes were described in the genomes of thermophilic members of the Sulfolobaceae family, including Metallosphaera spp. (Seo et al., 2008; Okazaki et al., 2012; Moon et al., 2016). In addition to metabolic defense with non-reducing sugars, key molecular elements for the repair of dehydration effects (e.g., oxidative stress and DNA damage) might also be engaged by microorganisms. Apart from intracellular molecular protectants, production of stress-induced biofilms can have a substantial influence on the dehydration tolerance of thermophilic archaea and bacteria, physically preventing water loss in the cells (La Paglia and Hartzell, 1997; Mortel and Halverson, 2004; Chang et al., 2007; Beblo et al., 2011). Another strategy, an inorganic protection by sulfidic ore particles, has been described for the extreme thermophile Hydrogenothermus marinus exposed to a combination of desiccation and irradiation (Beblo et al., 2011), asserting a “shielding by ore” effect.
A multitude of multifunctional biogenic structures emerged repeatedly and independently over the course of Earth's history. Armor as a protective mechanism against harsh environmental conditions has been particularly successful. Multicellular organisms, e.g., chitons, have taken advantage of metal-encrusted armor for more than 200 million years (Li et al., 2015). Metal-bearing cell walls of single-celled microorganisms can serve as a protective armor against phytoplankton predators. Microbial survival in extreme environments with high metal concentrations is usually connected with cell surface absorption and/or extracellular polymeric substance (EPS) complexation of various surrounding metals (Schultze-Lam et al., 1996; Loaëc et al., 1998; Gupta and Diwan, 2017; Hickman-Lewis et al., 2019). Moreover, for endolithic and metal-respiring microorganisms, metal accumulation on the cell surface can serve as a mechanism for nutrient and energy source. Certain cell wall structures serve as a nucleation site and might enhance the metal accumulation process by providing a surface that promotes adsorption or precipitation of metals. Prokaryotes (e.g., cyanobacteria, proteobacteria, archaea) are able to adsorb metals, and even radionuclides on their surfaces (proteinaceous S-layer, sheaths, capsules, cell walls), which can serve as an extracellular nucleation site (van Gemerden, 1986; Beveridge, 1989; Ehrlich, 1999). The potential to withstand and promote metal accumulation followed by subsequent cell preservation has been examined using different microbial strains (archaea and bacteria); however, only limited reports exist revealing archaeal–metal interactions. To gain deeper insight into what drives microbial metal accumulation, precipitation, and subsequent cellular preservation, microbes from the Bacteria and Archaea kingdoms have been artificially encrusted (impregnated) with metals since the 1970s. Cyanobacteria have been successfully silicified, which leads to microbial structures that are well-preserved and thoroughly embedded in a crystalline silica matrix (Oehler and Schopf, 1971). The first experimental silicification of archaeal strains was performed with two strictly anaerobic and hyperthermophilic microorganisms, Methanocaldococcus jannaschii and Pyrococcus abyssi that could have inhabited hydrothermal environments on the early Earth and probably the early Mars as well. The obtained observations demonstrated that not all (micro)organisms are susceptible to metal precipitation and preservation; they behave differently even though they are closely related phylogenetically. Although both strains possess similar cell wall structures, most M. janaschii cells could not withstand silicification and lysed quickly, whereas silica precipitated on the cell wall of P. abyssi and its cells were well-preserved (Orange et al., 2009). Experimental silicification of the Gram-positive bacterial species Geobacillus SP7A over a period of 5 years led the authors to the conclusion that this species impregnates with silica faster than other thermophilic or mesophilic Gram-negative bacteria and archaea, due to a Gram-positive characteristic thick peptidoglycan layer, containing abundant anionic functional groups as primary silica binding sites (carbonyl, hydroxyl, phosphoryl groups). Furthermore, fast and efficient metal accumulation processes seem to be crucial to prevent degradation of organic material and to preserve the structural integrity of cellular material throughout a long period of time (Li et al., 2014; Orange et al., 2014). Rapid and high-precision preservation of microorganisms in the geological record is of utmost importance for the successful fossilization process (Orange et al., 2014). Experimental fossilization as a tool to study the preservation potential of different Bacterial and Archaeal communities could improve our understanding of the nature of Earth's earliest fossils. Importantly, the experimental fossilization approach enables thorough investigation of the influence of geological/mineralogical settings on microbial preservation potential. To extend the search of preserved biomarkers from Earth to Mars, the polyextremotolerant bacterium Yersinia intermedia was artificially encrusted by silica and gypsum under cold and anoxic settings, similar to current Martian conditions. Yersinia cells interacted immediately with the metal-bearing materials, which favors the preservation of cells during aging due to early entombment in a metal-bearing matrix (Gaboyer et al., 2017). The capacity of biosorption depending on a specific cell wall structure has been examined in two halophilic archaeal strains of Halobacterium noricense, which sequesters uranium in cell agglomerates and structures (Bader et al., 2017).
The extreme thermoacidophilic archaeon Metallosphaera sedula is a metal-mobilizing organism capable of redox transformations of a variety of metal-bearing substrates (Huber et al., 1989; Peeples and Kelly, 1995; Auernik and Kelly, 2008, 2010a; Maezato et al., 2012; Mukherjee et al., 2012; Wheaton et al., 2016; Kölbl et al., 2017; Blazevic et al., 2019; Milojevic et al., 2019a,b). Our recent studies indicate that this metallophilic archaeon can form a metal-bearing crust encasing its S-layer (Blazevic et al., 2019; Milojevic et al., 2019a,b). Examining the physiology of this extreme metallophilic archaeon cultivated with diverse metal-bearing substrates of terrestrial (Blazevic et al., 2019; Milojevic et al., 2019a) and extraterrestrial origin (Kölbl et al., 2017; Milojevic et al., 2019b), we have observed its selective preservation under the conditions of desiccation. Here, we report on several cases of the metal-grown polyextremophilic archaeon M. sedula, which were particularly beneficial to preserve its viability and cellular integrity after long-term desiccation.
Materials and Methods
Strain and Media Composition
Metallosphaera sedula (DSMZ 5348) cultures were grown aerobically as described before (Kölbl et al., 2017) in DSMZ88 Sulfolobus medium containing 0.28 g KH2PO4, 1.3 g (NH4)2SO4, 0.07 g CaCl2·2 H2O, 0.25 g MgSO4·7 H2O, and 0.02 g FeCl3·6 H2O dissolved in 1 L of water. After autoclaving, Allen's trace elements solution was added to 1 L of media resulting in 4.50 mg Na2B4O7·10 H2O, 1.80 mg MnCl2·4 H2O, 0.05 mg CuCl2·2 H2O, 0.22 mg ZnSO4·7 H2O, 0.03 mg VSO4·2 H2O, 0.03 mg Na2MoO4·2 H2O, and 0.01 mg CoSO4 (final concentration). The pH was adjusted with 10 N H2SO4 to 2.0.
