- Max Planck Institute for extraterrestrial Physics, Garching, Germany
Plasma injection experiments in space are being ordered according to five aspects: (1) Diagnostics of electric fields, (2) Coupling to the ionosphere, (3) Interactions with the solar wind, (4) Modification experiments, and (5) Special physical processes. Historically first were releases of neutral gases with the aim to measure atmospheric parameters. They were soon followed by plasma injections applied to the measurement of plasma flows and parallel electric fields. Long-range coupling to the environment was a most important aspect of the plasma releases. It concerned, on the one hand, the need for corrections of the derived diagnostic parameters and, on the other hand, the understanding of the formation of the ubiquitous striations and deformations of the plasma clouds. A special application was the investigation of cometary interactions by releases in the solar wind. Modification experiments in the ionosphere were done intentionally or occurred as byproducts of rocket launches or other activities. A particular goal was to trigger natural large-scale ionospheric instabilities like equatorial spread F in order to improve the understanding of the natural phenomena. Large-scale plasma injections in the magnetosphere have been performed in order to change the conditions of wave-particle interactions and potentially trigger observable effects. Special goals were so-called skidding experiments and testing Alfvén's critical ionization velocity effect. In this review, we will emphasize the principle objectives and illustrate the results from selected experiments.
Diagnostics of Electric Fields by Tracing Visible Plasma Clouds
It was Bates (1950), who made the first proposal to release metallic and molecular vapor clouds in the upper atmosphere as a tool to measure atmospheric parameters and excitation processes. Beginning in the mid-fifties the idea was taken up by various groups in the United States (Edwards et al., 1956), Australia (Groves, 1960; Rees, 1961), and France (Blamont et al., 1960). A large number of elements were employed in chemical releases, metallic atoms such as Li, K, Na, Sr, Ba, Al, and compounds such as NO, NO2, SF6, AlO, and BaO. The main goal was the investigation of diffusion processes, wind profiles, density, turbulence, and temperature. The technique had the advantage of relative technical simplicity, short preparation times, and many novel insights into a hitherto little known territory. A short overview can be found in Harang (1969).
This review will not deal with the accomplishments pertaining to the neutral atmosphere, but concentrate on the exploration of the near-Earth plasma environment. It soon turned out that the injection barium vapor clouds was the most efficient tool. The first successful releases of barium were made by Armstrong (1963) at the Weapons Research Establishment in Woomera, Australia. The releases were carried out at heights of only 100 km and led to barely detectable ion emissions not yet exhibiting strong coupling to the magnetic and electric fields. However, it was to be expected that the groups working with chemical releases from sounding rockets would soon widen the application to the plasma realm. The main push came from a completely different direction.
It was his study of cometary plasma tails as natural probes of the state of the interplanetary medium what let Ludwig Biermann at the Max Planck Institute for Physics and Astrophysics in Munich propose to perform release experiments suited to create conditions similar to those found in cometary ion tails (Biermann et al., 1961). His proposal fortuitously coincided with the decision of the Federal Republic of Germany in 1961 to enter space research and to charge Biermann's Institute for Astrophysics with that task. Reimar Lüst, although a theorist, was asked to form the first German team dedicated to space research. Taking up Biermann's proposal of creating an “artificial comet” as the first goal, promised to be a quick start. It was of course not regarded necessary to employ materials as found in comets, such as CO+, N2+, etc. Materials with low photo-ionization potential and resonance lines in the visible spectral range, such as Sr and Ba, appeared to be the best choice. It was of invaluable help that Jacques Blamont offered the group of Reimar Lüst space on his sounding rockets. The first experiments in 1963 with mixtures of barium oxide and aluminum turned out not to produce significant amounts of free Ba atoms (Föppl et al., 1965). In late 1964, however, at experiments carried out on flights from Hammaguir in the Sahara desert, the observers saw for the first time substantial emissions of Ba I and Ba II (Föppl et al., 1967).
The first experiments were typically performed at heights of 150 km and primarily dedicated to finding the most efficient chemical reaction. Instead of the conventional thermite mixtures, using the reaction:
with n chosen above the stoichiometric equivalent to the amount of CuO, turned out to be the best choice. It became the standard mix for practically all subsequent experiments. A second most helpful result from these first experiments was the finding that photoionization of atomic barium was much faster than calculated in Föppl et al. (1965). Later experiments at higher altitude allowed to determine the true time-scale of nearly 30 s. The explanation was found by Haser (1967), namely a two-step process involving excitation of two metastable levels and ionization from there by the more intense solar UV emissions (Drappatz, 1972; Carlsten, 1975).
At the same time, Haerendel et al. (1967) addressed the relation between the observed motion of a Ba+ cloud and the transverse electric field. The essential point is that the modification of the electric field by the locally enhanced Pedersen conductivity is reduced by current exchange between the more highly conducting lower ionosphere and the weakly conducting cloud. Crucial is the ratio, , between the integrated Pedersen conductivity, ΣP, inside the whole flux tube pervading the cloud (index 2) and that at the outside (index 1). The ratio κibetween the gyro- and collision frequencies for the barium ions is typically well above unity for clouds generated in the middle or even upper F region. For the E region κi ≈ 1. Figure 1 depicts the situation. It shows a deviation of part of the E region Pedersen currents through the barium cloud by exchanging field-aligned currents. Thereby the contribution of the E layer to the electric polarization field is being reduced mitigating its enhancement by the presence of the barium plasma. Since the cloud is finite in three dimensions, also the Hall conductivity is of importance. However, in most cases it can be safely assumed that the Ba-cloud adds very little to it. Furthermore, the neutral wind velocity at cloud level, vn, enters into the relation derived for the transverse electric field, E⊥, the quantity of prime interest. Assuming that, owing to the high parallel conductivity, magnetic field lines are equipotentials, is a good approximation within the ionosphere. The relation connecting the observed cloud motion, V⊥, with the transverse electric field:
could be used in two ways. On the one hand, it allowed for the first a reliable determination of magnetospheric electric fields and, on the other hand, served for estimating the contribution of polarization fields. The latter are the cause of the frequently evolving distortions of the clouds by creating divergences of the Pedersen current in the E region and secondary conductivity changes thus modulating the E × B drift of the barium ions. Of course, the external electric field can be quite inhomogeneous from the outset, in particular in the auroral magnetosphere. In the following we will first concentrate on the first products of the barium cloud technique, the insights obtained into the magnetospheric electric fields, and address distortions and fine structures in section Distortions and Striations.
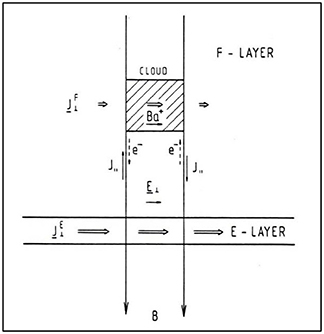
Figure 1. Interaction of a finite cylindrical cloud with the lower ionosphere. Horizontal arrows, , are ion currents, the suffix 2 referring to field-lines passing through the cloud. Vertical arrows, , are field-aligned electron currents, and the electric field. The cartoon shows that part of the E region Pedersen current is rerouted through the barium cloud by exchanging field-aligned currents. This leads to a reduction of the polarization field caused by the enhanced total conductivity and thus affects the observable motion of the cloud (Haerendel et al., 1967).
In the late 60s and early 70s direct electric field measurements were still in the development phase. Electric fields derived from Ba cloud motions provided the first trustworthy information about the convection of the magnetospheric plasma, albeit restricted to the times of sunrise and sunset due to the experimental conditions. A summary of the first years' experiments, not only by the Max Planck group, but augmented by similar experiments taken up by the University of Alaska (Wescott et al., 1969, 1970) and Goddard Space Flight Center (Heppner, 1971), is displayed in Figure 2 (from Haerendel, 1972). It exhibits the eastward and westward convection at high magnetic latitudes separating at a local time near 22:00. On the polar cap the sense appears to be reversed. Separating the flow vectors according to geomagnetic conditions showed already the typical ordering which was to be fully elaborated in subsequent years, both by interpreting ground magnetic perturbations in terms of overhead Hall currents (e.g., Heppner, 1969) and directly from electric field measurements by double probes (e.g., Mozer and Bruston, 1967; Aggson, 1969). Not being restricted to twilight conditions and a few launch sites, the latter methods made these products of chemical release experiments on the long run obsolete. However, simultaneous flights of double probes and plasma cloud experiments proved very useful for intercalibration of the two methods (Fahleson et al., 1971; Kelley et al., 1975).
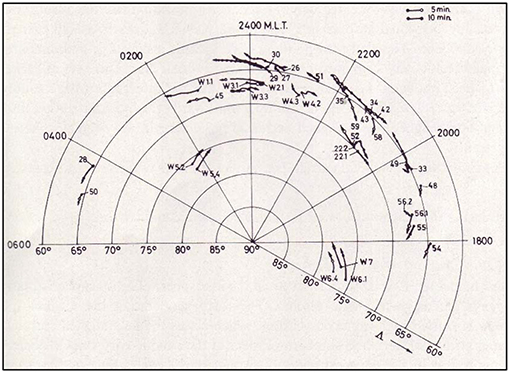
Figure 2. Summary of the paths of barium clouds projected into an invariant latitude-magnetic local time plane. Open circles end a 5 min interval, full circles a 10 min interval (Haerendel, 1972). Paths labeled W refer to data from Wescott et al. (1969, 1970) and Heppner (1971). It exhibits the eastward and westward convection at high magnetic latitudes separating at a local time near 22:00 and a reversed sense of convection on the polar cap.
Barium cloud experiments in the ionosphere had quickly become very popular. Besides the above mentioned groups also the Air Force Cambridge Research Laboratories (AFGRL) (Rosenberg, 1971) took up this technique already in 1967. Experiments were not only performed at high magnetic latitudes but also at mid-latitudes and near the magnetic equator. Useful insights were obtained, as for instance into the driver of the Sq-current system. A collection of data from releases at mid-latitudes provided electric field measurements supporting the dynamo theory of Stewart (1882) with the neutral wind as driver (Haerendel and Lüst, 1968a). Releases from Thumba/India confirmed the peculiar behavior of the equatorial ionosphere at sunset and sunrise as inferred from incoherent scatter data. We will return to this topic in section Modification Experiments.
There are, however, situations in which plasma cloud experiments can yield unique insights. One of them is the motions and distortions of the seeded plasma in the neighborhood of auroral arcs. Furthermore, propagation or expansion parallel to the magnetic field can at times yield valuable information on the magnetic field direction and its variation, and most importantly, on parallel acceleration. A few results will be discussed.
Relative Motions of Plasma Clouds and Auroral Arcs
A fascinating variety of situations has been met when barium plasma clouds were injected in the neighborhood of auroral arcs. They ranged between parallel alignments of the cloud paths with the orientation of the arcs and greatly different relative motions, including crossings of the two. The first type of observations was made during magnetic quiet conditions and revealed that the arcs were embedded in the general plasma convection. The electric field was typically found to point normal to the arcs (Wescott et al., 1969). The second type of findings was obtained during strong magnetic activity. It transpired that the source of energy injected into the arcs was not frozen into the magnetospheric plasma but originated from intrusions of energy arriving from progressively further poleward.
Figure 3 documents a striking example of the latter. The event resulted from the injection of a barium jet along B from 540 km altitude by means of a shaped charge. It was performed in the dusk sector during an ongoing substorm further east (Wescott et al., 1975). The Ba+ jet traveling upward to high altitudes had already split into several east-west separated streaks, when the aurora south of them was activated, forming a spiral structure and propagating poleward opposite to the equatorward drift of barium streaks. Crossing by the aurora had no effect on the plasma convection as manifested by the barium plasma. Another example occurred during a substorm about 3 h before magnetic midnight (Kelley et al., 1975). It was astounding how fast a rather irregular arc propagated poleward across the south-easterly drifting barium clouds. Both cases demonstrated the same facts, namely that the influx of new energy into the magnetosphere during substorms (and possibly also at other occasions) proceeded at progressively further poleward located flux tubes. However, it must be noted that, at the time of these experiments, the understanding of the connection to events at the outer boundary of the magnetosphere was at best rudimentarily developed.
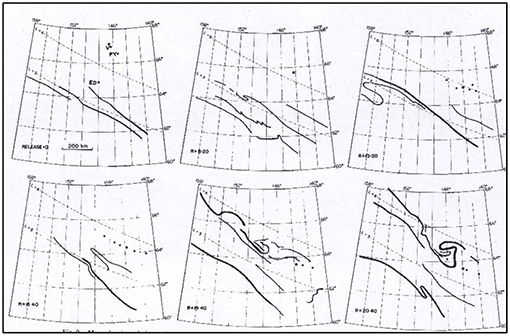
Figure 3. Maps showing relative positions of the aurora and the 100 km projected positions of individual barium streaks. The initial release locations are at Venetie (V), Ester Dome (ED), and Fort Yukon (FY). After 16 min a substorm starts with new arcs expanding poleward and intercepting the equatorward moving barium streaks [Reproduced from (Wescott et al., 1975) with permission of John Wiley and Sons].
The distribution of the energy injected into the magnetosphere from the tail is accompanied by different types of aurora. Even today not all of them are understood. The finding that some Ba+ clouds were moving more-or-less parallel to an arc was not confirmed by other experiments. Often path or elongation axis of the Ba+ clouds formed substantial angles with the auroral arcs (e.g., Haerendel et al., 1969). Later investigations substituting plasma clouds by incoherent scatter measurements of plasma drifts revealed the quite normal existence of proper motions between arcs and the environmental plasma (Haerendel et al., 1993). Such proper motions demonstrate that maintenance of the energy supply requires propagation of the arc into the energy reservoir constituted by sheared magnetic fields. This connection had been first proposed by the author in 1980 and subsequently elaborated in several papers (see Haerendel, 2007).
Field-Aligned Current in the Outer Magnetosphere
In 1969, the European Space Research Organization ESRO launched its first highly eccentric satellite, HEOS 1. It carried among others a barium release canister. It was ignited in March 1969 at a distance of 12. RE (Haerendel and Mende, 2012). Closer look at the orientation of the long axis, obtained by triangulation from widely separated ground stations, showed a significant variation during the half-hour visibility. Further look into the onboard magnetometer data and inspection of the ground magnetic perturbations in the foot area in the neighborhood of Godhavn/Greenland revealed in-phase variations in the ionosphere. This was the first simultaneous measurement of field-aligned electric currents between the outer magnetosphere and the ionosphere (Haerendel et al., 1971), at a time when one had just begun to find signatures of j|| from magnetic field measurements at low-orbiting satellites (Zmuda et al., 1966, 1970). This example just exhibits one of the pioneering aspects of the plasma injection experiments in space with, of course, little sustainable value.
