- 1Centre for Bioinnovation, University of the Sunshine Coast, Sippy Downs, QLD, Australia
- 2School of Science, Technology and Engineering, University of the Sunshine Coast, Sippy Downs, QLD, Australia
Arachnid venom peptides receive increasing attention from researchers for possible applications as human therapeutics, as bioinsecticides in agriculture or for targeting vectors of human disease. One commonly perceived disadvantage of peptides in contrast to small molecule drugs is their inability of crossing biological membranes comprised of lipid bilayers, providing a major obstacle for the delivery of peptide-based drugs and bioinsecticides. However, some arachnid venom peptides were reported to cross biological membranes, including cellular membranes, the vertebrate and insect blood brain barrier (BBB) and the insect midgut epithelium. This review will focus on these membrane-permeating arachnid peptides and discuss the underlying mechanisms. Different physico-chemical properties of membrane-permeating arachnid peptides and their contribution to the ability of crossing biological membranes will also be examined. In addition, several methods that facilitate or enable peptides to cross biological membranes will be discussed, which can be employed on peptides with no inherent membrane-permeating capabilities.
1 Introduction
Arachnid venoms are known to be complex chemical cocktails comprised of a mixture of small inorganic and organic molecules, large proteins and enzymes, linear peptides, and cystine-rich peptides (Lüddecke et al., 2022). These components exhibit various functions such as cytolytic, antimicrobial, and insecticidal activities, making them valuable for bio-insecticide and bio-therapeutic discovery (Saez et al., 2010; Windley et al., 2012). Venom peptides usually target various voltage-gated (e.g. sodium, calcium or potassium) or ligand-gated (e.g. acid-sensing) ion channels, glutamate receptors and transient receptor potential channels) as well as other signaling pathways in the central nervous system and neuromuscular junctions (De Lima et al., 2007; Lüddecke et al., 2022; Saez and Herzig, 2019). To assess the potential of venom peptides for therapeutic or agricultural applications, we need to understand how they access their targets, e.g. via traversing biological barriers such as the blood-brain barrier (BBB) or the digestive tract epithelium. Ultimately, this process involves the crossing of cell membranes, which are comprised of a lipid bilayer that compartmentalizes cells (Harayama and Riezman, 2018).
As arachnid venoms are injected into their prey or predators, there was no evolutionary drive to develop oral activity. Nevertheless, some arachnid venom peptides demonstrated oral activity against arthropods (Hardy et al., 2013; Monfared et al., 2022; Mukherjee et al., 2006). The astounding physico-chemical stability of arachnid venom peptides provided by disulfide-rich knottin structures like the inhibitor cystine knot (ICK) motif certainly contributes to their survival in harsh environments like the insect midgut (Pallaghy et al., 1994). However, surviving the midgut is not sufficient for oral activity, the insecticidal peptides also need to traverse epithelial cells lining the insect midgut. Oral activity varies between peptides and insect taxa and only the combination of stability within the midgut and an efficient means of traversing the midgut epithelium will enable oral activity. Although numerous studies recorded the information of protein absorption (hemoglobin, albumin, IgG) in both blood-feeding and non-blood-feeding arthropods (Jeffers and Michael Roe, 2008), very little is known about peptide movement across the insect midgut (Jeffers et al., 2005). The accumulation of orally delivered snowdrop lectin (Galanthus nivalis agglutinin: GNA) was demonstrated in the hemolymph of the lepidopteran Lacanobia oleracea and the hemipteran Nilaparvata lugens (Fitches and Gatehouse, 1998; Fitches et al., 2001; Gatehouse et al., 1998), promoting the application of GNA as vector for insecticidal peptides (Fitches et al., 2002). Common techniques for studying protein and peptide movement across the digestive tract include ELISA, western blots, and immunohistochemistry, comparing the concentration of protein/peptide between midgut and hemolymph (Jeffers and Michael Roe, 2008). In vitro membrane penetration assays using Ussing chambers combined with mass spectrometric detection are also frequently employed to determine peptide quantities on both sides of the midgut epithelium (Denecke et al., 2018; Herzig et al., 2018).