Cultivation Setup
Chemolithoautotrophic cultivation of M. sedula was performed in DSMZ88 Sulfolobus medium defined above as described earlier (Milojevic et al., 2019a,b). Chemolithoautotrophic cultures were supplemented with 10 g/L of (i) a tungsten-bearing scheelite ore containing impurities of Mn and Fe oxides (Blazevic et al., 2019; see Supplementary Table 1 for chemical composition); (ii) the Dawson-type tungsten-bearing polyoxometalate W-POM (K6[α-P2W18O62]·nH2O) (Contant et al., 2007; Milojevic et al., 2019a); (iii) the stony meteorite NWA 1172 (H5 ordinary chondrite Northwest Africa 1172) (Russell et al., 2002; Milojevic et al., 2019b; see Supplementary Tables 1, 2 for chemical and mineralogical composition); (iv) multimetallic waste products (voestalpine BÖHLER Edelstahl GmbH & Co KG); and (v) multimetallic Martian regolith simulants (MRSs; Kölbl et al., 2017; see Supplementary Tables 1, 2 for chemical and mineralogical composition). The metal-containing compounds were temperature sterilized in a heating chamber (180°C) for a minimum of 24 h prior to autoclaving (121°C for 20 min). Abiotic controls containing uninoculated culture media with all aforementioned metal-bearing sources were included through all the experiments. Growth of cells was examined by phase contrast/epifluorescence microscopy and metal release (Kölbl et al., 2017; Milojevic et al., 2019a,b).
Dehydration Experiments
Dehydration of M. sedula cultures grown on various metal-bearing materials was performed under oxic laboratory conditions and under atmospheric pressure. For the dehydration experiments, cultures of M. sedula autotrophically cultivated on metal-bearing materials were harvested at middle stationary phase (Supplementary Table 3) omitting centrifugation and concentration, deposited by spreading evenly on glass plates (VWR International, Ø7 cm), and desiccated at room temperature within 60 days. Abiotic controls containing uninoculated culture media with the corresponding metal-bearing sources were included throughout the experiments. The morphology of the desiccated cells of M. sedula and crystalline and amorphous precipitates were examined by means of scanning electron microscopy (SEM). The contents of glass plates with desiccated cells were transferred into the culture media supplemented with 1% tryptone extract and incubated heterotrophically in a shaking orbital bath at 73°C. Growth of the cells was monitored during 1-week post-inoculation by phase-contrast/epifluorescence microscopy with 60× and 100× magnification (Supplementary Figure 1).
Scanning Electron Microscopy
The precipitates obtained after dehydration experiments were examined with a Zeiss Supra 55 VP scanning electron microscope, operated with a field emission gun (Schottky-FE, DENKA). Prior to SEM, the dehydrated samples were coated with a 3 nm Au/Pd layer (spincoater Laurell WS-650-23).
Statistical Analysis
The Excel 2016 (version 7.0) and Sigma plot (version 13.0) software packages were used to perform statistical analysis and graphical representation of the obtained data.
Results
Dehydration of M. sedula Cells
The cultures of M. sedula chemolithoautotrophically grown on various metal-bearing materials (Supplementary Table 2, Kölbl et al., 2017; Blazevic et al., 2019; Milojevic et al., 2019a,b) were dehydrated for 60 days at room temperature under atmospheric laboratory conditions. The surface of the precipitates obtained after dehydration was examined using SEM. The cell cultures were deposited and dehydrated in their respective glassware as monolayers composed of single cells (Figures 1–3), thus avoiding protective “shielding” effect of cellular multilayers during dehydration. In the case of M. sedula grown on MRSs, no preserved cells were detected after the 60-day period of dehydration (Figures 3G–J).
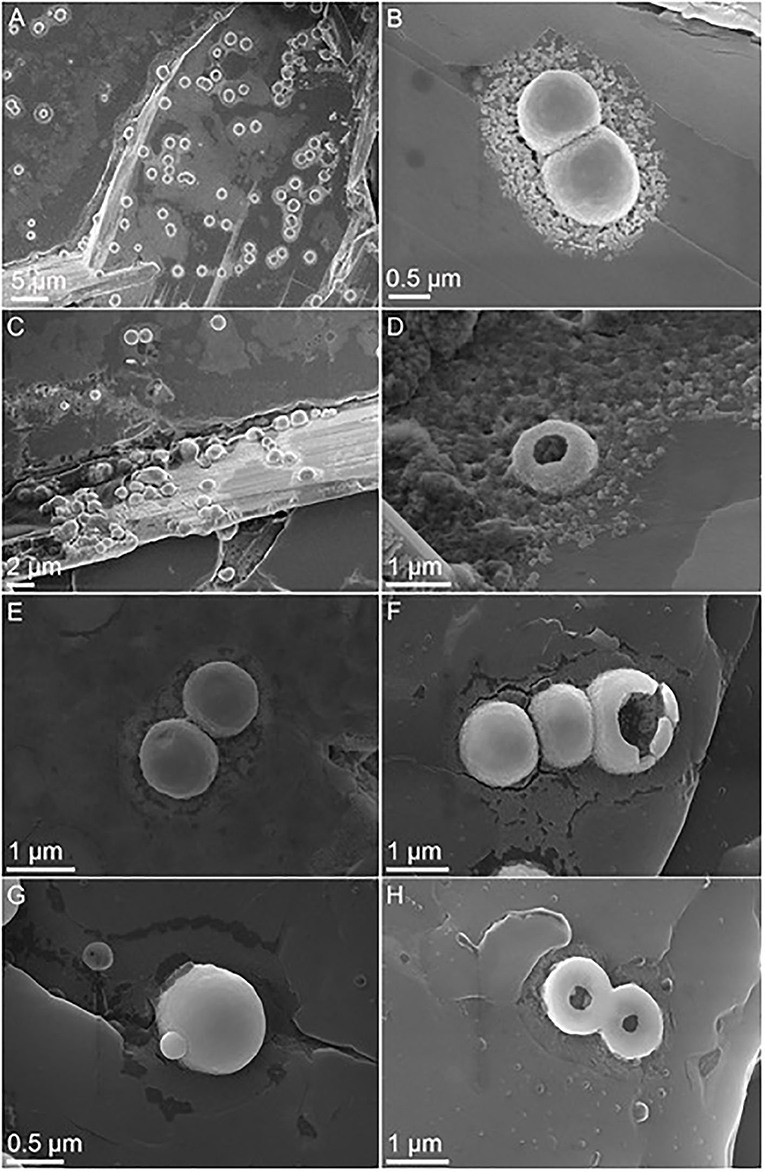
Figure 1. Scanning electron microscopy (SEM) images of desiccated M. sedula cells grown on tungsten (W) ore scheelite and preserved after 2 months of dehydration. (A,C) SEM images of dehydrated colonies of M. sedula cells grown on scheelite. (B) Magnified SEM image showing single scheelite-grown cells of M. sedula preserved after dehydration. (D–H) SEM images of intact cells of M. sedula maintaining the integrity after dehydration and broken dried cells of M. sedula revealing their cellular interior.