A later example of the above was the observation by Wescott et al. (1975) that the orientation of barium streaks produced by shaped-charge injections deviated appreciably from the magnetic field model. This was attributed to strong field aligned sheet currents flowing nearby and even allowed determination of their magnitude.
Field-Parallel Acceleration
The essence of auroral arcs is the conversion of free magnetic energy into kinetic and thermal energy of accelerated electrons and ions. Theories of the related existence of parallel potential drops were developed starting in the late 60's (Block, 1972; Swift et al., 1976) based on measurements of strongly field-aligned auroral electron distributions above auroral arcs (McIlwain, 1960; Evans, 1974). It was therefore most desirable to somehow succeed injecting Ba+ ions into an auroral acceleration region. Not only did it require long waiting periods before conditions suited for a barium injection experiment close to an aurora were met, but hitting an acceleration region required multiple tries and was more or less a matter of luck. Since the acceleration regions extended from about 2,000 km up to and above 8,000 km, Brunner et al. (1970) from MPE and Wescott et al. (1972) from the University of Alaska independently developed the shaped charge injection of barium jets propagating with peak velocities of about 14 km/s and thus capable of probing heights of up to 30 000 km. An experiment with this technique from Søndre Strømfjord/Greenland in January 1975 turned out to be a lucky occasion (Haerendel et al., 1976). It was aided by the intrinsic proper motions of the aurora relative to the background and the injected barium jet. Initially located in an area of scattered auroral arcs, it expanded upward according to the adiabatic motion of ions. After 12 min an auroral arc appeared in the neighborhood of the jet. The low-velocity part of the jet, which meanwhile had sedimented to an altitude of 260 km, showed strongly enhanced transverse electric fields. For a short while, the jet was lost from observation because of the decreasing brightness of the strongly elongated streak. It could be recovered, but only by the most sensitive TV camera at the Thule observing site. Lacking proper triangulation the height distribution was determined by fitting with model field lines. However, distortion of the field by neighboring field-aligned currents introduced substantial uncertainties which are indicated in the data presented in Figure 4. In any case, a gain of energy by several keV is clearly indicated. Interestingly, the other initially separated streak did not exhibit any acceleration. This shows that auroral acceleration if restricted to narrow current sheets.
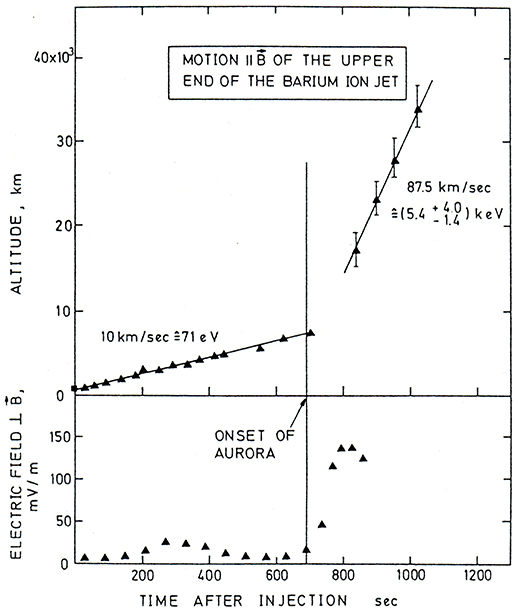
Figure 4. Upward propagation of the tip of a shaped-charge injected barium plasma jet above Greenland. The vertical line marks the appearance of an auroral arc in the foot area of the jet. The height determinations after the break were obtained by fitting the jet images from Thule with projected field lines and are somewhat uncertain (Haerendel et al., 1976).
Heppner et al. (1981) found another way for barium clouds to reach high altitudes. They were generated from a satellite orbiting at 965 km altitude. By the diamagnetic force, −μ∇B, the orbital momentum imparted to the ions was converted into parallel momentum in the upward diverging magnetic field. From the analysis of the field-aligned motions minor accelerations and decelerations were derived, but in one case substantial gain of parallel energy by 6 keV appeared to have occurred at heights above 15,800 km. The specific virtue of the releases from an orbiting spacecraft was that, contrary to shaped charge injections, they allowed measurements of very weak E|| fields and separation of spatial and temporal variations.
An intimately related result pertaining to the existence of field-parallel potential drops was the splitting of a streak at altitudes above 7,000 km by Wescott et al. (1976). It will be discussed in the next section in the context of coupling to the ionosphere. Taken together the few successful manifestations of field-parallel potential drops above auroral arcs can only be regarded as a proof of principle. No further insights into the acceleration process as such could be extracted. These were later on obtained from electric field and particle diagnostics from low-orbiting satellites, most importantly from the FAST mission (Carlson et al., 1998). Indeed, practically all aspects of the experimentation with plasma clouds discussed in this section were soon after successfully explored and even routinely measured by in-situ particle and field measurements. All the same, the barium plasma cloud experiments had the charm of being first, simple, visible, producing trustworthy results, and to fascinate professional or accidental observers. Already in 1968 this was honored by an article in Scientific American (Haerendel and Lüst, 1968b).
Coupling to the Ionosphere
Distortions and Striations
Barium plasma clouds are fascinating objects because of the visible manifestation of a variety of intriguing physical processes. A particularly striking aspect of experiments in the auroral ionosphere is the often observed strong distortions of the clouds. Figure 5A shows clouds over Ft. Churchill in 1967, one just being born out of the neutral barium gas, the other one generated 3 min earlier already extended transverse to the field by more than 200 km. The distortions of the gross shapes and the ubiquitous appearance of striations along the magnetic field direction, as shown in Figure 5B, have triggered much theoretical interest. Both experiments were performed in the auroral ionosphere where the electric fields are usually high. Distortions and striations result from interactions with the lower ionosphere, where the transverse electrical conductivity maximizes. As sketched in Figure 1, current exchange between cloud level and E-region by field-aligned electron currents can short-circuit or at least reduce electric polarization fields caused by conductivity enhancements by the Ba+ ions. This has the consequence of changing the conductivity in the E-region due to the divergences of the transverse Pedersen current at the interfaces with the field-aligned currents. The thereby generated secondary polarization fields act back on the on the motion of the barium ions. The artificial plasma clouds have mostly been injected at heights above 250 km. At these levels the ions are dominantly subject to E × B drifts. Any gross modifications of E⊥ are therefore translated into shear flows, i.e., into distortions.
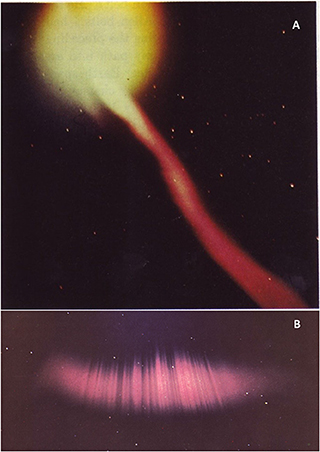
Figure 5. (A) Two barium clouds injected above Ft. Churchill/Canada with 3 min separation in August 1967. The yellow/blue colors indicate neutral Ba, the purple color the first injected cloud, strongly extended over 200 km horizontally. (B) Highly striated and extended barium cloud, also over Ft Churchill (Haerendel and Lüst, 1968b).
Equally striking are smaller-scale perturbations growing into visible striations. In the rear of a moving cloud, density (i.e., conductivity) enhancements are slowed down in comparison with the undisturbed motion because of the reduction of E⊥. They lag behind and become more pronounced. Density depletions, on the other hand, experience a higher electric fields and can advance faster into the cloud. Thus, the rear side of a drifting cloud is unstable with respect to small-scale perturbations. The opposite holds for the front side.
What has just been described has soon been recognized by Linson and Workman (1970) as leading to a cross-field or gradient drift instability. The non-linearity of the cloud-ionosphere interactions asked for treatment by numerical simulations. The stage was set by the two companion papers of Perkins et al. (1973) and Zabusky et al. (1973) and independently by Lloyd and Haerendel (1973). Indeed observed bifurcations (Figure 6) or splitting into multiple structures on the rear side of the distorted cloud were successfully reproduced by McDonald et al. (1981). In that paper the further question is pursued why structures of about 1 km width appear to be “freezing up,” i.e., persist for long times, while the gradient drift instability should lead to splitting into smaller-scale structures. Rocket flights through barium clouds measuring the electron density indeed verified the existence of large-amplitude perturbations with scales well below 1 km matching a power law distribution with a spectral index between −2 and −3 (Baker and Ulwick, 1978). McDonald et al. (1981) attributed the stabilization of km-scales to the presence of a hypothetical turbulent diffusivity which, depending on strength, could stabilize km-scale structures.
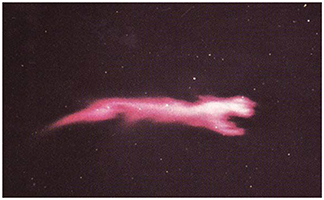
Figure 6. Barium cloud over Kiruna (1968) viewed along the magnetic field (Haerendel, 1996).
An analytical approach to the striation problem was chosen by Völk and Haerendel (1971). They took into account the feedback on density perturbations developing in the cloud from the density changes arising in the background plasma from the short-circuiting Pedersen currents. Because of a phase shift of the polarization field by 90 degrees between the density variations in the cloud and those in the background, the image striations in the background are tilted with respect to B. Furthermore, the finite parallel conductivity was included in the stability analysis. This has the consequence that the effective integrated Pedersen conductivity is reduced below a certain transverse scale and with it the depolarization effect. As a result the growth rate of a density perturbation or striation decreases strongly below a certain thickness.
In summary we have two competing explanations of the “freezing-up” of scales above about one km, turbulent diffusivity or reduced depolarization. The next sub-section introduces another but related aspect.
Magnetosphere-Ionosphere Coupling
With few exceptions barium cloud experiments were performed in or, by the shaped-charge technique, out of the ionosphere. A barium cloud injected from a Scout rocket at a geocentric distance of 6 RE in 1971 presented a new aspect, a prolonged phase of acceleration toward the flow speed of the ambient magnetospheric plasma. Figure 7 shows the development of the cloud during 1 h of observation. A few minutes after injection the cloud was seen to split into several magnetic field-aligned structures. While the brightest streak, the maximum-density core, followed closely the rocket trajectory, the other streaks with gradually lower brightness (density) separated with increasingly higher speed. In the first report on that experiment at a COSPAR conference (Haerendel, 1973) only preliminary results were presented with scarce speculative interpretations. Coupling to the ambient plasma and steady momentum deposition in the ionosphere was recognized as the most intriguing aspect raised by the experiment, but no detailed analysis was attempted. The greatest surprise was the apparent absence of oscillatory motions with a period of the bounce time of an Alfvén wave between cloud and ionosphere as predicted by the seminal theory of Scholer (1970). The rather different speeds assumed by the structures were (falsely) related to local gradients and variable ambient flow speeds.
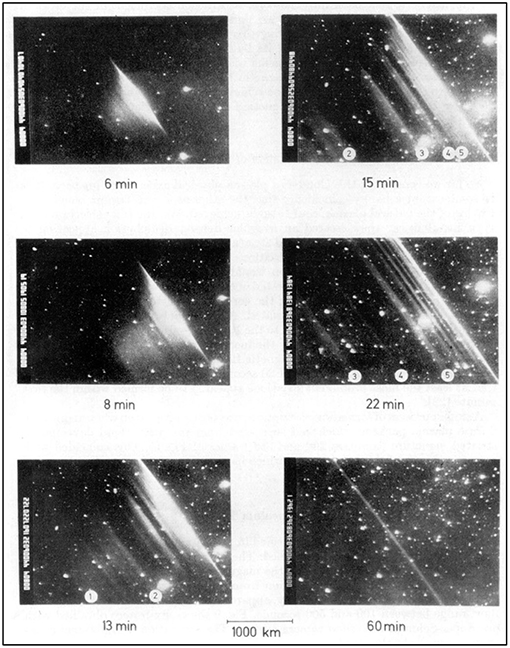
Figure 7. Six frames of the evolution of a barium cloud injected into the magnetosphere at 31 600 km altitude in 1971. The different relative speeds of the structures are inversely related to their mass loading density (Haerendel, 1973; Haerendel and Mende, 2012).
The other striking observation, made by the onboard search coil magnetometer, was the formation of a diamagnetic core. Entry into the returning field after one half minute indicated a width of the boundary much thinner than the gyroradius of a Ba ion. The true nature of the magnetic field return transpired much later at the occasion of the artificial comet experiments (s. section Interaction With the Solar Wind).
In retrospect it is hardly understandable that the evaluation of this most challenging experiment was left in this rudimentary stage. This can only be attributed to the lack of manpower and the pressure exerted by the close sequence of campaigns in various remote places, but not be excused. Publication in a refereed journal had to wait for 40 years. Finally, the treasures inherent in that experiment were finally unearthed.
Haerendel and Mende (2012) first reviewed the experimental parameters of cloud and environment and, following (Scholer, 1970) determined the magnitude of the theoretical coupling time constant to the environments:
With ρc being the mass density in the respective streak of the cloud, ℓ|| the effective length of the streak, vAthe Alfvén speed, and B the ambient magnetic field strength. It turned out to be almost 4 orders of magnitude larger than the bounce period of an Alfvén wave. With the high reflectivity of the ionosphere, little momentum would be transferred to the ionosphere but instead stored in increases of the magnetic shear stresses. Application of Scholer's theory predicted that the relative velocity of the cloud should have decreased by an order of magnitude after about 15 bounce periods (5 min) or even been reversed.
None of this was observed, but rather constant accelerations of the streaks, the greater, the lower the respective density/brightness. This meant that, after a short initial short interaction with the ionosphere by exchanging Alfvén waves, each streak had found its coupling constant in accordance with its respective mass content. How was that achieved? After a few reflections, observationally not resolvable, the streaks had assumed widths with scales below 1 km, when mapped into the ionosphere. Scholer (1970) had already suggested that owing to the finite parallel resistivity, σ||, the integrated Pedersen conductivity would have to be replaced by a substantially smaller effective Pedersen conductivity, ΣP, eff. The potential drop inside the ionosphere would not be controlled solely by the Pedersen conductivity, , but by a geometric mean conductivity, . This would reduce the reflectivity of Alfvén waves and thus lower the braking time.