Besides oral insecticidal activity for agricultural applications of arachnid venom peptides, some therapeutic applications might necessitate delivery into the central nervous system (CNS) of vertebrates. The BBB in vertebrates shields the CNS from neurotoxic substances entering the circulatory system, which complicates the delivery of CNS-active drugs into the brain and spinal cord. Traditionally, CNS-active drugs are administrated by invasive delivery strategies, such as intrathecal injection, intraventricular drug infusion or local intracerebral implants (Temsamani et al., 2001). However, due to the inherent risks of physically breaching the BBB, the development of non-invasive therapeutic strategies has received increasing attention. This includes identifying BBB-penetrating peptides or vector-mediated endogenous transport pathways such as carrier or receptor-mediated transport (Zhou et al., 2021). Based on previous data, only 2% of lipophilic small molecules have the capacity of crossing the BBB to reach their therapeutic targets, and nearly 98% of small molecules (<500 Da) and 100% of large molecules including peptides and proteins fail to penetrate the BBB without effective delivery systems (Zhou et al., 2021; Zou et al., 2013). Cell-penetrating peptides, such as rabies virus glycoprotein (RDP) (Fu et al., 2012) and human immunodeficiency virus-1 (HIV-1) (Frankel and Pabo, 1988), are considered efficient carriers for CNS drug delivery (Zou et al., 2013), albeit with distinct mechanisms (El-Andaloussi et al., 2005). Cell penetrating activity is also employed by some venom peptides, although the underlying mechanism remains unclear.
Considering the significant potential of arachnid venom peptides in pharmacology and agronomy, the mechanism of how they cross biological membranes deserves further attention. The present review will therefore shed some light on the capacity and characteristics of arachnid venom peptides penetrating biological membranes with a detailed focus on the underlying mechanisms.
2 Cell membranes and cell-penetrating peptides
Cell membranes consist of a lipid bilayer, which is a biological membrane composed of two layers of lipid molecules, each containing a hydrophilic head and a hydrophobic tail. Mammalian membrane lipids mainly include phosphatidylcholine (PC), phosphatidylethanolamine (PE), cholesterol, phosphatidylserine (PS), glycolipids, and sphingolipids (Cockcroft, 2021). In contrast, the membrane composition in insect cells differs significantly from that of mammalian cells. Insect cells have shorter fatty chains: a higher content of PE and phosphatidylinositol (PI) and lower levels of PS, glycolipids, sphingolipids, and cholesterol (Alberts B and Lewis, 2002). Additionally, insect cell membranes contain a lower saturation of fatty acid chains. It is generally believed that insects have different membrane lipid compositions to adapt to lower body temperatures (Marheineke et al., 1998).
Cell-penetrating peptides (CPPs) have been shown to cross cell membranes either via endocytosis or direct permeation (Jiao et al., 2009). Most known CPPs are typically rich in positively charged residues like arginine and lysine (Duchardt et al., 2007; Skotland et al., 2015; Trabulo et al., 2010). The guanidinium group in arginine and the amine group in lysine play important roles in interacting with negatively charged surfaces on the cell membrane, as well as forming hydrophobic alpha helical structures in membrane bilayers. Cationic CPPs can enter the cell by directly penetrating cell membranes or by triggering endocytosis-independent uptake (Green et al., 1989; Nakase et al., 2004). In case of transfer by endocytosis, CPPs are packed into endosomes and must escape from endosomes before being transported to lysosomes for degradation. The high content of cationic amino acids in CPPs was shown to modify the cytomembrane pH, resulting in endosomal escape of CPPs (Magzoub et al., 2005; Takechi-Haraya and Saito, 2018). Amphipathic peptides comprise another group of CPPs, which translocate through the cell membrane by forming multi-peptide complexes with the hydrophobic domains facing outward and the hydrophilic domains facing inward, resulting in direct translocation through the bilayer (Deshayes et al., 2006).