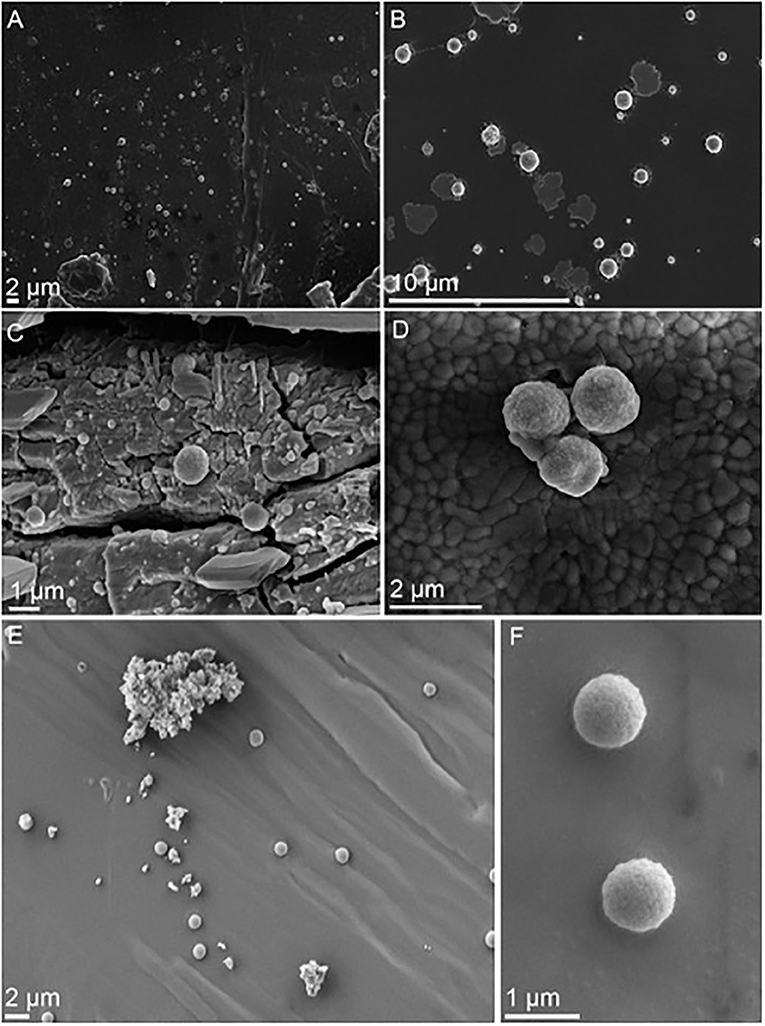
Figure 2. SEM images showing desiccated cells of M. sedula cultivated with W-POM and multimetallic waste material after 2 months of dehydration. (A–D) SEM images of dehydrated M. sedula cells cultivated with W-POM. (E,F) SEM images of dehydrated M. sedula cells cultivated with multimetallic waste material.
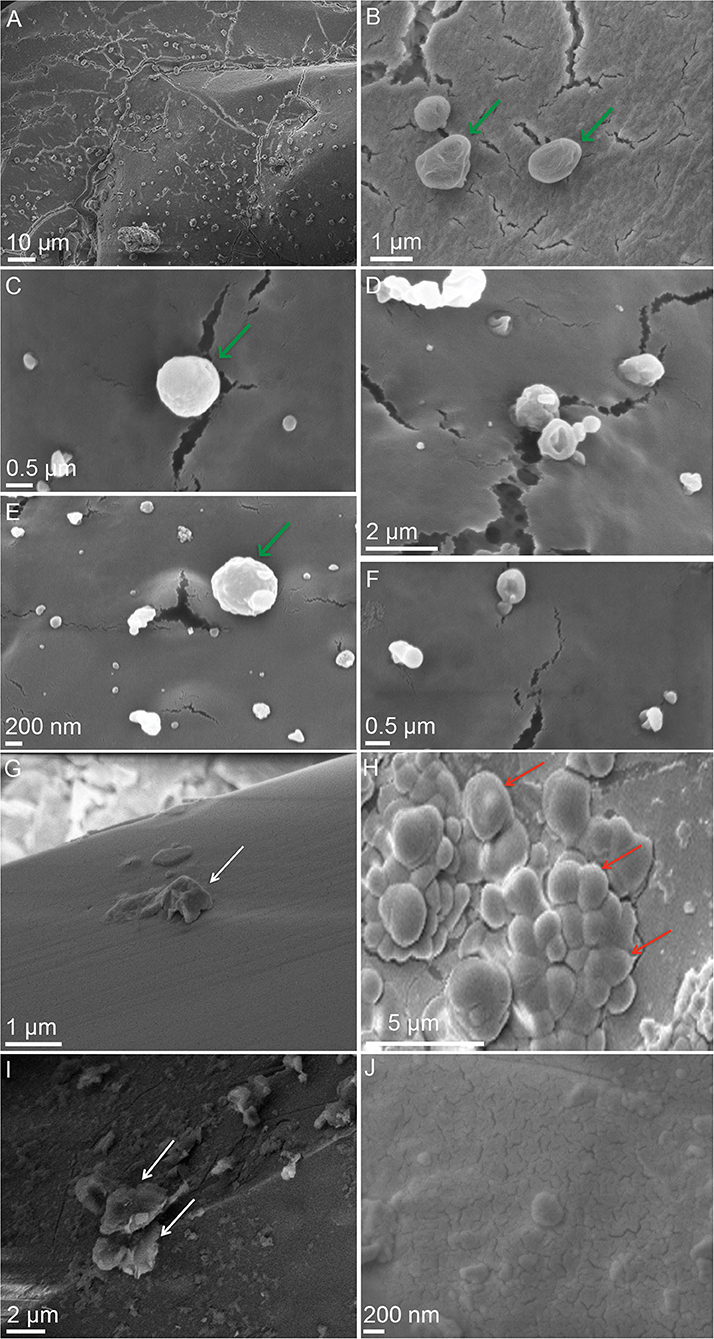
Figure 3. SEM images showing desiccated cells of M. sedula cultivated with the stony meteorite NWA 1172 and surfaces of Martian regolith simulants (MRSs) dehydrated for 2 months after cultivation with M. sedula. (A–F) SEM images of dehydrated M. sedula cells cultivated with NWA 1172. (B,C,E) Magnified SEM images showing single cells of M. sedula preserved after dehydration. (D,F) Magnified SEM images of broken dried cells of M. sedula revealing their cellular interior. (G–J) SEM images of MRSs dehydrated for 2 months after cultivation with M. sedula. (G) Scanning electron image showing a surface of precipitate obtained after the cultivation of M. sedula on JSC 1A. (H) Scanning electron image showing a surface of precipitate obtained after the cultivation of M. sedula on P-MRS. (I) Scanning electron image showing a surface of precipitate obtained after the cultivation of M. sedula on S-MRS. (J) Scanning electron image showing a surface of precipitate obtained after the cultivation of M. sedula on MRS07/52. M. sedula cells are shown with green arrows. White arrows indicate cell debris (e.g., cells and EPS remnants). Al-rich microspheroids are depicted with red arrows. Representative SEM-EDS analysis of carbon-rich cells, cell debris, and Al-rich microspheroids is provided in Supplementary Figure 2.