Haerendel and Mende (2012) went one step further and postulated that scale breaking of the initial cloud was the reason for the formation of the striations. Perfect matching between wave impedance and effective conductivity, i.e., Rw ▪ ΣP, eff = 1, yields zero reflectivity. In this case the coupling constant is:
The principle behind scale-breaking is the optimization of the energy dumping into the ionosphere (see Haerendel, 2014). This energy resides in the magnetic tensions acquired by the initial reflections at the ionosphere. The consequence of perfect matching is that the transverse electric field of the striation is applied to the ionosphere and decays inside of it. The existence of a substantial potential drop above the ionosphere was discarded by the authors. Thus, the barium structures moved decoupled from the frame of the ambient plasma but were continuously losing momentum and kinetic energy with respect to the plasma frame until reaching the ambient flow speed. The leading diffuse streak in Figure 7 was indeed seen to acquire a velocity consistent with the external flow speed.
It is interesting to compare the here discussed coupling process with that of the ionospheric releases. The difference lies in the ratio of the time scales for momentum coupling with growth times of any deformation. In case of magnetospheric plasma injections, the ratio is large. The opposite holds for ionospheric clouds. Effective depolarization, as discussed in the preceding sub-section, means effective coupling. Formation of smaller-scale striations is enabled by decoupling due to reduction of the effective Pedersen conductivity thus allowing instabilities and relative motions. An interesting intermediate case was the Cameo releases from an orbiting spacecraft at 965 km, already discussed in sub-section Field-Parallel Acceleration (Heppner et al., 1981). All releases formed sheets of series of field-aligned streaks, obviously owed to the above discussed scale-breaking process. It would be rewarding if one could re-evaluate the observed irregularities of the initial transverse motions in terms of a finite momentum coupling time.
It is interesting to compare the above described experiment with later releases at various heights in the magnetosphere by the Combined Release and Radiation Effects Satellite (CRRES) (Fuselier et al., 1994). Depending on geocentric distance (2.0 RE, 3.4 RE, 4.8 RE), i. e. on magnetic field strength and plasma density, the coupling times to the frame of the ambient medium varied from 25 s to 5 min. This was entirely attributed to the momentum coupling to the ambient plasma. Only in one of the releases (G-3) there were indications of reflection at the ionosphere. A comprehensive overview of the CRRES experiments has been provided by Bernhardt (1992).
Decoupling by Potential Drops in the Magnetosphere
Decoupling from the ionosphere by processes within the magnetosphere can occur basically in two ways, by field-parallel potential drops in field-aligned current sheets or within kinetic Alfvén waves, owing to a finite E|| component. In the first case, the macro-physical reason is the mirror effect on the low-density energetic electrons carrying the current. The micro-physical reasons for sustaining the parallel electric fields are smaller-scale turbulence and, in particular, the action of solitary waves. While quasi-stationary auroral current sheets have typically widths of the order of 10 km, propagating kinetic Alfvén waves have considerably smaller transverse scale comparable to the electron gyroradius. What would we expect from barium plasmas injected into such acceleration region? Two effects, upward acceleration of the positive ions accompanied by decreasing brightness and decoupling from magnetospheric convection as indicated by accompanying lower-altitude clouds. The ion jet experiment from Greenland, discussed in sub-section Field-Parallel Acceleration, showed upward acceleration, but the decoupling, although very likely, could not be confirmed because of the lack of proper triangulation of the upper streak.
An experiment by Wescott et al. (1976) exhibited a reversed behavior. Clear decoupling was seen but very little upward acceleration. Barium ion jets were injected by rocket flights from Alaska into a diffuse aurora in the dawn sector. Figure 8 shows the development of the streak. At 15 min after injection a brightening of almost a factor of 3 was observed above an altitude of 5,500 km accompanied by a splitting into two secondary rays above that altitude. This has been interpreted as a decoupling by a parallel potential drop. The origin of the brightening was attributed to a Doppler shift out of the minimum of the Ba+ absorption line at 4,554 Å, caused by a sudden increase of the combined upward and transverse ion velocities away from the sun. Quantitative checks supported this explanation. Only relatively low velocity increases were needed. And curiously, evaluation of the upward motions suggested an energy gain of only 34 eV, well below what is typical for auroral arcs.
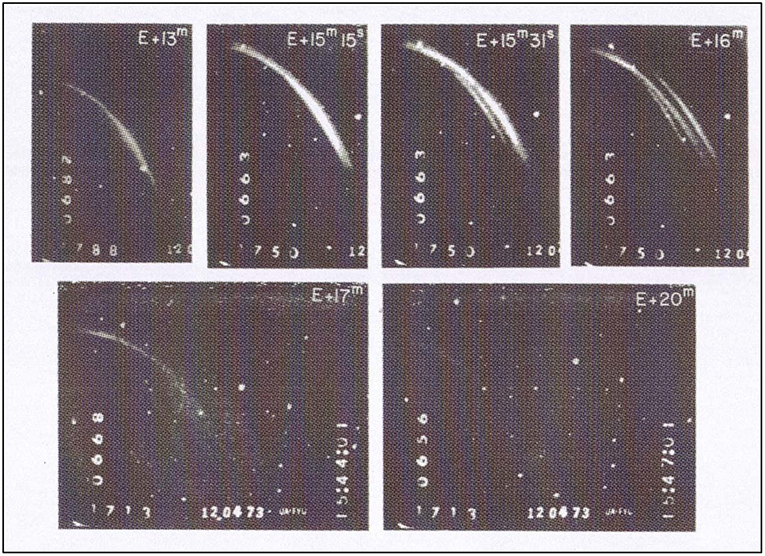
Figure 8. Plasma jet injected from Alaska showing anomalous brightening after 15 min and 15 s above an altitude of 5,500 km accompanied by splitting into two secondary streaks, which are decoupled from the lower sections of the respective flux tubes. Non-parallelism of the streaks and deviations from model field indicate nearby upward sheet currents (Reproduced from Wescott et al., 1976 with permission of John Wiley and Sons).
The reason for the absence of any strong upward acceleration observed probably lied in the auroral situation. The barium streak was determined to nearly coincide with the poleward edge of an omega band. At this boundary exists a strong flow shear from mildly eastward inside the visible bands to a rapid eastward speed of up to 4 km/s (Buchert et al., 1988). Furthermore, from fitting the high-altitude main streak with magnetic field models, Wescott et al. (1976) derived the existence of an upward sheet current of 0.2 A/m. This is in good agreement with the nature of omega bands which are caused by precipitation of typically 10 keV magnetospheric electrons carrying rather weak parallel currents of order 1 μA/m2distributed over 100 km north-south or more (Buchert et al., 1990). This means that for sustaining the current no post-acceleration of the electrons is needed. There is a dilemma. The observed splitting of flux tubes filled with barium plasma above 5,000 to 6,000 km is not disputable and no other obvious reason for that than electrostatic decoupling comes to mind. On the other hand, the geophysical nature of the local aurora does not require parallel potential drops, nor do the Ba+ ions indicate their existence except for a few tens of volt. Can it be that there are indeed weak potential drops existent at the interface between the dilute hot magnetosphere and the cooler and denser topside ionosphere? The one-dimensional model of electric fields in upward field-aligned currents by Ergun (2002) showed exactly that possibility. At the time of writing of this review, this appears to be the best explanation for the discussed observations.
The here presented evidence for magnetospheric decoupling regarded together with the prior discussion of coupling and decoupling, including the evidence for field-parallel acceleration in Sub-section Field-Parallel Acceleration, represent examples of the unique contributions of the plasma cloud experiments to the exploration of the fascinating subject of magnetosphere-ionosphere coupling.
Interaction With the Solar Wind
The proposal of Biermann et al. (1961) to generate plasma conditions in the solar wind simulating its interaction with comets, was the first goal of German space research. It took a quarter century, space around Earth was already widely explored, before this goal was fulfilled. Only two such “artificial comet” experiments were performed within the AMPTE mission (Krimigis et al., 1982). However, the presence of extended in-situ diagnostics in support of the optical tracing from ground and aircraft led to a rich harvest of new physical insights. AMPTE was standing for Active Magnetospheric Particle Tracer Explorers and consisted of three spacecraft from the USA, Germany, and the United Kingdom. The name was derived from the other goal of the mission, namely to investigate the mass transfer efficiency from solar wind and magnetotail into the inner magnetosphere by injecting primarily Lithium ions form the outer spacecraft, the Ion Release module (IRM), and searching for them with the Charge Composition Explorer (CCE) inside the magnetosphere. Unfortunately, this part of the mission was not successful (Krimigis et al., 1986). Here we will only summarize the physical processes taking place at the location of the injected plasma.
Pick-Up Ions
The contribution of interstellar helium to the ionic constituents of the solar wind by photo-ionization of the neutral atoms near the sun had been a subject of investigation long before the actual pick-up of freshly ionized lithium ions was recorded by the AMPTE/IRM spacecraft. A first Li-cloud was injected into the solar wind ahead of the bow shock for the tracing purposes mentioned above in September 1984. Being ionized with a time scale of 1 h, Li+ ions were continuously generated in the freely expanding neutral cloud and became immediately subject to the force of local electric field. Initially they just followed the electric vector, but when born at larger distance from the spacecraft and thus sufficiently accelerated, the Lorentz force took over. As seen by the plasma analyzer (Paschmann et al., 1986) and the Supra-thermal Energy Ionic Charge Analyzer (SULEICA) (Möbius et al., 1986), the energy of the ions grew with time and the arrival direction changed. Only weeks after the latter instrument registered for the first time interstellar He-ions in situ (Möbius et al., 1985). This was the beginning of a long success story of interstellar pick-up ions and their role in the solar wind (Möbius et al., 1988). The AMPTE mission was first in actually observing the very pick-up process.
Magnetic Cavities
A genuine plasma physical effect of the Li+ injections was the formation of magnetic cavities. Even with the long ionization time of lithium and the more so with the fast ionization of barium, the freshly generated plasma caused a strong inflation and depression of the magnetic field, lasting until the momentum of the plasma cloud equaled the external magnetic pressure. This was already seen in the Scout experiment in the magnetosphere (section Magnetosphere-Ionosphere Coupling). The altogether eight AMPTE releases offered a valuable sample of that effect allowing deeper investigation of the ongoing physical processes. The most important and hitherto unknown ones were the unexpectedly fast recovery of the cavity, the mass pile-up behind the front of the returning field, and the thinness and longitudinal structure of the magnetic boundary.
Figure 9A documents the first two effects as recorded during the first artificial comet experiment upstream of the bow shock on 27 December 1984 (Gurnett et al., 1986). Immediately after ignition of the Ba charges the magnetic field strength dropped below the level of sensitivity. After 80 s, a sharp return of the field with greatly amplified amplitude was accompanied by an increase of density by a factor of about 5. From estimates of time and radius of maximum expansion one could determine that the speed of the returning front of the field was 4.5 km/s. Haerendel et al. (1986) interpreted what had happened as consequence of a snow plow effect. The returning field sweeps up the yet un-magnetized Ba plasma which piles up behind the front. The high mass load slows down the speed of the returning field to the thus modified Alfvén speed. Combining magnetic gradient and curvature forces yielded a value of a Alfvén speed consistent with the derived speed of the snow plow. In the second comet experiment of July 1985 and even better in a later experiment in the magnetotail the snow plow propagation could be clearly observed.
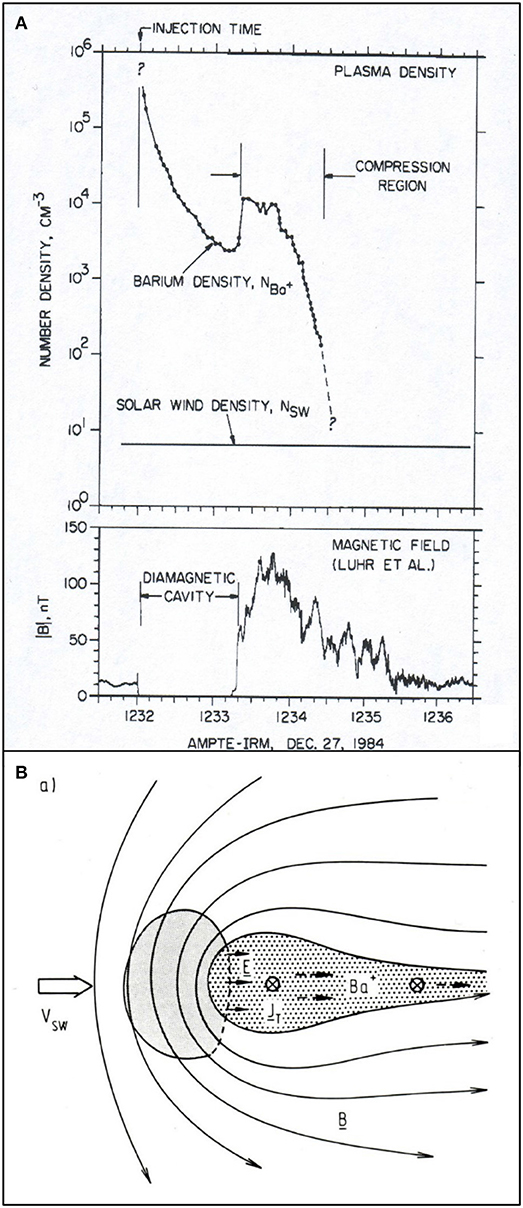
Figure 9. (A) Top panel: Electron number density obtained from the observed plasma oscillations. Bottom panel: Total magnetic field strength exhibiting a magnetic cavity for 80 s. Owing to the action of the solar wind, the magnetic field is strongly compressed and penetrates into the cavity thereby sweeping up the barium plasma like a snow plow (Gurnett et al., 1986). (B) Cartoon showing the magnetic normal and shear forces acting on the cloud. The momentum is transferred through the cloud and is applied to injecting ions from the rear end into the tail (Haerendel et al., 1986).
The magnetic field compression from 10 to 130 nT was attributed to the action of the solar wind. As sketched in Figure 9B, magnetic normal and shear stresses contribute about equally to the force acting on the plasma cloud. This leads to the compression factor (Haerendel et al., 1986; Haerendel, 1987):
With MA⊥≈ 6 the relation can explain the observed amplification.