The efficiency of cationic CPP entry is largely influenced by the lipid composition of the membrane (Islam et al., 2018). Experiments using a mouse endothelioma cell line revealed that longer fatty acid chains and higher cholesterol content result in less stable endosomes, leading to a reduced rate of CPP entry (Islam et al., 2018). Therefore, the shorter fatty acid chains in insect cell membranes may lead to higher permeability for CPPs compared to mammalian cell membranes. However, further experiments are required to confirm how the lipid composition in insects might affect the selectivity and efficiency of CPPs.
2.1 Arachnid CPPs
Eight arachnid CPPs are reported from four spider and from four scorpion species, respectively, with six of them being cationic and two amphipathic (WaTx and LETX-VI) as shown in Table 1; Figures 1, 2. The CPPs for which the mechanism has been investigated are latarcin 1, lycosin-1 and LETX-VI. The antimicrobial peptide latarcin 1 and the antitumor peptide lycosin-1 were the first spider venom peptides being investigated for cellular entry via endocytosis (Ponnappan and Chugh, 2017; Tan et al., 2017). Cold temperature and endocytosis inhibitors significantly reduce the membrane translocation rate of both latarcin 1 and lycosin-1 (Ponnappan and Chugh, 2017; Tan et al., 2017; Zhang et al., 2020). The amphipathic peptide LETX-VI from the eggs of black widow spiders Latrodectus tredecimguttatus was shown to penetrate cell membranes and promote dopamine release. Tang and colleagues discovered that LETX-VI penetrates PC12 cell membranes via the endocytosis pathway, using the vesicular transmembrane protein synaptotagmin 1 as a receptor (Tang et al., 2022).