Preservation of Desiccated M. sedula Cells Grown on Tungsten (W) Ore Scheelite
SEM analysis revealed that after a long-term treatment under dehydration conditions (up to 2 months), most M. sedula cells grown on scheelite tungsten ore as the sole energy source (Blazevic et al., 2019) remained intact, did not show significant alteration in the overall morphology, and preserved their cellular integrity, maintaining the structural stability and exposing a fine-scale irregular surface (Figures 1A–C). The cells of M. sedula with budding vesicles attached to the cell surface occurred in this case as well (Figure 1G). To a minor extent, lysed and broken cells were clearly recognizable too, revealing their empty interior content (evidence of cell lysis, Figures 1D,F,H).
Preservation of Desiccated M. sedula Cells Grown on Tungsten Polyoxometalate (W-POM)
Dehydration by evaporation (up to 2 months) has been applied toward microbial cultures harvested after cultivation of M. sedula with W-POM (Milojevic et al., 2019a). The crystalline material obtained after dehydration was examined by SEM. Colonies of M. sedula cells were found attached to the surface of the obtained material (Figures 2A–D), revealing that after long-term treatment under dehydrating conditions, cells of M. sedula visually remained undamaged, did not show SEM-detectable alterations in the overall morphology, and preserved their cellular integrity in frames of SEM detection, exposing a fine-scale irregular surface (Figures 2C,D). Particularly in regard with W-POM grown cells, no broken cells or cells with a damaged cell surface were detectable after a long-term dehydration. Physical reorganization of cellular structures after dehydration did not occur, nor was structural evolution of the cell surface after the dehydration process observed in this case (Figure 2). In addition, the desiccated cells forming budding vesicles were recognizable too (Figure 2).
Preservation of Desiccated M. sedula Cells Grown on Multimetallic Waste Material
Cells were harvested after cultivation with multimetallic waste material as the sole energy source and subjected to dehydration by slow evaporation for up to 2 months. Extensive post-dehydration monitoring of these cultures was performed. SEM-assisted evaluation of the post-dehydration structural integrity revealed intact colonies of dried M. sedula cells attached to the surface of the obtained dehydrated material (Figures 2E,F). Single undamaged dried cells were inspected after a long-term treatment with dehydrating conditions. The cells did not show alterations in their overall morphology and preserved their cellular integrity, exposing a fine-scale irregular surface (Figures 2E,F).
Preservation of Desiccated M. sedula Cells Grown on the NWA 1172 Meteorite
Cultures of M. sedula grown on the stony meteorite Northwest Africa 1172 (NWA 1172; an H5 ordinary chondrite; Milojevic et al., 2019b) were subjected to a dehydration procedure for 60 days. Dehydration of M. sedula cultures by evaporation resulted in the formation of amorphous and crystalline materials. Our SEM analysis, performed after the dehydration period, displayed M. sedula cells deposited on the surface of the obtained amorphous and crystalline material (Figures 3A–F; see Supplementary Figure 2C for SEM-EDS analysis). Most of these desiccated cells remained intact on the level of SEM detection and visually preserved their cellular and structural integrity, exposing a fine-scale irregular surface (Figures 3B,C,E). Flourishing and dividing cells occurred as well; however, lysed and broken cells were clearly recognized too, revealing their empty interior content (evidence of cell lysis) (Figures 3D,F). Precipitated nanoglobules were detected attached to the surface of M. sedula cells in this case as well (Figures 3D,E).
Dehydration of M. sedula Grown on MRSs
Cultures of M. sedula grown on multimetallic MRSs as the sole energy sources (Kölbl et al., 2017) were subjected to dehydration by slow evaporation for 2 months at room temperature under atmospheric laboratory conditions. Neither preserved cells, nor cellular-like morphologies with broken, damaged, or lysed exterior were detected on the surface of dehydrated MRSs precipitates (Figures 3G–J). In the case of MRSs, we solely observed the formation of the aluminum/chlorine containing microspheroids published previously (Kölbl et al., 2017; Figure 3H and Supplementary Figure 2A). These hemispheroid formations were mostly composed of oxygen, chlorine, and aluminum and were characterized as nearly carbon-free metal inclusions, thus excluding the cellular nature of these morphologies (Kölbl et al., 2017; Figure 3H and Supplementary Figure 2A). Apart from aluminum/chlorine containing microspheroids, the deposition of cell debris materials was frequently detected in dehydrated cultures of M. sedula grown on MRSs (Figures 3G,I and Supplementary Figure 2B).
Survivability of M. sedula After Desiccation
The cells of M. sedula grown on various metal-bearing substrates after desiccation treatment for 2 months were transferred into the culture medium and were allowed to recover heterotrophically for 120 h. The cells of M. sedula grown and desiccated on NWA 1172, multimetallic waste, and tungsten-bearing materials (scheelite and W-POM) possess the ability to survive desiccation (Figure 4). The cells of M. sedula grown on four MRSs (JSC-1A, P-MRS, S-MRS, and MRS 07/52) showed impeded recovery after desiccation treatment when compared to NWA 1172, multimetallic waste, and tungsten-bearing materials scheelite and W-POM (Figure 4). Overall, heterotrophic re-cultivation of desiccated M. sedula cells on NWA 1172 yielded almost 2-fold higher cell number after 120 h compared to multimetallic waste, scheelite, and W-POM, showing a comparable re-cultivation capacity of cells grown on these substrates. The reduction of cell number after the desiccation treatment (“0” time point) and the subsequent impeded recovery of cells (120 h) was observed with all four types of tested MRSs (Figure 4). The cells grown and desiccated on MRS-07/52 exhibited the highest recovery among all four types of tested MRSs, which was however about two orders of magnitude lower than the recovery of the cells grown and desiccated on NWA 1172 and again, a 70- to 100-fold lower when compared to multimetallic waste and both tested tungsten-bearing materials (Figure 4). Among all examined metal-bearing substrates, the highest number of recovered cells after 120 h of post-desiccation incubation was observed in the case of NWA 1172 (Figure 4).
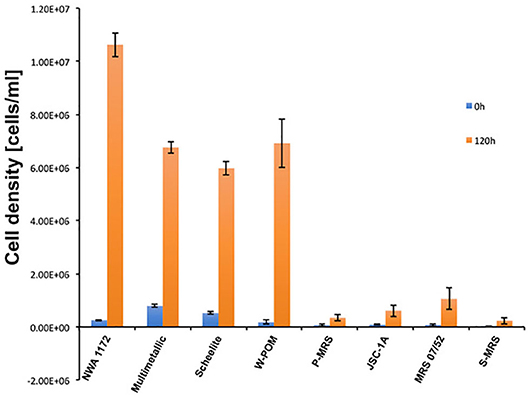
Figure 4. Cell densities of M. sedula cells recovered after desiccation for 2 months. Blue bars represent cell counts after immediate re-cultivation in heterotrophic medium (“0”), orange bars represent cell counts after 120 h of growth after re-culturing. Columns and error bars show the mean and error-represented standard deviation, respectively, of n = 3 biological replicates. If not visible, error bars are smaller than symbols.