The returning magnetic field offered two surprises, the short duration of the entry of only 0.5 s and the low amplitude of the electric noise appearing below the electron gyrofrequency (Gurnett et al., 1986). Expected was a high noise level and broader width indicating the presence of some kind of anomalous magnetic diffusivity. The short duration of the electric noise and its low amplitude suggest the existence of an electron-scale sub-layer, through which the electrons are scattered into the snow plow front, which is substantially wider. This became clearer with a barium injection in the tail in the following year. In that experiment, the magnetic cavity and the propagating snow plow were observed more or less along the magnetic field and well resolved over many minutes. Most remarkable were the field-aligned ripples covering the inner surface of the already magnetized plasma (Figure 10a) (Bernhardt et al., 1987). This modulation was subsequently interpreted by the author in a paper dedicated to Ludwig Biermann (in German) as owed to the different ways ions and electrons enter the magnetic field (Haerendel, 1986). As sketched in Figure 10b, the boundary is so thin that the ions are hardly deflected by the magnetic field but stopped by an opposing polarization field. The electrons are scattered into the boundary layer and, being magnetized, carry a Hall current shielding the magnetic field. Balancing it with the current carried by the entering ions, a width of the order of c/ωpi was derived (Szegö et al., 2000, p. 617). The observed ripples had widths of about 300 km, whereas the ion inertial radius of the ions was about twice as large. By contrast, Bernhardt et al. (1987) attributed the ripples to an interchange instability, but obtained scales at least one order of magnitude too low. There have been other interpretations (see below).
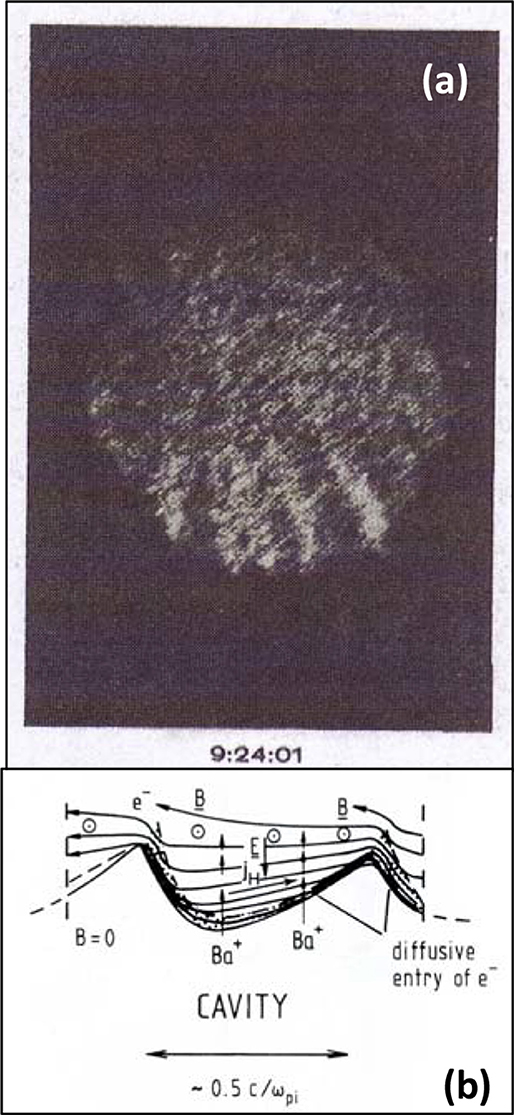
Figure 10. (a) Ripples on a barium plasma cloud released in the Earth's magnetotail (Bernhardt et al., 1987). (b) Proposed formation of the ripples as caused by the ions penetrating directly into the field with the electrons following by diffusive entry. The electric field, E, stopping the ions drives a Hall current which is shielding the magnetic field. Balancing the energy entered by the ions with the energy carried away along the magnetic field (normal to the plane) enforces structuring of order c/ωpi (Szegö et al., 2000).
Many years later the author noticed the similarity between barium ions entering the returning magnetic field and a situation encountered in the central plasma sheet during the onset of substorms (Haerendel, 2015). He postulated the formation of a sharp boundary of width, c/ωpi, where ions in the high-beta central current sheet are being slowed down without being reflected. The question, not asked in the barium experiment, is: Where do the energy and momentum go that are deposited by the ions? The answer came from the reverse side by wondering about the source of the energy of the highly structured thin arcs observed at substorm onset. The short-lived erratic bead structure was attributed to the impact of kinetic Alfvén waves launched from corresponding ripples of the Hall current in what was called the “stop layer.” In these ripples the kinetic energy of the ions is converted into electromagnetic energy and carried away by the waves. Since their amplitude is limited by the strength of the stopping magnetic field and Hall current, energy and momentum can only be deposited within a limited lateral scale of the order of c/ωpi. While the stop layer in the equatorial magnetosphere at typically 8 RE is still a conjecture and the ripples have not yet been observed, the barium experiment demonstrates their existence in a not dissimilar situation.
Magnetic cavities were also studied with releases of the CRRES mission (Huba et al., 1992). The emphasis was mainly directed toward comparison with numerical simulations by MHD and non-ideal Hall MHD codes. Structures of scales size 1–2 km formed within 3 s for the release at 4.8 RE and with scale sizes of 10–15 km within 22 s for the release at 6.2 RE. They are attributed to the collisionless Rayleigh-Taylor instability. This differs decisively from the stop layer mechanism discussed above. Equally different is the reason for the density increase after the maximum expansion time in these simulations. It is a pile-up resulting from sequential deceleration, first of the fastest ions and followed by the slower ones which are catching up. Owing to the use of MHD a snow plow effect does not exist. Unfortunately, there is no accompanying documentation of the actual optical observations.
Ion Extraction and Momentum Balance
Among the many surprises of the AMPTE barium releases in the solar wind the perhaps most perplexing one at first sight was the dynamic behavior of the comet head. During the first 4 min, the center of mass was not at all displaced in the direction of the oncoming solar wind but at right angles (Valenzuela et al., 1986). The explanation was given by Haerendel et al. (1986) and in more detail in Haerendel (1987). In the frame of the barium cloud (comet head), there is an electric field, E⊥ = −vsw × B, which is felt by the ions in the boundary layer of the cloud. Ions are being extracted and enter a cycloidal path much wider than the cloud dimension. Momentum balance, i.e., the recoil of the extracting force, pushes the bulk plasma sideways, opposite to E⊥. The long station-keeping of the cloud in s.w. direction is a consequence of the generation of a tail. The normal momentum imparted by the solar wind is transported through the comet head and delivered to its rear. From there ions are extracted, again by an electric force, and injected downstream. A cloud image from the first artificial comet experiment and an explanatory sketch are contained in Figures 11a,b.
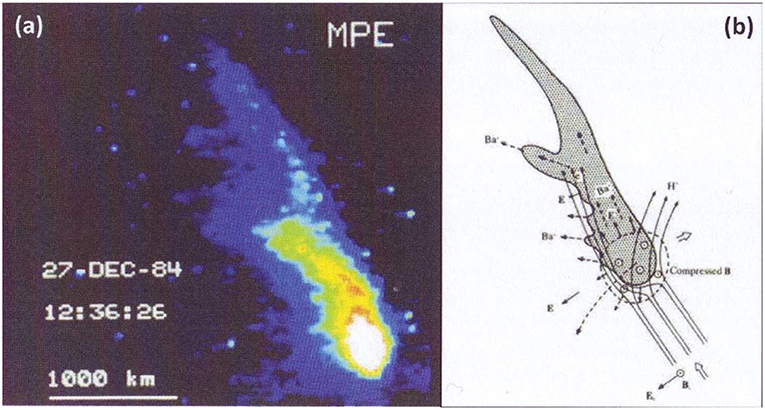
Figure 11. (a) False color image of the first artificial (plasma) comet 4.4 min after release in December 1984 exhibiting ion extraction (to the left) and tail formation (Valenzuela et al., 1986). (b) Interpretation of the cause of the lateral displacement of the comet head (open arrow) as reaction to the extraction of ions by the solar wind electric field, E. Solar wind protons, H+, are only slightly deflected by the trapped magnetic field, whereas the magnetized electrons perform an ExB-drift along the opposite flank. The associated Hall current shields the field on one side (Haerendel et al., 1986).
Simple analytical calculations to be found in Haerendel (1987) and Szegö et al. (2000) lead to the following simple relations. The accelerating force experienced by the cloud via the draped and compressed magnetic field is:
Ac is the cross-section of the cloud, and Mc the total mass. In the factor 4 are contained the momentum imparted by the Alfvén wings of the draped magnetic field and the compression of the field (s. Equation 5). The transverse acceleration was determined to be:
The latter actually matches the observations of the lateral displacement (Valenzuela et al., 1986). Being applied to the tail formation, g|| could not be directly observed (s. below).
Figure 11b shows the flow lines of the electrons (left) and of the sw protons (right). The electrons are magnetized and follow the E × B drift, while the ions experience only mild deflections by the strong internal magnetic field. Both flows constitute Hall currents corresponding to the field concentration in the comet head. The magnetic field is asymmetrically distributed in the cloud owing to the different widths of the electron and ion Hall currents. It is the negative magnetic pressure gradient existing through much of the cloud what transfers the recoil of the ion extraction pushing the comet head sideways.
Because of the lateral deflection, the AMPTE/IRM exits the cloud in the direction of the ion extraction. When it had reached the low-density flank, suddenly (at 1234:23 UT) a very strong electrostatic noise appeared (Gurnett et al., 1986). The latter author interpreted the rather unstructured electrostatic noise as generated by an ion beam-plasma instability between the nearly stationary barium ions and the rapidly moving solar wind protons. In the frame of the E × B drifting electrons, the Ba-ions moving along the E-vector are practically at rest. Papadopoulos et al. (1987), expanded the theory along the same lines and argued that the noise was saturating by proton trapping in the electric wave field. From the spectral energy density in the lower hybrid region he determined an anomalous ion-ion collision frequency. This implies a dynamic momentum coupling from the protons to the barium ions in addition to the laminar momentum coupling by the extracting quasi-static electric field.
Tail Formation
As argued above, the station keeping of the cloud in the sw direction was owed to the momentum imparted by the draped magnetic field and transported through the cloud to its rear end. Like in the popular toy of several suspended balls in contact, it is the last mass that carries away the momentum. But how is that achieved in the Ba+ cloud? A simple argument is presented in Szegö et al. (2000). In most of the cloud the upstream directed normal stress (magnetic and pressure) is balanced by the downstream pointing tangential stress of the field. The bulk plasma is not accelerated. Toward the rear, the relation changes. Both forces point downstream, only balanced by the inertial force of the accelerated ions. The acceleration completed, the density of the ion outflow must equal approximately that of the solar wind, since the ions are neutralized by the solar wind electrons entering the forming tail from the side and not by the photo-electrons (Haerendel, 1987). With that assumption the speed attained by the ions is (Szegö et al., 2000):
μiis the atomic weight of the ion. For Ba+ this amounts to 24 % of the sw speed. Photometry of the plasma cloud was not really possible. However, the pictures obtained by the Doppler imaging system of Rees et al. (1986) show clearly the strong drop in density to be expected from the above considerations.
Computer Modeling
Naturally, the above presented simple analytical derivations are very simplistic, just trying to catch the essential physics. Reality is more complex. Fortunately the theoretical plasma physics community was fascinated by such plasma experiments not impeded by walls and analyzed on the microphysical scale by in-situ diagnostics. Many efforts have been made to study the physics by numerical simulations.
Cheng (1987) studied the lateral deflection of the cloud and emphasized the role of the deflection of the solar wind protons. It generates an electric polarization field driving a Hall current on the side opposite to the solar wind electric field. This maintains the compressed magnetic field and its normal stresses contribute to the sideways motion.
Brecht and Thomas (1987) performed a three-dimensional simulation with a hybrid code in which the electrons were considered as a massless fluid. They studied in particular the formation of magnetic cavities and the magnetic field compression within the snow plow region. They could also reproduce the cross-field deflection of the cloud.
Huba et al. (1987) applied theory and simulation of the Rayleigh-Taylor instability to the appearance of striations in the tail release, discussed and interpreted above. They see the driver in the slowdown of the initially expanding cloud by the outer magnetic field. In the large Larmor radius limit, the fastest growth rates were found at the shortest wavelengths. This is in agreement with the analysis of Bernhardt et al. (1987) (see above), but not consistent with the observation. However, the simulations also showed the longer wavelengths to dominate the forming structures.
Bingham et al. (1988) simulated the initial magnetic field compression around the barium cloud and addressed in addition the observed heating of the electrons. Calculating the beam-plasma instability and the growth rate of lower-hybrid waves they found substantial electron heating by Landau damping along the magnetic field. However, the observed electron spectra, as measured by the United Kingdom Sub-satellite (UKS) only a bit outside the cavity (Bryant et al., 1985), were more alike to an acceleration by a quasi-static electric potential drop.
An interesting approach was the bi-ion fluid simulations of Sauer et al. (1994) and Bogdanov et al. (1996). Light and heavy ions are considered as fluids and the massless electrons follow from charge neutrality and Ampere's law. Christian Fischer in Szegö et al. (2000) extended the bi-ion fluid simulations to three dimensions and computed the case of a plasma comet. In Figure 12 one can clearly recognize the different paths taken by the heavy ions and the solar wind protons. Furthermore, it reproduces the observation, more striking with the second experiment in 1985, that the tail flows are not laminar but form clumps or knots. The author had suggested a clumping instability for their formation (Szegö et al., 2000). The simulations confirm the underlying mechanism that the heavy ions move in and out of the knots and are thus on average faster than the knots are proceeding. This has implications on so-called disconnections in comet tails.
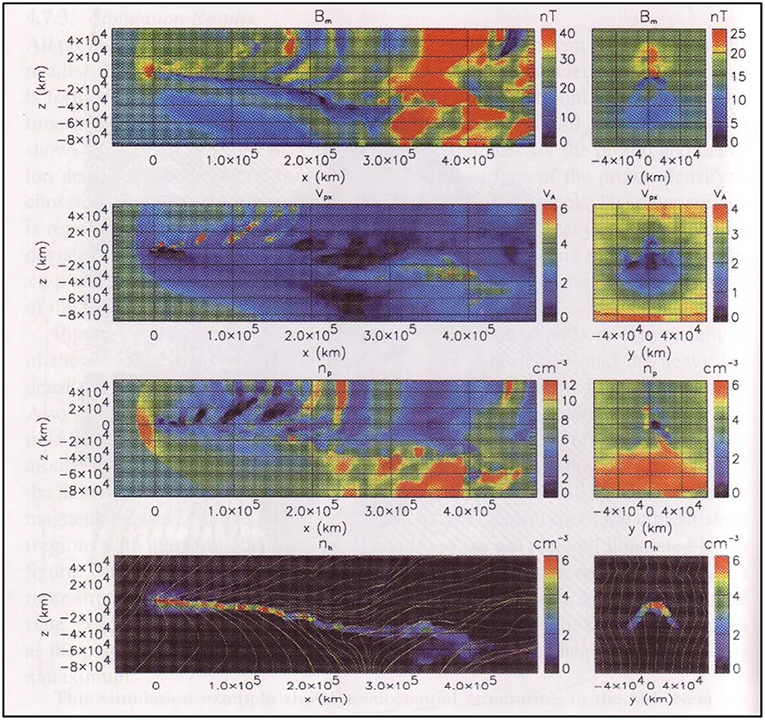
Figure 12. 3D bi-ion fluid simulation box at t = 200 of magnetic field, Bm, proton velocity, vpx, proton density, np, and heavy ion density, nh, for a 5,000 km large mass loading event in the solar wind. Left: xz-cut, right: yz-cut. Field lines in the bottom xz-cut are projections of the 3D field through the tail (Christian Fischer in Szegö et al., 2000).