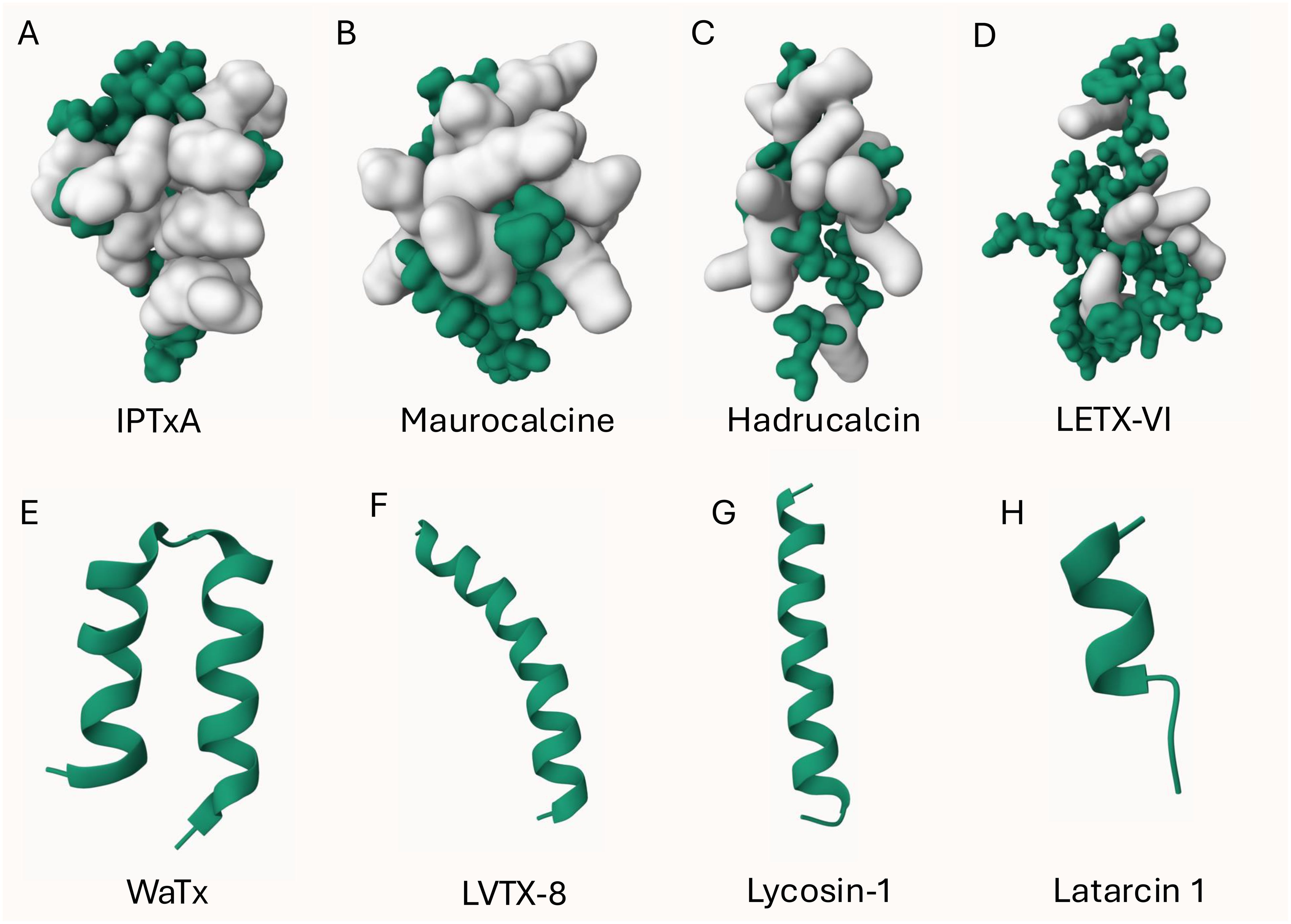
Figure 1. 3D structure of arachnid CPPs. The ICK peptide (A-D) structures were displayed using Gaussian surface in green and basic residues (R, H, K) were highlighted in white. Non-ICK peptides (E-H) structures were displayed using cartoon in green. (A): obtained from PDB ID: 1IE6; (B): obtained from PDB ID: 1C6W; (E): obtained from PDB ID: 6OFA. The other structures were predicted by AlphaFold 3 (Jumper et al., 2021; Varadi et al., 2024).
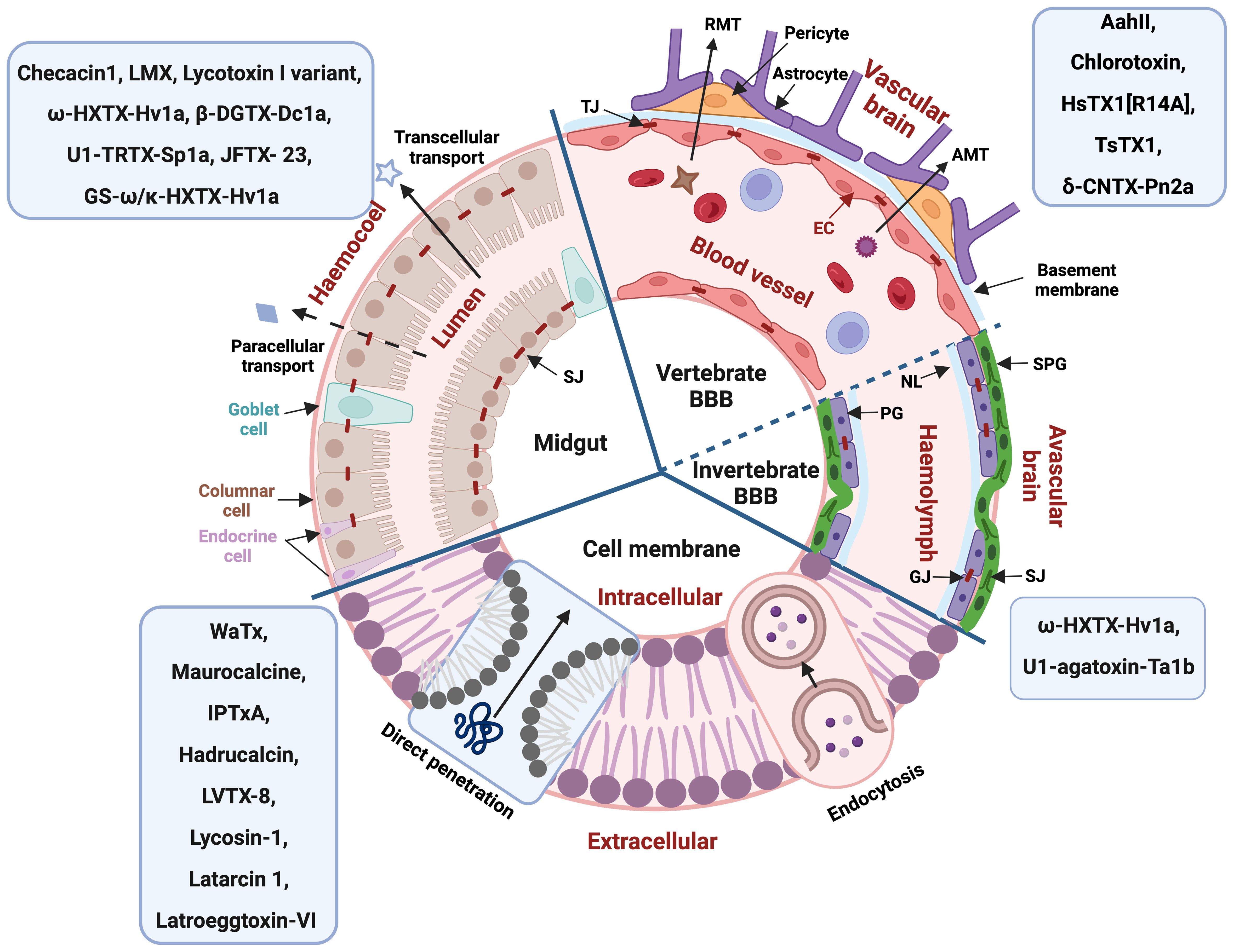
Figure 2. General structure of different biological barriers with arachnid peptides known to permeate these barriers indicated in the blue boxes. BBB, blood-brain barrier; RMT, receptor-mediated transcytosis; AMT, adsorptive-mediated transcytosis; TJ, tight junction; SJ, septate junction; GJ, gap junction; EC, epithelial cell; NL, Neural lamella; PG, perineurial glia cells; SPG, subperineurial glia cells. Figure created with BioRender.com.
Arachnid CPPs with currently unknown mechanism of membrane permeation are LVTX-8, WaTX, TRPA1, IPTxA and hadrucalcin. LVTX-8 is another spider venom CPP found to permeate cancer cell membranes, thereby showing potential for cancer-targeting delivery and therapy (Tan et al., 2017; Zhang et al., 2020). LVTX-8 sensitizes cancer cells by activating the mitochondrial death pathway and by up-regulating p27 to inhibit cell proliferation (Liu et al., 2012). All four reported scorpion CPPs are