Discussion
Our results showed that cells of M. sedula cultivated with certain metal-bearing materials (tungsten-bearing substrates, the stony chondrite meteorite NWA 1172, and multimetallic waste product) can be well-preserved (Figures 1–3) and recovered after dehydration up to 60 days under atmospheric conditions (Figure 4). Dehydrated cells of M. sedula cultivated on the solid metal-bearing materials were dried in a single cell layer to avoid microbial cell-aggregates, such that a “cell-by-cell shielding effect” expected in cellular multilayers is minimized. We have previously shown that metal incorporation by the cell surface of M. sedula leads to the formation of a metal crust around a cell (Blazevic et al., 2019; Milojevic et al., 2019a,b). Such metal-bearing crust might protect cell integrity, providing an additional barrier against complete water loss by the cell and thus retains a minimal level of the water phase, with implication for microbial preservation in severe dehydrated conditions. Various metal-containing precipitates and accumulations were reported previously on the S-layer of other extremophilic bacteria and archaea (Orange et al., 2011, 2014; Oggerin et al., 2013; Sanchez-Roman et al., 2015; Kish et al., 2016). Interestingly, cell surface deposition of metals and subsequent cellular encrustation does not necessarily lead to the cell entombment and death. Microorganisms capable of metal sorption and encrustation have developed strategies to deal with such micro-barriers. Recent work conducted with the archaeon S. acidocaldarius (closely related to M. sedula) demonstrated that this microorganism forms encrusted outer membrane vesicles when heavily encrusted with Fe-containing accumulations (Kish et al., 2016). This strategy is likely attributed to the removal of impaired S-layer proteins to enable substitution with new, precipitate-free proteins. Elimination of damaged encrusted S-layer portions may serve as an important strategy for Sulfolobales members to regenerate their cell wall structure and survive in extreme environments.
Moreover, differences in preservation of dried M. sedula cells were observed depending on the nature of metal-bearing materials used for cultivation of this chemolithotroph. In contrast to cultures grown on tungsten-bearing substrates, the NWA 1172 meteorite, and multimetallic waste products, no cells after desiccation could be recovered or detected when M. sedula was cultivated on sulfide ores, elemental sulfur, molecular hydrogen (Beblo et al., 2009, 2011), volcanic glass (Supplementary Figure 3), and synthetic extraterrestrial materials such as MRSs (Figures 3G–J). The chemolithotrophic growth of M. sedula on all these metal-bearing matrixes has been well documented (Huber et al., 1989; Auernik and Kelly, 2008, 2010a,b; Kölbl et al., 2017; Blazevic et al., 2019; Milojevic et al., 2019a,b). Similar to our observations, Beblo et al. (2009) also reported no survival after long-term desiccation of M. sedula grown on elemental sulfur, sulfide ores, or on solfatara sand. In these cases, it is possible that even when cells of M. sedula were subjected to metal sorption, the composition of these metal-containing substrates did not provide a sufficient protection layer to resist dehydration. The observed selective preservation of M. sedula cells after desiccation in these cases can be explained by a discriminating “shielding by metal nano-precipitates” effect. Apparently, only certain cell wall–metal interactions can strengthen prokaryotic cell envelopes and augment their structural stability implicating in cell preservation during harsh long-term dehydration. In this regard, the tungsten incorporation by the cell envelope of M. sedula (Blazevic et al., 2019; Milojevic et al., 2019a) may certainly serve as an efficient strengthening strategy, as this is a hard element with the highest melting point and extraordinary properties among all metals. Being suitable for high-temperature applications in energy and lighting technology, and in the space industry, it is also used as alloys, superalloys, and radiation-shielding. We have previously reported that M. sedula (cultivated on scheelite and W-POM) mineralizes its S-layer via encrusting with crystalline nanoparticles containing tungsten carbide-like structures (Blazevic et al., 2019; Milojevic et al., 2019a). Tungsten exhibits a hardness of ~9–9.5 on the Mohs hardness scale (Tabor, 1954) and can potentially provide an efficient barrier against water loss, warranting preservation of cell integrity after desiccation. M. sedula cells grown on the NWA 1172 meteorite are also heavily mineralized with the amorphous crust containing a CuxFeyOz(SPNiAl)–SiO2 product (Milojevic et al., 2019b). This multimetallic crust of mixed Ni/Al/Si content might have implications for the preservation of desiccated cells. Growth on elemental sulfur and related sulfur-bearing substrates (Beblo et al., 2009, 2011) as energy sources would imply sulfur incorporation by S-layer of M. sedula. Sulfur is a soft and very brittle material [2 on the Mohs scale of mineral hardness Tabor, 1954]. Therefore, sulfur-bearing crust might not provide sufficient mechanic protection against desiccation and sulfur-supplemented growth represents a contrasting case in which the substrate does not provide the required raw materials for defense against desiccation stress.
In environments of low organic carbon content, high temperature, acidic pH, and iron-rich surroundings, microorganisms face multiple challenges maintaining their cell population and growth. The four MRSs used in this study were modeled to represent global Martian regolith chemistry and are therefore limiting possible microbial–mineral interactions compared to natural minerals (e.g., NWA 1172, scheelite ore). In addition, these synthetic mixtures do not take Mars' actual Fe2+/Fe3+ ratios into account (Ramkissoon et al., 2019), generating regolith simulants high in Fe3+ rather than abundantly detected and metabolically feeding Fe2+ on the planet's surface (Boynton et al., 2008; Nixon et al., 2013). Elevated cultivation temperatures for M. sedula, the high ferric iron content, and the synthetic and therefore trace metal-depleted nature of the used MRSs (Supplementary Table 1) could have played a major role in the degradation of organic matter, since organic carbon is thermodynamically unstable in the presence of abundant Fe3+ (Sumner, 2004; Hays et al., 2017), even though there is evidence for organic matter-Fe coprecipitation and conservation over extended timescales (Lalonde et al., 2012). Our SEM observations (Figures 3G–J) and subsequent re-culturing under heterotrophic conditions show that thermoacidophilic M. sedula cells were not entombed on the surface of the MRSs mineral mixtures under the given experimental circumstances.