In summary of this incomplete account of the various processes observed in the two artificial comet experiments, one can say that they led to the recognition that fundamental transport processes are often enabled just by the different inertia of ions and electrons without much accompanying noise.
Modification Experiments
Inospheric Modifications
There are many ways of injecting neutral gases into the upper atmosphere, both intentionally and as by-products of rocket exhaust. Once released, some gases, like barium, strontium, europium are photo-ionized or, in special cases, can be ionized by collisions. Others undergo chemical reactions by which the natural plasma component is reduced by recombination processes, thus creating plasma holes rather than clouds (Mendillo, 1988). The latter techniques and applications have been reviewed by Bernhardt et al. (2012). Here we summarize the attempts to modify the ionosphere for stimulating, triggering, or at least tracing natural ionospheric instabilities. The formation of striations in barium clouds discussed in section Coupling to the Ionosphere is an example.
A most ambitious goal was the attempt to trigger equatorial spread-F. This phenomenon has attracted much attention. It is an ionospheric realization of the Rayleigh-Taylor instability after sunset when the gradient of the denser F-region has risen up to and above 350 km. A wide range of secondary processes follows (Hudson and Kennel, 1975; Zalesak et al., 1982). It has been explored primarily by radar measurements, optical observations, and by in-situ probing with sounding rockets (Balsley et al., 1972; Woodman and La Hoz, 1976; Kelley et al., 1982; Kelley, 1989). MPE conducted four rocket campaigns under spread-F conditions, initially for tracing the evolution of the irregularities and finally for triggering an instability. The first three attempts took place in Thumba/India in 1972, in Natal/Brazil in 1973, and in Punta Lobos/Peru in 1979. Either the rockets failed or spread-F did not develop, as long as the clouds were observable. The only result was the appearance of the ubiquitous striations and distortions. However, in the combined campaigns of BIME by Narcisi (1983) and Colored Bubbles by Haerendel et al. (1983) in Natal in 1982, there was partial success. Later theoretical considerations made clear that charges with 26 kg of the Ba-CuO mixture as employed in these experiments would not suffice to generate sufficiently heavy plasma clouds capable of massively disturbing the ionosphere. Much higher masses would be needed. However, they sufficed to excite localized spread-F.
The basic idea of the Colored Bubbles experiments was to place two big barium clouds side by side, on upleg and downleg of a rocket flight, respectively. It was to be expected, that while the two flux tubes heavily loaded with barium plasma would move downward, the flux tube in between would rise. This is owed to the incompressibility of the magnetic field. The hope was that eventually the rising flux tube would evolve into a bubble, the name introduced for a low-density plasma rising into the dense upper F-region. Such bubbles, specifically their westward borders, have been recognized as the main sites of secondary instabilities due to the eastward blowing neutral wind (Zalesak et al., 1982). However, numerical simulations of the experimental situation by Çakir et al. (1992) later showed that the creation of a real bubble would require 40 times higher masses to be injected.
Besides the two big barium releases near apogee, there were five small releases on the upleg, in order to trace the vertical shear of the plasma flows, and a light europium release for tracing the expected plasma uplift between the big clouds. Above about 250 km the flows were directed eastward. This meant that the barium clouds disappeared in the shadow after about 20 min. This precluded optical tracing of the longer-term development. For this reason the two campaigns had secured the cooperation of several groups with competence in radio wave tracing by ionosondes, radar, or recording scintillations of radio beacons from geostationary satellites. The latter was performed by Johnson and Hocutt (1984) on a NASA aircraft. Following the eastward motion of the clouds, they were able to track scintillations with steadily growing amplitudes consistent with the continued eastward drift of a region related to the two big clouds. The best matching localization was the space between them. Figure 13 shows the measurements during the second experiment. After 40 min the amplitudes were of a size as found in any natural spread-F. In conclusion, a gross ionospheric instability arising from the non-linear development of a bubble did not occur, but equatorial spread-F was for the first time artificially stimulated.
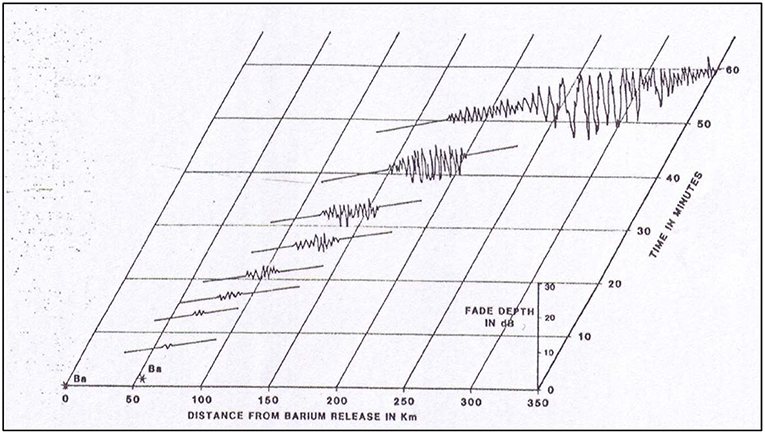
Figure 13. Scintillation amplitudes of a radio beacon signal through a region disturbed by two injected barium clouds at 350 km height propagating eastward (Reproduced from Johnson and Hocutt, 1984 with permission of John Wiley and Sons).
There was another outcome of the Colored Bubbles campaign. It was derived from the observed motions of the five small releases on upleg, which exhibited a strong vertical variation of the horizontal plasma flows. In an attempt to understand the reasons for the shear flows, an analytical model using flux tube integrated quantities was developed and the temporal evolution of the post-sunset equatorial ionosphere computed. Thereby it was possible to analyze the roles of the three most important contributors to shear flow and post-sunset rise of the F-region, the neutral wind dynamo, the Hall current, and the divergence of the equatorial electrojet during sunset (Haerendel et al., 1992).
The author is not aware of any subsequent experiment with the same goal and employing plasma clouds. Attempts with generating ionospheric holes are being reviewed by Paul Bernhardt (this volume).
Magnetospheric Modifications
Subsequent to the classical paper by Kennel and Petschek (1966) on the electromagnetic interaction of energetic particles trapped in the Earth's magnetic field with ion cyclotron and whistler waves, a great many of publications appeared dealing with the possibility of modifying artificially the resonant energies by artificially changing the ambient density. The first suggestion came from Neil Brice (1970). Many detailed calculations followed showing that plasma enhancement could lower the resonant energies to an extent that the originally stable ions or electrons were destabilized leading to enhanced precipitations and measurable auroral light emissions (Cornwall, 1974; Cuperman and Landau, 1974). When the Max Planck Institute for extraterrestrial Physics was offered a free ride on the second Ariane test launch, project “Firewheel” was initiated. A mother spacecraft carrying 12 release containers and four instrumented sub-satellites were built in labs in the USA, Canada, the United Kingdom, and Germany under the leadership of MPE. Besides the goals described above (section Interaction With the Solar Wind) in the context of the later performed artificial comet experiments, a central objective was to realize a substantial plasma density enhancements by a lithium release at about 9.5 RE geocentric distance near midnight and observe in-situ the response of the wave activity and from ground the stimulated precipitation through enhanced light emissions. In the Li experiment, a volume of 500 km cross-section would receive a density enhancement of more than one order of magnitude, and the phase velocities of the cyclotron waves would be lowered by the same ratio. Not discussed was the possibility of a maser effect by trapping of the waves. To the great disappointment, not only of the participants but also of a widely interested plasma physics community, the Ariane L02 launch failed.
While the AMPTE mission did not allow for releases inside the magnetosphere, there appeared a new opportunity with the Combined Release and Radiation Effects Satellite of NASA which was launched in July 1990. Besides a diagnostic payload the spacecraft carried 24 chemical release containers for 14 different experiments. A larger number of research institutions and scientists joined the observational efforts and divided the responsibilities for each of the 6 types of investigations. Two releases of lithium at high altitudes conjugate to the auroral ionospheres were planned and performed. However, no positive results have been reported.
Proof that the predicted stimulated precipitation can work in principle is supported by a natural experiment, the so-called SubAuroral Morning Proton Spots (SAMPS) (Frey et al., 2004). Following a geomagnetic storm, the previously strongly eroded plasmasphere usually starts growing in size because of refilling with cool ionospheric plasma. Still existing fluxes of energetic protons from the ring current then drift into a new high-density environment lowering the threshold for ion-cyclotron resonances. This was the situation reported in the cited paper. With no diagnostics available testifying for the stimulated wave-particle interactions, only the appearance of isolate proton emissions in the morning sector had to be taken as evidence.
Auroral Stimulation Experiments
By contrast with the unsuccessful attempts to stimulate auroral particle precipitation in the magnetosphere, there was a rich and successful activity to do so in the auroral ionosphere. Probably the first encounter of electron precipitation stimulated by a plasma injections occurred during two barium experiments in Esrange/Kiruna. Observing auroral emissions from the E-region, Stoffregen (1970) found enhancements of the 5,577 and 4,278 Å lines by a few percent lasting for a few tens of sec. No other diagnostics being available this was only indirect evidence for stimulated electron precipitation.
Kelley et al. (1974) reported about perturbations connected with the barium injection process performed on five rocket flights in the auroral ionosphere dedicated to comparing different techniques of measuring electric fields. Seven types of perturbations were identified including changes in electron temperature and density, in the ac and dc electric fields, and in the electron flux. Triggering these effects had not been planned for. The configurations cloud-payload were therefore somewhat accidental. While the perturbations on the local plasma environment are to be expected, changes in the energetic electron fluxes could be indications of long-range effects. However, in the light of later experiments (see below) it was rather puzzling that in the only once observed enhancement of the electron flux, lasting for about 20 s, the pitch-angle distribution above 15 keV was shifted toward 90°. The authors suggested that Alfvén waves triggered by the explosion might have interacted resonantly with trapped electrons several 100 km above the rocket.
The first experiment expressly dedicated to triggering electron precipitations was performed by Holmgren et al. (1980). It was quite appropriately named “Trigger” and consisted of a mother-daughter payload. The cesium release from the daughter on the downleg occurred 1.5 km above the instrumented mother payload. Cesium was chosen in order to obtain a fast ion production from the explosion, which for obvious reasons was planned to be performed in darkness. Forty milliseconds after the explosion a strong electric signal appeared, reaching a peak of 200 mV/m after 140 ms, and subsiding 100 ms later. About coincident with the electric field maximum a sudden increase of electrons fluxes by several orders of magnitude was registered by the downgoing as well as by the upgoing channels, with no increases in the 90° pitch-angles (Figure 14). Most pronounced were the fluxes at 2 keV. The spectra obtained by Lundin and Holmgren (1980) are most revealing. The sharp fall-off above a few keV, together with the field-aligned concentration, means that there must have been electrostatic acceleration along B. Downgoing fluxes of up to 11 erg cm−2 s−1 corresponded to a current density of 1 μA/m2.
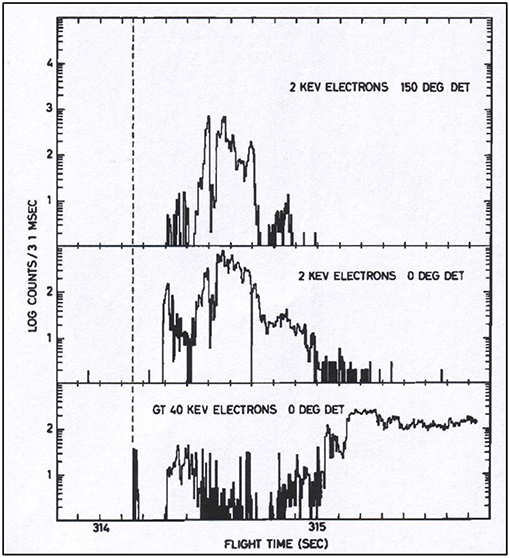
Figure 14. Burst of electrons following the injection of a cesium cloud at 164 km height at Esrange in 1977. Top panel: 2 keV electrons near 150° pitch angle; middle panel: near 0° pitch angle; bottom panel: 40 keV electrons near 0° (Reproduced from Holmgren et al., 1980 with permission of John Wiley and Sons).
Marklund et al. (1988) analyzed the Trigger results and concluded that the expanding neutral cloud swept up the ambient plasma creating a radial Hall current which was partially balanced by a reverse Pedersen current driven by the generated polarization field. The net current was closed by field-aligned currents in both directions. Thus, two Alfvén wave were launched. Estimating the Pedersen and wave conductances the authors concluded that the expanding neutral cloud, including a preceding shock wave, constituted a voltage generator. On the basis of the measured amplitude of the electric field and the dimensions of the cloud, field-aligned current densities well above those observed in auroral arcs can be derived, although with a high degree of uncertainty. Some of the authors therefore discussed the possibility of the current becoming unstable and developing anomalous resistivity somewhere in lower densities above the cloud (Kelley et al., 1980; Yau and Whalen, 1988). This way field-parallel voltages of 2 kV and above could have been generated. However, the above cited energy flux would be inconsistent with such a model and also with the presence of similar electron fluxes from below. Dissipation of the field-aligned current due to the finite parallel resistivity of the ionosphere and runaway electrons emerging therefrom, have not been considered. On the other hand, it is very unlikely that the field-parallel electron fluxes could have been generated by pitch-angle scattering of magnetospheric electrons. This hypothesis was therefore discarded by Holmgren et al. (1980). Equally difficult to explain is that next to the dominant electron component of 2 keV short enhancements were measured as well at energies up and above 40 keV and near 0° within a second after injection. The experiment left many open questions.
Next to the electron enhancements and the electric field pulses also electric field and density waves were observed, first overlapping with the electron burst and, after 1 s, for another interval of about 1 s (Kintner et al., 1980). The second pulse in the range of 1–2 kHz appeared when the Cs-cloud had surrounded the payload. The authors interpreted the waves as excited by an ion-ion streaming instability between Cs+ and the ambient ions.