Apart from abundant Fe3+ content, additional reasons may contribute to the observed low efficiency of MRSs in the preservation of dehydrated cells. Elemental and mineralogical comparison of MRSs with genuine extraterrestrial and terrestrial materials shows that natural minerals (e.g., NWA 1172 and scheelite) exhibit a rich and complex mixture of elements in bulk as well as in trace concentrations (Supplementary Table 1). After cultivation of M. sedula on the ordinary chondrite NWA 1172, HAADF-STEM analysis detected complex mixed mineral phases (e.g., Cu, Fe, and Al) encasing individual cells (Milojevic et al., 2019b). Such complexity of encapsulated cell wall may contribute to cellular preservation under dehydrating conditions in the case of NWA 1172. Furthermore, NWA 1172 is characterized by much pronounced Mg and Ni elemental content (Supplementary Table 1) and its microbially mediated biotransformation leads to the formation of magnesium and nickel sulfates MgSO4x7H2O and NiSO4x6H2O (Milojevic et al., 2019b). These sulfate salts may assert their kosmotropic effect, bringing order to the surrounding solution by accumulating several water layers around them and acting as potential protein (organic matter) stabilizers under desiccation stress conditions (Okur et al., 2017; Kang et al., 2020). On the contrary, each regolith simulant lacks an authentic composition and subsequently diminishes the possibilities of more complex mineral–microbe interactions. Since growth of M. sedula on simulated Martian regolith was successful and the hydrogeological and iron-rich makeup of Mars could have supported iron and/or sulfur-transforming, chemolithoautotrophic microorganisms at one point, the identification of their putative (iron-) relevant fingerprints is of general interest (Amils et al., 2011; Nixon et al., 2012; Kölbl et al., 2017). Despite not being able to withstand dehydrating conditions in this study, we suggest that further investigations with alternative MRSs/analogs varying in overall and iron chemistry [reviewed by Ramkissoon et al. (2019)] should be performed. Since M. sedula cells were preserved after growth and desiccation on the meteorite NWA 1172, which exhibits a similar chemical composition to synthetic MRSs, most valuable would be the investigation of chemolithotrophs grown on real Martian meteorite materials.
Metal-organic associations in the fossil record are key indicators of past life and can be crucial in assessing whether microstructures found in rocks are of biological origin. Metabolic activity of certain microorganisms leads to mineral precipitation extracellularly; however, in metal-laden environments, e.g., associated with contamination or natural enrichments of S, Fe, Mn, and other metals, cells are subjected to metal sorption and mineral nucleation followed by metal encrustation of the cell surface (Schultze-Lam et al., 1996). Cell surface biomineralization can serve as a powerful microbial fingerprint and potential biosignature for the presence and/or activity of metal-transforming microorganisms. Assigning biogenicity to a certain structure or signature in ancient rocks remains very challenging due to probable degradation of microbial remains during diagenesis or microbial-like morphologies being produced abiotically (Gaboyer et al., 2017). The heavily encrusted desiccated cells depicted in our study may serve as relevant biosignatures to be looked for in the geological record, if they are not destroyed during diagenetic or metamorphic processes and are intact during different stages of fossilization. With regard to the subject of the preservation potential of desiccated microbes, many fascinating questions remain to be answered, including the influence of complex environmental parameters (e.g., the environmental chemistry, the rapidity of mineral encapsulation, and various post-diagenetic factors) on biomineralized cell wall. Such investigations may serve in establishing mineralogical and morphological criteria for the identification of metal-containing microfossils. The results of our study also suggest that desiccation-resistant and heavily encrusted cell walls might be identified in other representatives of archaeal order Sulfolobales, including fossil species where the S-layer could be preserved due to biomineralization, and remain intact.
Conclusion
Communities of archaea grown on tungsten-bearing materials, the NWA 1172 meteorite, and a multimetallic waste product provide well-preserved and recoverable cells of M. sedula under dehydrating conditions with NWA 1172 grown cells exposing the highest number of recovered cells after the desiccation treatment. Preservation of desiccated M. sedula cells described in the present study appears to be a discriminatory process, which depends on the nature and content of metal-bearing source used for growth of this metal-oxidizing archaeon. Our research report emphasizes the importance of considering microorganisms in their geological/mineralogical setting during investigations of geobiological environmental constrains. More importantly, the preservation of dehydrated M. sedula cells on metal-bearing substrates described herein suggests that this polyextremophilic archaeon is an ideal candidate for further survivability studies during future space exposure experiments and under the simulated space environmental conditions, including the testing of M. sedula persistence in a vacuum and after a combination of multiple stressors, for example, vacuum conditions combined with UV and gamma irradiation.
Data Availability Statement
The raw data supporting the conclusions of this article will be made available by the authors, without undue reservation.
Author Contributions
DK, AB, MA, and TM performed experiments. DK and TM performed, planned, and interpreted experiments described in this article. All authors provided editorial contribution to the manuscript, critically revised the report, and accepted the final version.
Funding
This study was supported by the Austrian Science Fund (FWF) with an Elise-Richter Research fellowship V333 Iron- and Sulfur-oxidizing Machinery of the bioleaching Archaeon Metallosphaera sedula.
Conflict of Interest
CF was employed by company Voestalpine BÖHLER Edelstahl GmbH & Co KG, Austria.
The remaining authors declare that the research was conducted in the absence of any commercial or financial relationships that could be construed as a potential conflict of interest.
Acknowledgments
We warmly thank Stephan Puchegger (University of Vienna, Physics Faculty Center for Nano Structure Research) for the support of electron microscopy investigations. Christian Koeberl, Ludovic Ferrière, and Franz Brandstätter (Natural History Museum of Vienna, Austria) are deeply acknowledged for providing the NWA 1172 meteorite sample and fruitful discussions. The authors would also like to thank Steffen Schmidt (Wolfram Bergbau und Hütten AG) for providing scheelite and Felix W. von Aulock (School of Environmental Sciences, University of Liverpool) for providing volcanic glass.