Years later the Swedish group tried to repeat the Trigger experiment with two rocket flights, the Tor project, however with less success (Holmgren et al., 1988). With similar intentions Wescott et al. (1985) injected barium with shaped charges transverse to the magnetic field in sunlight for photo-ionization. Electric field pulses as well as waves were observed but no stimulated electron precipitation. However, promptly following a barium release, ions up to 6.8 keV with auroral intensity were observed, not energized, but presumably pitch-angle scattered by the explosion.
Another type of active experiments along the same lines were the plasma depletions caused by the exhaust of the Space Shuttle (Mendillo et al., 1975) and, intentionally, by releases of H2O, CO2, and N2 from explosive charges of nitromethane and ammonium nitrate in Project Waterhole (Yau et al., 1981; Whalen et al., 1985). The goal was to reduce the local plasma density by a factor of 10 and, in the presence of strong field-aligned currents, lower the threshold for current instabilities. The ensuing effects were to be observed in situ and by watching the auroral emissions along the affected flux tube. A release into a stable auroral arc led to large depressions in the local plasma density, in the precipitating electron flux, and in the emissions at 5,577 Å. This lasted for more than 2 min. In another rocket flight, the electron flux at 1.5 keV decreased slightly, but large enhancement were observed at small pitch angles between 0.1 and several keV. Furthermore, large transient electric and magnetic fields appeared with the passage of the shock front from the explosive release. The strong transverse perturbation field of 275 nT clearly indicated the generation of field-aligned currents. Yau and Whalen (1988) speculated that electrostatic ion cyclotron waves may have been excited by the current in the reduced plasma density of the hole.
The cited paper by Yau and Whalen (1988) contains an excellent review of all the here mentioned auroral modification experiments including the relevant associated literature. What stands out among the various attempts, is the Trigger experiment of 1977. The mechanism leading to enhanced electron fluxes, most likely accelerated out of the ionospheric plasma background, remains largely unexplained. At the time of writing of this review, active plasma experiments in space appear to be stories from the pioneering past. However, the open questions remain und would deserve new attempts for their clarification. However, one must acknowledge that, like in case of the magnetospheric modification experiments, it is not easy to initiate nature to give away its secrets by the modest means accessible to space researchers.
Special Physical Processes
Critical Ionization Velocity Experiments
Alfvén (1954) introduced the critical ionization velocity (CIV) effect in a theory of planetary formation. It is a beam-plasma discharge developing when a beam of neutral particles crosses a magnetized plasma. The energy is drawn from the kinetic energy of the neutrals transverse to the magnetic field in the plasma frame. The transverse velocity component must exceed a critical threshold, vcrit:
(mn is the mass of the neutral particle). The idea is that instabilities of the freshly generated ions excite plasma waves (e.g., lower hybrid), by which the electrons are resonantly heated up to and beyond the ionization potential, Vion, of the neutrals. If the electrons make at least one ionizing collision during the time of contact with the neutrals the discharge can proceed. The latter is the so-called Townsend condition. It implies a lower limit on the density of the neutrals.
Alfvén's idea soon found much interest among theorists and experimenters, foremost in Alfven's laboratory in Stockholm. Whereas, theories concentrated on the energy coupling processes from ions to electrons, various situations in astrophysics, cometary and planetary sciences were proposed to be sites of this effect. It was soon recognized that space offered good possibilities for testing the effect in the absence of walls and with the availability of in-situ diagnostics of particles and waves without disturbing the process. It so happened that in the 1970's a series of rocket flights was to be performed in Esrange/Kiruna (Project Porcupine). The payloads, highly instrumented for plasma research in the auroral ionosphere, also carried a shaped charge experiment (see section Diagnostics of Electric Fields by Tracing Visible Plasma Clouds). The author, PI of the project, decided to dedicate one the shaped charge firings to studying the CIV effect (Haerendel, 1982).
Figure 15A explains the situation. The neutral barium jet contained a large fraction of atoms with velocities well above vcrit for barium, which is 2.7 km/s. In order to separate CIV ionization from photo-ionization, the injection occurred below the terminator for the lowest ionizing photons from the sun. The charge was pointed at an angle of 28° with respect to B, so that, after a short while, initially ionized barium would appear in sunlight and could be well-separated from subsequently photo-ionized particles. Figure 15B shows the result of the experiment of 1979 in terms of densitometer traces of the cloud as observed from ground: A narrow streak along the field lines through the injection point and a diffuse cloud to the right. Haerendel (1982) estimated that 30 % of the component above vcrit, i.e., between 15 and 20% of the total, had been ionized initially. Since the main payload was offset from the flux tube through the Ba jet, only fringe effects of the generated electromagnetic perturbations could be observed. The presentation of the observations was accompanied by theoretical considerations of the energy transfer process and of the limitations on the successful burning of the discharge. Both aspects were subsequently taken up in a great number of similar space experiments and theoretical investigations. Excellent reviews have been published by Brenning (1992), Lai et al. (1996), and Lai (2001).
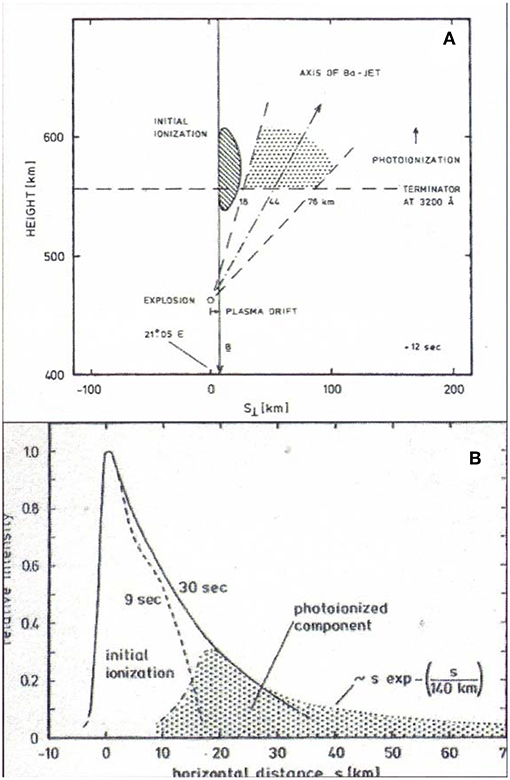
Figure 15. (A) Configuration of the injection of a barium jet at 560 km height and 100 km below the UV terminator at Esrange in 1979 with the goal to test the Critical Ionization Velocity effect. Inclination of the neutral beam with respect to the field lines allows separation of instantly generated ions from the photo-ionized ones when appearing in sunlight. (B) Densitometer traces of the ion distribution above the terminator. Nearly all ions to the left of 15 km are the result of the initial ionization (Haerendel, 1982).
The disturbing finding was that none of the following rocket experiments up to CRIT 2 in 1989 showed any CIV associated yield. Several theories tried to explain the reason for the discrepancy with the Porcupine result (e.g., Machida and Goertz, 1988; Torbert, 1988; Papadopoulos, 1992; Moghaddam-Taaheri and Goertz, 1993). However, finally the CRIT 2 rocket experiment (Torbert et al., 1992) and several releases from the CRRES satellite (Wescott et al., 1994) produced yields of at least a few percent. In CRIT 2, for instance, the release-rocket configuration was such that the energized electrons could be intercepted by the main payload directly along the field line of the explosion and compared with the optical yield. Still many questions remained with respect to the enormous differences found for the CIV related ionization between the various experiments. The cited references and many more to be found in these papers demonstrate to how much depth the exploration of the CIV process in space, laboratory, and natural environments has been subjected to carefully designed experiments, theory, and numerical modeling.
“Skidding” of Fast Plasma Clouds
The Combined Release and Radiation Effects Satellite (CRRES) was designed to perform a number of plasma injection experiments with diverse objectives, most of them along the lines of previous studies, such as diamagnetic cavities (cf. section Magnetic Cavities), magnetosphere-ionosphere coupling (cf. section Magnetosphere-Ionosphere Coupling), field line tracing and acceleration (cf. section Field-Parallel Acceleration), stimulating wave-particle interactions in the magnetosphere (cf. section Magnetospheric Modifications), and testing the CIV effect (cf. section Critical Ionization Velocity Experiments). However, a new goal was conceived under the name “skidding.” It was to study the initially delayed braking of a plasma cloud injected from an orbiting spacecraft into the upper ionosphere (350–450 km). What is new in comparison with the coupling of ion clouds to the ionosphere as discussed in section Relative Motions of Plasma Clouds and Auroral Arcs, is the impact of the high inertia of the injected ions. Mitchell et al. (1985) had studied the situation with numerical simulations and predicted that a 10 kg cloud would be able to skid for tens of seconds and tens of kilometers before coming to rest with respect to the ionosphere. The ion braking sets up a polarization field with E × B in the direction of the neutral cloud motion. Other than in the magnetosphere-ionosphere coupling discussed in section Magnetosphere-Ionosphere Coupling, the ambient ionosphere not only transfers momentum. Propagating almost instantly to the ionosphere, momentum is to be shared with the mass along the flux tube and with the region of current closure in the lower ionosphere. The motion decays due to dissipation by the Pedersen current, but with a delay because of the inertia temporarily stored in the background. The CRRES results (Huba et al., 1992) confirmed the predictions, however, skidding times and distances turned out to be lower than calculated, namely 3 s and 9 km for a 10 kg release. Further discussions on the current state of skidding theory and experiment can be found in a review paper by Winske et al. (2019).
Cycloid Bunching
At one of the CRRES barium releases at an altitude of 6,180 km a new phenomenon was observed, the formation of non-field-aligned curved structures (Bernhardt et al., 1993). The effect is explained as consequence of the motion of ions generated from a fast moving neutral cloud expanding as a spherical shell. If the linear motion of the spacecraft is faster than the cloud's expansion velocity, the trajectories of the injected ions are dominated by cycloidal motions transverse to B, while also proceeding along the field. At the cusps of the cycloids the transverse velocity goes to zero and the density is strongly enhanced. This leads to phase bunching as a purely kinetic effect, no instability involved. However, there may be modifications caused by the resulting electric polarization fields. The key issue underlying the appearance of visible structures is the Doppler shift and the deep absorption profile of the solar Fraunhofer line at 4,555 Å. Depending on the component of the gyromotion along the cloud-sun line, the brightness is varying with the cyclotron frequency. Unfortunately, the observing intensified CCD camera did not have the sensitivity to resolve the variations with gyro-frequency, however 2 sec exposures showed for about 10 s curved structures, alluding to the contour of the expanding neutral cloud. Bernhardt et al. (1993) supported the interpretation as due to cycloid bunching by an integral solution of the Vlasov equation for the evolution of the ion distributions including the Doppler effect. The observed phenomenon once more demonstrated that with space plasmas single particle effects can be important, in this case for the optical appearance, in the artificial comet experiment even for the overall dynamics.
Conclusions
Letting the here reported physical processes passing one's mind, one is induced to ask three questions: What has been and will remain unique? What has been superseded by later investigations with diagnostic instrumentation? And what is worth doing again? It is undisputable that what was described in section Diagnostics of Electric Fields by Tracing Visible Plasma Clouds, the role of the plasma clouds as tracers of natural motions, has been replaced by the development of double Langmuir probes for measuring electric fields. By contrast, the detailed insights into coupling processes in magnetosphere and solar wind summarized in section Coupling to the Ionosphere and Interaction With the Solar Wind are hard to obtain from orbiting vehicles in combination with ground-based measurements. Modifications of the ionosphere with plasma injections have only been partially successful. In the magnetosphere they did not succeed at all. Would it make sense to try again? The efforts would have to be substantially higher than those made in the past. On the other hand, the equatorial ionosphere and, in particular, the spread-F instabilities, have been thoroughly investigated by radar and in-situ rocket and satellite measurements. The physics of wave-particle interactions in the magnetosphere has been and still is being explored by many satellite missions in close cooperation with theory and simulations. In both cases there is little reason for new active experiments. The situation differs with respect to the stimulation of electron precipitation in the auroral ionosphere. Only few incomplete data sets are available. Little is really understood. There is no other way to clarify what is happening than with active experiments. By contrast, the CIV experiments with rockets and satellites have generated plenty of significant data and have stimulated intensive support by lab experiments and theory. Further space experiments are not likely to add much new information. Skidding and cycloid bunching are processes of minor general importance and the few experiments done can be considered as sufficiently well-understood.
We passed over the coupling processes treated in sections Coupling to the Ionosphere and Interaction With the Solar Wind without conclusions. The experiments were certainly unique and generated a wealth of new insights. It is hard to imagine how they could be replaced by diagnostics from orbiting satellites. Plasma cloud experiments supported by in situ and remote observations seem to be the only way. Can we say that there is enough material available and sufficiently developed understanding? While magnetospheric and ionospheric experiments have been continued into the early nineties by the CRRES mission, artificial comet experiments were done only twice by AMPTE. Would it be a good idea to undertake a new mission? It would most likely add new facets to the solar wind-plasma cloud interactions, clarify some details, but would probably not produce new fundamental insights. It has to be taken into account that the experimental and logistic efforts are great. Raising support for a new mission from a space agency would not be easy today. On the other hand, related physical processes are being and will continue to be explored with cometary and planetary missions.
We must accept that active plasma experiments in space were pioneering deeds of the past. They led to many unexpected discoveries and explored territory unknown at that time. Those actively involved experienced a most fascinating and often adventurous period of their lives. This short review tries to direct attention to the main achievements in understanding the encountered physical processes. It cannot convey the fascination.
Author Contributions
The author confirms being the sole contributor of this work and has approved it for publication.
Funding
Only institutional source: Max Planck Institute for extraterrestrial Physics.
Conflict of Interest Statement
The author declares that the research was conducted in the absence of any commercial or financial relationships that could be construed as a potential conflict of interest.
Acknowledgments
I was very fortunate to enter the group of Reimar Lüst in my last graduate years and participate from the beginning in development and exploitation of the barium cloud technique. Seven years later I found myself leading the group which was continuously expanding its technical competence allowing finally to realize Biermann's vision of an artificial comet with the AMPTE mission and the in-house built IRM spacecraft. Here I want to express my deep appreciation of the dedication and enthusiasm which guided my colleagues in realizing our research, often under severe pressure and with personal sacrifices. I want to thank in particular: Hans Neuss, Bernhard Meyer, Hermann Föppl, Erich Rieger, Arnoldo Valenzuela, Harald Frey, Leo Haser, Friedrich Melzner, Siegfried Drappatz, Karl-Wolfgang Michel, Wolfgang Brunner, Horst Hippmann, Bernd Häusler, Herwig Höfner, Jacob Stöcker, Peter Parigger, Rolf Schöning, Uwe Pagel, Johann Loidl, Kurt Gnaiger, Bernhard Merz, Werner Lieb, Werner Göbel, Theda Wendt, Ursula Höpstein, Karin Regenfelder, Helga Krombach, Barbara Mory, and Mariele Rieperdinger.