Supplementary Material
The Supplementary Material for this article can be found online at: https://www.frontiersin.org/articles/10.3389/fspas.2020.00041/full#supplementary-material
References
Amils, R., González-Toril, E., Aguilera, A., Rodríguez, N., Fernández-Remolar, D., Gómez, F., et al. (2011). From Río Tinto to Mars. Adv. Appl. Microbiol. 77, 41–70. doi: 10.1016/B978-0-12-387044-5.00002-9
Auernik, K. S., and Kelly, R. M. (2008). Identification of components of electron transport chains in the extremely thermoacidophilic crenarchaeon Metallosphaera sedula through iron and sulfur compound oxidation transcriptomes. Appl. Environ. Microbiol. 74, 7723–7732. doi: 10.1128/AEM.01545-08
Auernik, K. S., and Kelly, R. M. (2010a). Physiological versatility of the extremely thermoacidophilic archaeon Metallosphaera sedula supported by transcriptomic analysis of heterotrophic, autotrophic, and mixotrophic growth. Appl. Environ. Microbiol. 76, 931–935. doi: 10.1128/AEM.01336-09
Auernik, K. S., and Kelly, R. M. (2010b). Impact of molecular hydrogen on chalcopyrite bioleaching by the extremely thermoacidophilic archaeon Metallosphaera sedula. Appl. Environ. Microbiol. 76, 2668–2672. doi: 10.1128/AEM.02016-09
Bader, M., Muller, K., Foerstendorf, H., Drobot, B., Schmidt, M., Musat, N., et al. (2017). Multistage bioassociation of uranium onto an extremely halophilic archaeon revealed by a unique combination of spectroscopic and microscopic techniques. J. Hazard. Mater. 327, 225–232. doi: 10.1016/j.jhazmat.2016.12.053
Beblo, K., Douki, T., Schmalz, G., Rachel, R., Wirth, R., Huber, H., et al. (2011). Survival of thermophilic and hyperthermophilic microorganisms after exposure to UV-C, ionizing radiation and desiccation. Arch. Microbiol. 193, 797–809. doi: 10.1007/s00203-011-0718-5
Beblo, K., Rabbow, E., Rachel, R., Huber, H., and Rettberg, P. (2009). Tolerance of thermophilic and hyperthermophilic microorganisms to desiccation. Extremophiles 13, 521–531. doi: 10.1007/s00792-009-0239-1
Beveridge, T. J. (1989). Role of cellular design in bacterial metal accumulation and mineralization. Annu. Rev. Microbiol. 43, 147–171. doi: 10.1146/annurev.mi.43.100189.001051
Blazevic, A., Albu, M., Mitsche, S., Rittmann, S. K.-M. R., Habler, G., and Milojevic, T. (2019). Biotransformation of scheelite CaWO4 by the extreme thermoacidophile Metallosphaera sedula: tungsten–microbial interface. Front. Microbiol. 10:1492. doi: 10.3389/fmicb.2019.01492
Boynton, W. V., Taylor, G. J., Karunatillake, S., Reedy, R. C., and Keller, J. M. (2008). “Elemental abundances determined via the Mars Odyssey GRS,” in The Martian Surface: Composition, Mineralogy and Physical Properties, Cambridge Planetary Science, ed J. Bell (Cambridge: Cambridge University Press), 103–124. doi: 10.1017/CBO9780511536076.006
Chang, W.-S., van de Mortel, M., Nielsen, L., Nino de Guzman, G., Li, X., and Halverson, L. J. (2007). Alginate production by Pseudomonas putida creates a hydrated microenvironment and contributes to biofilm architecture and stress tolerance under water-limiting conditions. J. Bacteriol. 189, 8290–8299. doi: 10.1128/JB.00727-07
Contant, R., Klemperer, W. G., and Yaghi, O. (2007). “Potassium octadecatungstodiphosphates(V) and related lacunary compounds,” in Inorganic Syntheses, ed A. Ginsberg (New York, NY: John Wiley and Sons, Inc.), 104–111. doi: 10.1002/9780470132586.ch18
Ehrlich, H. L. (1999). Microbes as geologic agents: their role in mineral formation. Geomicrobiol. J. 16, 135–153. doi: 10.1080/014904599270659
Gaboyer, F., Le Milbeau, C., Bohmeier, M., Schwendner, P., Vannier, P., Beblo-Vranesevic, K., et al. (2017). Mineralization and preservation of an extremotolerant bacterium isolated from an early Mars analog environment. Sci. Rep. 7:8775. doi: 10.1038/s41598-017-08929-4
Gupta, P., and Diwan, B. (2017). Bacterial exopolysaccharide mediated heavy metal removal: a review on biosynthesis, mechanism and remediation strategies. Biotechnol. Rep. 13, 58–71. doi: 10.1016/j.btre.2016.12.006
Hays, L. E., Graham, H. V., Des Marais, D. J., Hausrath, E. M., Horgan, B., McCollom, T. M., et al. (2017). Biosignature preservation and detection in mars analog environments. Astrobiology 17, 363–400. doi: 10.1089/ast.2016.1627
Hickman-Lewis, K., Gautret, P., Arbaret, L., Sorieul, S., De Wit, R., Foucher, F., et al. (2019). Mechanistic morphogenesis of organo-sedimentary structures growing under geochemically stressed conditions: keystone to proving the biogenicity of some archaean stromatolites? Geosciences 9:359. doi: 10.3390/geosciences9080359
Hincha, D. K., and Hagemann, M. (2004). Stabilization of model membranes during drying by compatible solutes involved in the stress tolerance of plants and microorganisms. Biochem. J. 383, 277–283. doi: 10.1042/BJ20040746
Huber, G., Spinnler, C., Gambacorta, A., and Stetter, K. O. (1989). Metallosphaera sedula gen. and sp. nov. represents a new genus of aerobic, metal-mobilizing, thermoacidophilic archaebacteria. Syst. Appl. Microbiol. 12, 38–47. doi: 10.1016/S0723-2020(89)80038-4
Kang, B., Tang, H., Zhao, Z., and Song, S. (2020). Hofmeister series: insights of ion specificity from amphiphilic assembly and interface property. ACS Omega 5, 6229–6239. doi: 10.1021/acsomega.0c00237
Kish, A., Miot, J., Lombard, C., Guigner, J. M., Bernard, S., Zirah, S., et al. (2016). Preservation of archaeal surface layer structure during mineralization. Sci. Rep. 6:26152. doi: 10.1038/srep26152
Kölbl, D., Pignitter, M., Somoza, V., Schimak, M. P., Strbak, O., Blazevic, A., et al. (2017). Exploring fingerprints of the extreme thermoacidophile Metallosphaera sedula grown on synthetic martian regolith materials as the sole energy sources. Front. Microbiol. 8:1918. doi: 10.3389/fmicb.2017.01918
La Paglia, C., and Hartzell, P. L. (1997). Stress-induced production of biofilm in the hyperthermophile Archaeoglobus fulgidus. Appl. Environ. Microbiol. 63, 3158–3163. doi: 10.1128/AEM.63.8.3158-3163.1997
Lalonde, K., Mucci, A., Ouellet, A., and Gélinas, Y. (2012). Preservation of organic matter in sediments promoted by iron. Nature 483, 198–200. doi: 10.1038/nature10855
Li, J. H., Bernard, S., Benzerara, K., Beyssac, O., Allard, T., Cosmidis, J., et al. (2014). Impact of biomineralization on the preservation of microorganisms during fossilization: an experimental perspective. Earth Plan. Sci. Lett. 400, 113–122. doi: 10.1016/j.epsl.2014.05.031
Li, L., Connors, M. J., Kolle, M., England, G. T., Speiser, D. I., Xiao, X., et al. (2015). Multifunctionality of chiton biomineralized armor with an integrated visual system. Science 350, 952–956. doi: 10.1126/science.aad1246