Furthermore, I profited greatly from scientific discussions with Reimar Lüst, Jacques Blamont, Hannes Alfvén, Carl-Gunne Fälthammar, Willi Stoffregen, Heinz Völk, Hans Kappler, Ulf Fahleson, Forrest Mozer, Michael Kelley, Charles Carlson, Roy Torbert, Hans Stenbaek- Nielsen, Gene Wescott, Jim Heppner, Dennis Papadopoulos, Stephen Mende, Paul Bernhardt, and naturally the whole AMPTE team.
References
Aggson, T. L. (1969). “Probe measurements of electric fields in space,” in Atmospheric Emissions, eds B. M. McCormac and A. Omholt (New York, NY: Van Nostrand Reinhold Company), 305–316.
Armstrong, E. B. (1963). Observations of luminous clouds produced in the upper atmosphere by exploding Grenades–I. Atomic emissions. Planet. Space Sci. 11, 733–742.
Baker, K. D., and Ulwick, J. C. (1978). Measurement of electron density structure in striated barium clouds. Geophys. Res. Lett. 5, 723–726.
Balsley, B. B., Haerendel, G., and Greenwald, R. A. (1972). Equatorial spread F: recent observations and a new interpretation. J. Geophys. Res. 77, 5625–5628.
Bates, D. R. (1950). A suggestion regarding the use of rockets to vary the amount of atmospheric sodium. J. Geophys. Res. 55:347. doi: 10.1029/JZ055i003p00347
Bernhardt, P. A. (1992). Probing the magnetosphere using chemical releases from the CRRES satellite. Phys. Fluids B 4, 2249–2256.
Bernhardt, P. A., Ballenthin, J. O., Baumgardner, J. L., Bhatt, A., Boyd, I. D., Burt, J. M., et al. (2012). Ground and space-based measurement of rocket engine burns in the ionosphere. IEEE Trans Plasma Sci. 40, 1267–1286.
Bernhardt, P. A., Huba, J. D., Pongratz, M. B., Simons, D. J., and Wolcott, J. H. (1993). Plasma irregularities caused by cycloid bunching of the CRRES G-2 barium release. J. Geophys. Res. 98, 1613–1627.
Bernhardt, P. A., Roussel-Dupre, R. A., Pongratz, M. B., Haerendel, G., Valenzuela, A., Gurnett, D. A., et al. (1987). Observations and theory of the AMPTE magnetotail barium releases. J. Geophys. Res. 92, 5777–5794.
Biermann, L., Lüst, R., Lüst, R. H., and Schmidt, H. U. (1961). Zur Untersuchung des interplanetaren Mediums mit Hilfe von künstlich eingebrachten Ionenwolken. Z. f. Astrophysik 53, 226–236.
Bingham, R., Bryant, D. A., Hall, D. S., Dawson, J. M., Kazeminejad, F., Su, J. J., et al. (1988). AMPTE observations and simulations results. Comput Phys Commun. 49, 257–266.
Blamont, J. E., Lory, M. L., and Courtes, G. (1960). Réactivité du sodium et du lithium dans la haute atmosphère. Ann. Geophys. 16:435.
Bogdanov, A. T., Sauer, K., Baumgärtel, K., and Shrivastava, K. (1996). Plasma structures of weakly outgassing comets–results from bi-ion fluid analysis. Planet. Space Sci. 44, 519–528.
Brecht, S. H., and Thomas, V. A. (1987). Three-dimensional simulation of an active magnetospheric release. J. Geophys. Res. 92, 2289–2304.
Brice, N. (1970). Artificial enhancement of energetic particle precipitation through cold plasma injection. J. Geophys. Res. 75, 4890–4892.
Brunner, W., Föppl, H., and Michel, K. W. (1970). Vaporization of metals in two-phase jets with various modes of energy deposition. Astronaut. Acta 15, 613–624.
Bryant, D. A., Hall, D. S., and Chaloner, C. P. (1985). “Energization of electrons following ion release in the solar wind,” in Proceedings if the 7th ESA Symposium (Paris: European Space Agency), 217.
Buchert, S., Baumjohann, W., Haerendel, G., La Hoz, C., and Lühr, H. (1988). Magnetometer and incoherent scatter observations of an intense Ps6 pulsation event. J. Atm. Terr. Phys. 50, 357–367.
Buchert, S., Haerendel, G., and Baumjohann, W. (1990). A model for the electric fields and currents during a strong Ps6 pulsation event. J. Geophys. Res. 95, 3733–3743.
Çakir, S., Haerendel, G., and Eccles, J. V. (1992). Modeling the ionospheric response to artificially produced density enhancements. J. Geophys. Res. 97, 1193–1207.
Carlson, C. W., Pfaff, R. F., and Watzin, J. G. (1998). The fast auroral snapshot (FAST) mission. Geophys. Res. Lett. 25, 2013–2016.
Carlsten, J. L. (1975). Photoionization of barium clouds via the 3D metastable levels. Planet. Space Sci. 23, 53–60.
Cheng, A. F. (1987). Transverse deflection and dissipation of small plasma beams and clouds in magnetic media. J. Geophys. Res. 92, 55–63.
Cornwall, J. M. (1974). Magnetosphere dynamics with artificial plasma clouds. Space Sci. Rev. 15, 841–860.
Cuperman, S., and Landau, R. W. (1974). On the enhancement of the whistler mode instability in the magnetosphere by cold plasma injection. J. Geophys. Res. 69, 128–134.
Drappatz, S. W. (1972). The radiative transfer problem I freely expanding gaseous clouds and its application to barium cloud experiments. Planet. Space Sci. 20, 663–682.
Edwards, H. D., Bedinger, J. F., Manring, E. R., and Cooper, C. D. (1956). The Airglow and the Aurorae, eds E. B. Armstrong and A. Dalgarno (London: Pergamon Press), 122.
Ergun, R. E. (2002). Parallel electric fields in the upward current region of the aurora: numerical solutions. Phys. Plasmas 9, 3695–3704. doi: 10.1063/1.1499121
Evans, D. S. (1974). Precipitation electron fluxes formed by a magnetic-field-aligned potential difference. J. Geophys. Res. 79, 2853–2858. doi: 10.1029/JA079i019p02853
Fahleson, U. V., Fälthammar, C.-G., Pedersen, A., Knott, K., Brommundt, G., Schumann, G., et al. (1971). Simultaneous electric field measurements made in the auroral ionosphere by using three independent techniques. Radio Sci. 6, 233–245.
Föppl, H., Haerendel, G., Haser, L., Loidl, J., Lütjens, P., Lüst, R., et al. (1967). Artificial strontium and barium clouds in the upper atmosphere. Planet. Space Sci. 15, 357–372.
Föppl, H., Haerendel, G., Loidl, J., Lüst, R., Melzner, F., Meyer, B., et al. (1965). Preliminary experiments for the study of the interplanetary medium by the release of metal vapour in the upper atmosphere. Planet. Space Sci. 13, 95–114
Frey, H. U., Haerendel, G., Mende, S. B., Forrester, W. T., Immel, T. J., and Østgaard, N. (2004). Subauroral morning proton spots (SAMPS) as a result of plasmapause-ring-current interaction. J. Geophys. Res. 109:305. doi: 10.1029/2004JA010516
Fuselier, S. A., Mende, S. B., Geller, S. P., Miller, M., Hoffman, R. A., Wygant, J. R., et al. (1994). Dynamics of the CRRES barium releases in the magnetosphere. J. Geophys. Res. 99, 17379–17389.
Groves, G. V. (1960). “Wind and temperature results obtained in Skylark experiments,” in Space Research, ed H. Kallmann Bijl (Amsterdam: North-Holland Publ. Co.), 144–153.
Gurnett, D. A., Anderson, R. R., Ma, T. Z., Haerendel, G., Paschmann, G., Bauer, O. H., et al. (1986). Analysis and interpretation of the shocklike electrostatic noise observed during the AMPTE solar wind lithium releases. J. Geophys. Res. 91, 1201–1310.
Haerendel, G. (1972). “Plasma drifts in the auroral ionosphere derived from barium releases,” in Earth's Magnetospheric Processes, ed B. M. McCormac (Dordrecht, D. Reidel), 146–257.
Haerendel, G. (1973). Results from barium cloud releases in the ionosphere and magnetosphere. Space Res. 2, 601–617.
Haerendel, G. (1982). Alfvén critical velocity effect tested in space. Z. Naturforsch. 37a, 728–735.
Haerendel, G. (1987). “Tail exploration and tail formation with artificial plasma clouds,” in Proceedings of the Chapman Conference on Magnetosphere, September 1985, in 'Monograph on Magnetotail Physics', ed A. T. Y. Lui (Johns Hopkins University Press), 337–350.
Haerendel, G. (1996). Curiosity and chance. J. Geophys. Res. 101, 10541–10553. doi: 10.1029/96JA00136
Haerendel, G. (2007). Auroral arcs as sites of magnetic stress release. J. Geophys. Res. 112:214. doi: 10.1029/2007JA012378
Haerendel, G. (2014). M-I coupling and energy dumping. Geophys. Res. Lett. 41, 1846–1853. doi: 10.1002/2014GL059582
Haerendel, G. (2015). Substorm onset: current sheet avalanche and stop layer. J. Geophys. Res. Space Phys. 120, 1697–1714. doi: 10.1002/2014JA020571
Haerendel, G., Bauer, O. H., Çakir, S., Föppl, H., Rieger, E., and Valenzuela, A. (1983). “Coloured bubbles, an experiment for triggering equatorial spread F,” in Proceedings of an International Symposium on Active Experiments in Space, ESA SP-195 (Paris), 295–298.
Haerendel, G., Buchert, S., La Hoz, C., Raaf, B., and Rieger, E. (1993). On the proper motion of auroral arcs. J. Geophys. Res. 98, 6087–6099. doi: 10.1029/92JA02701
Haerendel, G., Eccles, J. V., and Çakir, S. (1992). Theory for modeling the equatorial evening ionosphere and the origin of shear in the horizontal plasma flow. J. Geophys. Res. 97, 1209–1223. doi: 10.1029/91JA02226
Haerendel, G., Hedgecock, P. C., and Akasofu, S.-I. (1971). Evidence for magnetic field-aligned currents during the substorms of March 18, 1969. J. Geophys. Res. 76, 2382–2395. doi: 10.1029/JA076i010p02382
Haerendel, G., and Lüst, R. (1968a). “Electric fields in the upper atmosphere,” in Earth's Particles and Fields, ed B. M. McCormac (Dordrecht: Reinhold Publishing Corporation), 271–285.
Haerendel, G., Lüst, R., and Rieger, E. (1967). Motion of artificial ion clouds in the upper atmosphere. Planet. Space Sci. 15, 1–18.
Haerendel, G., Lüst, R., Rieger, E., and Völk, H. (1969). “Highly irregular artificial clouds in the auroral zone,” in Atmospheric Emissions, ed B. M. McCormac and A. Omholt (New York, NY: Van Nostrand Reinhold Comp), 293–303.
Haerendel, G., and Mende, S. B. (2012). Magnetosphere-ionosphere coupling and scale breaking of a plasma cloud in the magnetosphere. J. Geophys. Res. 117:233. doi: 10.1029/2012JA018021
Haerendel, G., Paschmann, G., Baumjohann, W., and Carlson, C. W. (1986). Dynamics of the AMPTE artificial comet. Nature 320, 720–723. doi: 10.1038/320720a0
Haerendel, G., Rieger, E., Valenzuela, A., Föppl, H., Stenbaek-Nielsen, H. C., and Wescott, E. M. (1976). “First observation of electrostatic acceleration of barium ions into the magnetosphere,” in Proceedings of the Symposium on Present and Future European Sounding Rocket and Balloon Research in the Auroral Zone, Schloß Elmau, ESA SP-155, (Neuilly), 203–211.
Harang, O. (1969). “Summary on spectroscopy of rocket releases,” in Atmospheric Emissions, ed B. M. McCormac and A. Omholt (New York, NY: Van Nostrand Reinhold Company), 489–499.
Haser, L. (1967). “Use of artificial barium clouds to study magnetic and electric fields in the atmosphere,” in Aurora and Airglow, ed B. M. McCormac (New York, NY: Reinhold Publishing Corporation), 391–403.
Heppner, J. P. (1969). “Magnetospheric convection patterns inferred from high latitude activity,” in Atmospheric Emissions, eds B. M. McCormac and A. Omholt (New York, NY: Van Nostrand Reinhold Company), 251–266.
Heppner, J. P. (1971). Electric-field measurements and the identification of currents causing magnetic disturbances in the polar cap. J. Geophys. Res. 76, 6028–6053.
Heppner, J. P., Miller, M. L., Pongratz, M. B., Smith, G. M., Smith, L. L., Mende, S. B., et al. (1981). The Cameo barium releases: E|| fields over the polar cap. J. Geophys. Res. 86, 3519–3542. doi: 10.1029/JA086iA05p03519
Holmgren, G., Boström, R., Kelley, M. C., Kintner, P. M., Lundin, R., Fahleson, U. V., et al. (1980). Trigger, an active release experiment that stimulated auroral particle precipitation and wave emissions. J. Geophys. Res. 85, 5403–5053. doi: 10.1029/JA085iA10p05043
Holmgren, G., Marklund, G., Eliasson, L., Opgenoorth, H., Söraas, F., Primdahl, F., et al. (1988). Ionospheric response to chemical releases in the high-latitude E and F regions. Adv. Space Res. 8,79–83. doi: 10.1016/0273-1177(88)90345-6
Huba, J. D., Bernhardt, P. A., and Lyon, J. G. (1992). Preliminary study of the CRRES magnetospheric barium releases. J. Geophys. Res. 97, 11–24. doi: 10.1029/91JA02144
Huba, J. D., Lyon, J. G., and Hassam, A. B. (1987). Theory and simulation of the Rayleigh-Taylor instability in the limit of large Larmor radius. Phys. Rev. Lett. 59, 2971–2974. doi: 10.1103/PhysRevLett.59.2971
Hudson, M. K., and Kennel, C. F. (1975). Linear theory of equatorial spread F. J. Geophys. Res. 80, 4581–4590.
Johnson, A. L., and Hocutt, A. M. (1984). Scintillation results from ionospheric modification experiment. Radio Sci. 19, 741–748. doi: 10.1029/RS019i003p00741
Kelley, M. C. (1989). The Earth's Ionosphere-Plasma Physics and Electrodynamics, International Geophysics Series 43. San Diego, CA: Academic Press, Inc.