Loaëc, M., Olier, R., and Guezennec, J. (1998). Chelating properties of bacterial exopolysaccharides from deep-sea hydrothermal vents. Carbohydr. Polym. 35, 65–70. doi: 10.1016/S0144-8617(97)00109-4
Maezato, Y., Johnson, T., McCarthy, S., Dana, K., and Blum, P. (2012). Metal resistance and lithoautotrophy in the extreme thermoacidophile Metallosphaera sedula. J. Bacteriol. 194, 6856–6863. doi: 10.1128/JB.01413-12
Milojevic, T., Albu, M., Blazevic, A., Gumerova, N., Konrad, L., and Cyran, N. (2019a). Nanoscale tungsten-microbial interface of the metal immobilizing thermoacidophilic archaeon Metallosphaera sedula cultivated with tungsten polyoxometalate. Front. Microbiol. 10:1267. doi: 10.3389/fmicb.2019.01267
Milojevic, T., Kölbl, D., Ferrière, L., Albu, M., Kish, A., Flemming, R. L., et al. (2019b). Exploring the microbial biotransformation of extraterrestrial material on nanometer scale. Sci. Rep. 9:18028. doi: 10.1038/s41598-019-54482-7
Moon, J. H., Lee, W., Park, J., Choi, K. H., and Cha, J. (2016). Characterization of a trehalose-degrading enzyme from the hyperthermophilic archaeon Sulfolobus acidocaldarius. J. Biosci. Bioeng. 122, 47–51. doi: 10.1016/j.jbiosc.2015.12.011
Mortel, M. V. D., and Halverson, L. J. (2004). Cell envelope components contributing to biofilm growth and survival of Pseudomonas putida in low-water-content habitats. Mol. Microbiol. 52, 735–750. doi: 10.1111/j.1365-2958.2004.04008.x
Mukherjee, A., Wheaton, G. H., Blum, P. H., and Kelly, R. M. (2012). Uranium extremophily is an adaptive, rather than intrinsic, feature for extremely thermoacidophilic Metallosphaera species. Proc. Natl. Acad. Sci. U.S.A. 109, 16702–16707. doi: 10.1073/pnas.1210904109
Nixon, S. L., Cockell, C. S., and Tranter, M. (2012). Limitations to a microbial iron cycle on Mars. Planet. Space Sci. 72, 116–128. doi: 10.1016/j.pss.2012.04.003
Nixon, S. L., Cousins, C. R., and Cockell, C. S. (2013). Plausible microbial metabolisms on Mars. Astron. Geophys. 54, 1.13–1.16. doi: 10.1093/astrogeo/ats034
Oehler, J. H., and Schopf, J. W. (1971). Artificial microfossils: experimental studies of permineralization of blue-green algae in silica. Science 174, 1229–1231. doi: 10.1126/science.174.4015.1229
Oggerin, M., Tornos, F., Rodriguez, N., del Moral, C., Sanchez-Roman, M., and Amils, R. (2013). Specific jarosite biomineralization by Purpureocillium lilacinum, an acidophilic fungus isolated from Rio Tinto. Environ. Microbiol. 15, 2228–2237. doi: 10.1111/1462-2920.12094
Okazaki, N., Tamada, T., Feese, M. D., Kato, M., Miura, Y., Komeda, T., et al. (2012). Substrate recognition mechanism of a glycosyltrehalose trehalohydrolase from Sulfolobus solfataricus KM1. Protein Sci. 4, 539–552. doi: 10.1002/pro.2039
Okur, H. I., Hladílková, J., Rembert, K. B., Cho, Y., Heyda, J., Dzubiella, J., et al. (2017). Beyond the Hofmeister series: ion-specific effects on proteins and their biological functions. J. Phys. Chem. B 121, 1997–2014. doi: 10.1021/acs.jpcb.6b10797
Orange, F., Chabin, A., Gorlas, A., Lucas-Staat, S., and Geslin, C. (2011). Experimental fossilisation of viruses from extremophilic Archaea. Biogeosciences 8, 1465–1475. doi: 10.5194/bg-8-1465-2011
Orange, F., Dupont, S., Le Goff, O., Bienvenu, N., Disnar, J. R., and Westall, F. (2014). Experimental fossilization of the thermophilic gram-positive bacterium Geobacillus SP7A: a long duration preservation study. Geomicrobiol. J. 31, 578–589, doi: 10.1080/01490451.2013.860208
Orange, F., Westall, F., Disnar, J. R., Prieur, D., Bienvenu, N., and Défarge, C. (2009). Experimental silicification of the extremophilic archaea Pyrococcus abyssi and Methanocaldococcus jannaschii: applications in the search for evidence of life in early earth and extraterrestrial rocks. Geobiology 7, 403–418. doi: 10.1111/j.1472-4669.2009.00212.x
Peeples, T. L., and Kelly, R. M. (1995). Bioenergetic response of the extreme thermoacidophile Metallosphaera sedula to thermal and nutritional stress. Appl. Environ. Microbiol. 61, 2314–2321. doi: 10.1128/AEM.61.6.2314-2321.1995
Ramkissoon, N. K., Pearson, V. K., Schwenzer, S. P., Schröder, C., Kirnbauer, T., Wood, D., et al. (2019). New simulants for martian regolith: controlling iron variability. Planet. Space Sci. 179:104722. doi: 10.1016/j.pss.2019.104722
Russell, S., Zipfel, J., Grossman, J., and Grady, M. (2002). The meteoritical bulletin no. 86. Meteorit. Planet. Sci. 37(suppl.), 157–184. doi: 10.1111/j.1945-5100.2002.tb00913.x
Sanchez-Roman, M., Puente-Sanchez, F., Parro, V., and Amils, R. (2015). Nucleation of Fe-rich phosphates and carbonates on microbial cells and exopolymeric substances. Front. Microbiol. 6:1024. doi: 10.3389/fmicb.2015.01024
Schultze-Lam, S., Fortin, D., Davis, B. S., and Beveridge, T. J. (1996). Mineralization of bacterial surfaces. Chem. Geol. 132, 171–181. doi: 10.1016/S0009-2541(96)00053-8
Seo, J. S., An, J. H., Cheong, J. J., Choi, Y. D., and Kim, C. H. (2008). Bifunctional recombinant fusion enzyme between maltooligosyltrehalose synthase and maltooligosyltrehalose trehalohydrolase of thermophilic microorganism Metallosphaera hakonensis. J. Microbiol. Biotechnol. 9, 1544–1549.
Sumner, D. Y. (2004). Poor preservation potential of organics in meridiani planum hematite-bearing sedimentary rocks. J. Geophys. Res. 109:E12007. doi: 10.1029/2004JE002321
Tabor, D. (1954). Mohs hardness scale – a physical interpretation. Proc. Phys. Soc. B, 67, 249–257. doi: 10.1088/0370-1301/67/3/310
van Gemerden, H. (1986). Production of elemental sulfur by green and purple sulfur bacteria. Arch. Microbiol. 146, 52–56. doi: 10.1007/BF00690158
Keywords: desiccation, meteorite, Metallosphaera sedula, metals, SEM
Citation: Kölbl D, Blazevic A, Albu M, Fasching C and Milojevic T (2020) Desiccation of the Extreme Thermoacidophile Metallosphaera sedula Grown on Terrestrial and Extraterrestrial Materials. Front. Astron. Space Sci. 7:41. doi: 10.3389/fspas.2020.00041
Received: 22 April 2020; Accepted: 16 June 2020;
Published: 11 August 2020.
Edited by:
Daniela Billi, University of Rome Tor Vergata, ItalyReviewed by:
Keyron Hickman-Lewis, University of Bologna, ItalyBeatrice Cobucci-Ponzano, Institute of Bioscience and Bioresources (CNR), Italy
Copyright © 2020 Kölbl, Blazevic, Albu, Fasching and Milojevic. This is an open-access article distributed under the terms of the Creative Commons Attribution License (CC BY). The use, distribution or reproduction in other forums is permitted, provided the original author(s) and the copyright owner(s) are credited and that the original publication in this journal is cited, in accordance with accepted academic practice. No use, distribution or reproduction is permitted which does not comply with these terms.
*Correspondence: Tetyana Milojevic, dGV0eWFuYS5taWxvamV2aWNAdW5pdmllLmFjLmF0