Kelley, M. C., Fahleson, U. V., Holmgren, G., Boström, R., Kintner, P. M., and Kudeki, E. (1980). Generation and propagation of an electromagnetic pulse in the Trigger experiment and its possible role in electron acceleration. J. Geophys. Res. 85, 5055–5060. doi: 10.1029/JA085iA10p05055
Kelley, M. C., Haerendel, G., Kappler, H., Mozer, F. S., and Fahleson, U. V. (1975). Electric field measurements in a major magnetospheric substorm. J. Geophys. Res. 80, 3181–3195. doi: 10.1029/JA080i022p03181
Kelley, M. C., Pedersen, A., Fahleson, U. V., Jones, D., and Köhn, D. (1974). Active experiments stimulating waves and particle precipitation with small ionospheric barium releases. J. Geophys. Res. 79, 2859–2867. doi: 10.1029/JA079i019p02859
Kelley, M. C., Pfaff, R., Baker, K. D., Ulwick, J. C., Livingston, R., Rino, C., et al. (1982). Simultaneous rocket probe and radar measurements of equatorial spread F –transitional and short wavelength results. J. Geophys. Res. 87, 1575–1588.
Kennel, C. F., and Petschek, H. E. (1966). Limit on stably trapped particle fluxes. J. Geophys. Res. 71, 1–28. doi: 10.1029/JZ071i001p00001
Kintner, P. M., Kelley, M. C., Holmgren, G., and Boström, R. (1980). The observation and production of ion acoustic waves during the Trigger experiment. J. Geophys. Res. 85, 5071–5077. doi: 10.1029/JA085iA10p05071
Krimigis, S. M., Haerendel, G., Gloeckler, G., McEntire, R. W., Shelley, E. G., Decker, R. B., et al. (1986). AMPTE lithium tracer releases in the solar wind: observations inside the magnetosphere. J. Geophys. Res. 91, 1339–1353. doi: 10.1029/JA091iA02p01339
Krimigis, S. M., Haerendel, G., McEntire, R. W., Paschmann, G., and Bryant, D. A. (1982). The active magnetospheric particle tracer explorers (AMPTE) program. Johns Hopkins Apl Tech. Dig. 63, 843–850. doi: 10.1029/EO063i045p00843
Lai, S. T. (2001). A review of critical ionization velocity. Rev. Geophys. 39, 471–506. doi: 10.1029/2000RG000087
Lai, S. T., McNeil, W. J., and Murad, E. (1996). The yield of ionization in critical ionization velocity space experiments. Astrophys. Space Sci. 235, 269–287. doi: 10.1007/BF00644447
Linson, L. M., and Workman, J. B. (1970). Formation of striations in ionospheric plasma clouds. J. Geophys. Res. 75, 3211–3219. doi: 10.1029/JA075i016p03211
Lloyd, K. H., and Haerendel, G. (1973). Numerical modeling of the drift and deformation of ionospheric plasma clouds and of their interaction with other layers of the ionosphere. J. Geophys. Res. 78, 7389–7415. doi: 10.1029/JA078i031p07389
Lundin, R., and Holmgren, G. (1980). Rocket observations of stimulated electron acceleration associated with the Trigger experiment. J. Geophys. Res. 85, 5061–5070. doi: 10.1029/JA085iA10p05061
Machida, S., and Goertz, C. K. (1988). The electromagnetic effect on the critical ionization velocity process. J. Geophys. Res. 93, 11495–11506. doi: 10.1029/JA093iA10p11495
Marklund, G., Brenning, N., Holmgren, G., and Haerendel, G. (1988). A model for transient electric fields associated with chemical release experiments by rockets. Adv. Space Res. 8, 85–92. doi: 10.1016/0273-1177(88)90346-8
McDonald, B. E., Ossakow, S. L., Zalesak, S. T., and Zabusky, N. J. (1981). Scale sizes and lifetimes of F region plasma cloud striations as determined by the condition of marginal stability. J. Geophys. Res. 86, 5775–5784. doi: 10.1029/JA086iA07p05775
McIlwain, C. E. (1960). Direct measurement of particles producing visible aurora. J. Geophys. Res. 65, 2727–2747. doi: 10.1029/JZ065i009p02727
Mendillo, M. (1988). Ionospheric holes: a review of theory and recent experiments. Adv. Space Res. 8, 51–62. doi: 10.1016/0273-1177(88)90342-0
Mendillo, M., Hawkins, G. S., and Klobuchar, J. A. (1975). A large-scale hole in the ionosphere caused by the launch of Skylab. Science 187, 343–346. doi: 10.1126/science.187.4174.343
Mitchell, K. G. Jr., Fedder, J. A., Huba, J. D., and Zalesak, S. T. (1985). Transverse motion of high-speed barium clouds in the ionosphere. J. Geophys. Res. 90, 11091–11095. doi: 10.1029/JA090iA11p11091
Möbius, E., Hovestadt, D., Klecker, B., Scholer, M., Gloeckler, G., and Ipavich, F. M. (1985). Direct observation of He+ pick-up ions of interstellar origin in the solar wind. Nature 318, 426–429. doi: 10.1038/318426a0
Möbius, E., Hovestadt, D., Klecker, B., Scholer, M., Gloeckler, G., Ipavich, F. M., et al. (1986). Observation of lithium pick-up ions in the 5 to 20 keV energy range following the AMPTE solar wind releases. J. Geophys. Res. 91, 1325–1332. doi: 10.1029/JA091iA02p01325
Möbius, E., Klecker, B., Hovestadt, D., and Scholer, M. (1988). Interaction of interstellar pick-up ions with the solar wind. Astrophys. Space Sci. 144, 487–505.
Moghaddam-Taaheri, E., and Goertz, C. K. (1993). Numerical quasi-linear study of the critical ionization velocity phenomenon. J. Geophys. Res. 98, 1443–1460. doi: 10.1029/92JA02237
Mozer, F. S., and Bruston, P. (1967). Electric field measurements in the auroral ionosphere. J. Geophys. Res. 72, 1109–1114.
Narcisi, R. S. (1983). “Overview of project BIME,” in Proceedings of an International Symposium on Active Experiments in Space, ESA SP-195 (Neuilly), 255–264.
Papadopoulos, K. (1992). The CIV processes in the CRIT experiments. Geophys. Res. Lett. 19, 605–608. doi: 10.1029/92GL00405
Papadopoulos, K., Huba, J. D., and Lui, A. T. Y. (1987). Collisionless coupling in the AMPTE artificial comet. J. Geophys. Res. 92, 47–54. doi: 10.1029/JA092iA01p00047
Paschmann, G., Carlson, C. W., Baumjohann, W., Loidl, H., Curtis, D. W., Sckopke, N., et al. (1986). Plasma observations on AMPTE/IRM during the lithium releases in the solar wind. J. Geophys. Res. 91, 1271–1281. doi: 10.1029/JA091iA02p01271
Perkins, F. W., Zabusky, N. J., and Doles, J. H. III. (1973). Deformation and striation of plasma clouds in the ionosphere. J. Geophys. Res. 78:697–709. doi: 10.1029/JA078i004p00697
Rees, D., Hallinan, T. J., Stenbaek-Nielsen, H. C., Mendillo, M., and Baumgardner, J. (1986). Optical observations of the AMPTE artificial comet from the northern hemisphere. Nature 320, 704–708. doi: 10.1038/320704a0
Rees, J. A. (1961). Diffusion coefficients determined from sodium vapour trails. Planet. Space Sci. 8, 35–36. doi: 10.1016/0032-0633(61)90116-7
Rosenberg, N. W. (1971). Observations of striation formation in a barium ion cloud. J. Geophys. Res. 76, 6856–6864.
Sauer, K., Bogdanov, A. T., and Baumgärtel, K. (1994). Evidence of an ion composition boundary (protonopause) in bi-ion fluid simulations of solar wind mass loading. Geophys. Res. Lett. 21, 2255–2258. doi: 10.1029/94GL01691
Scholer, M. (1970). On the motion of artificial ion clouds in the magnetosphere. Planet. Space Sci. 18, 977–1004. doi: 10.1016/0032-0633(70)90101-7
Stewart, B. (1882). “Terrestrial magnetism,” in Encyclopaedia Britannica, 9th ed. Vol. 16, eds T. S. Baynes and W. R. Smith (Edinburgh: A & C Black), 159–184.
Stoffregen, W. (1970). Electron density variation observed in the E-layer below an artificial barium cloud. J. Atmos. Terr. Phys. 32, 171–177. doi: 10.1016/0021-9169(70)90189-3
Swift, D. W., Stenbaek-Nielsen, H. C., and Hallinan, T. J. (1976). An equipotential model for auroral arcs. J. Geophys. Res. 81, 3931–3934.
Szegö, K., Glassmeier, K.-H., Bingham, R., Bogdanov, A., Fischer, C., Haerendel, G., et al. (2000). Physics of mass loaded plasmas. Space Sci. Rev. 94, 421–671.
Torbert, R. B. (1988). Critical velocity experiments in space. Adv. Space Res. 8, 39–49. doi: 10.1016/0273-1177(88)90341-9
Torbert, R. B., Kletzing, C. A., Liou, K., and Rau, D. (1992). Prompt ionization in the CRIT II barium releases. Geophys. Res. Lett. 19, 973–976. doi: 10.1029/91GL02777
Valenzuela, A., Haerendel, G., Föppl, H., Melzner, F., Neuss, H., Rieger, E., et al. (1986). The AMPTE artificial comet experiments. Nature 320, 700–703. doi: 10.1038/320700a0
Völk, H. J., and Haerendel, G. (1971). Striations in ionospheric ion clouds. J. Geophys. Res. 76, 4541–4559. doi: 10.1029/JA076i019p04541
Wescott, E. M., Peek, H. M., Stenbark-Nielsen, H. C., Murcray, W. B., Jensen, R. J., and Davis, T. N. (1972). Two successful field line tracing experiments. J. Geophys. Res. 77, 2982–2986.
Wescott, E. M., Stenbaek-Nielsen, H. C., Davis, T. N., Murcray, W. B., Peek, H. M., and Bottoms, P. J. (1975). The L = 6.6 Oosik barium plasma injection experiment and magnetic storm of March 7, 1972. J. Geophys. Res. 80, 951–967.
Wescott, E. M., Stenbaek-Nielsen, H. C., Hallinan, T., Deehr, C., Romick, J., Olson, J., et al. (1985). Plasma-depleted holes, waves, and energized particles from high-altitude explosive plasma perturbation experiments. J. Geophys. Res. 90, 4281–4298.
Wescott, E. M., Stenbaek-Nielsen, H. C., Hallinan, T. J., Davis, T. N., and Peek, H. M. (1976). The Skylab barium plasma injection experiments 2. Evidence for a double layer. J. Geophys. Res. 81, 4495–4502.
Wescott, E. M., Stenbaek-Nielsen, H. C., Hampton, D. L., and Delamere, P. A. (1994). Results of critical velocity experiments with barium, strontium and calcium releases from the CRRES satellite. J. Geophys. Res. 99, 2145–2158. doi: 10.1029/93JA01921
Wescott, E. M., Stolarik, J. D., and Heppner, J. P. (1969). Electric fields in the vicinity of auroral forms from motions of barium vapor releases. J. Geophys. Res. 74, 3469–3487.
Wescott, E. M., Stolarik, J. D., and Heppner, J. P. (1970). “Auroral and polar cap electric fields from barium releases,” in Particles and Fields in the Magnetosphere, ed B. M. McComac (Dordrecht: D. Reidel Publishing Company), 229–253.
Whalen, B. A., Yau, A. W., Creutzberg, F., Wallis, D. D., McNamara, A. G., Harris, F. R., et al. (1985). Waterhole auroral arc modification experiments: electrodynamic response. J. Geophys. Res. 90, 8377–8386. doi: 10.1029/JA090iA09p08377
Winske, D., Huba, J. D., Niemann, C., and Ari, L. (2019). Recalling and updating research on diamagnetic cavities: experiments, theory, simulations. Front Astr. Space Sci. 5:51. doi: 10.3389/fspas.2018.00051
Woodman, R. F., and La Hoz, C. (1976). Radar observations of F region equatorial irregularities. J. Geophys. Res. 75, 5447–5466.
Yau, A. W., and Whalen, B. A. (1988). Auroral perturbation experiments. Adv. Space Res. 8, 67–77. doi: 10.1016/0273-1177(88)90344-4
Yau, A. W., Whalen, B. A., Creutzberg, F., Pongratz, M. B., and Smith, G. (1981). Observations of particle precipitation, electric field, and optical morphology of an artifically perturbed auroral arc: project waterhole. J. Geophys. Res. 86, 5601–5613.
Zabusky, N. J., Doles, J. H. III., and Perkins, F. W. (1973). Deformation and striation of plasma clouds in the ionosphere, 2. Numerical simulation of a nonlinear two-dimensional model. J. Geophys. Res. 78, 711–724. doi: 10.1029/JA078i004p00711
Zalesak, S. T., Ossakow, S. L., and Chaturvedi, P. K. (1982). Nonlinear equatorial spread F: the effect of neutral winds and bacckground Pedersen conductivity. J. Geophys. Res. 87, 151–166. doi: 10.1029/JA087iA01p00151
Zmuda, A. J., Armstrong, J. C., and Heuring, F. T. (1970). Characteristics of transverse magnetic disturbances observed at 1100 km in the auroral oval. J. Geophys. Res. 75, 4757–4762.
Keywords: barium clouds, coupling to ionosphere, modification experiments, artificial comets, critical ionization velocity, auroral acceleration, auroral stimulations
Citation: Haerendel G (2019) Experiments With Plasmas Artificially Injected Into Near-Earth Space. Front. Astron. Space Sci. 6:29. doi: 10.3389/fspas.2019.00029
Received: 11 December 2018; Accepted: 01 April 2019;
Published: 30 April 2019.
Edited by:
Gian Luca Delzanno, Los Alamos National Laboratory (DOE), United StatesReviewed by:
Paul A. Bernhardt, United States Naval Research Laboratory, United StatesKonstantinos Papadopoulos, University of Maryland, College Park, United States
Copyright © 2019 Haerendel. This is an open-access article distributed under the terms of the Creative Commons Attribution License (CC BY). The use, distribution or reproduction in other forums is permitted, provided the original author(s) and the copyright owner(s) are credited and that the original publication in this journal is cited, in accordance with accepted academic practice. No use, distribution or reproduction is permitted which does not comply with these terms.
*Correspondence: Gerhard Haerendel, aGFlQG1wZS5tcGcuZGU=