believed to possess cell permeating properties due to their receptor/channel binding sites being located intracellularly. The Wasabi receptor toxin WaTx was reported to penetrate the cell membrane to reach its intracellular binding site on TRPA1 channels (Lin King et al., 2019). Despite being an amphipathic peptide with a low content of cationic residues, evidence supports that WaTx penetrates cells via passive diffusion. The scorpion toxins imperatoxin A (IPTxA) from Pandinus imperator and maurocalcine from Scorpio palmatus share 83% sequence similarity and both activate the ryanodine receptors (RyRs) located in the endoplasmic membrane (Fajloun et al., 2000; Gurrola et al., 2010; Xiao et al., 2016). Hadrucalcin is another scorpion CPP that activates RyRs with high affinity (Schwartz et al., 2009).
Four arachnid CPPs are disulfide-rich ICK peptides and the other four are non-ICK peptides as shown in Figure 1. Apart from the amphipathic peptide LETX-VI (Figure 1D), all the other ICK peptides are cationic peptides with basic residues located on one face of the peptide (Figures 1A-C). Although the mechanism remains unknown, we presume that IPTxA, maurocalcine and hadrucalcin trigger the endocytosis uptake using their highly positively charged surface regions. The non-ICK peptides (Figures 1E-H) all contain high content of hydrophobic amino acids, forming an α-helix for binding and/or crossing membrane lipids.
3 The vertebrate blood-brain barrier
The vertebrate blood-brain barrier (BBB) is composed of endothelial cells which form the walls of the capillaries, surrounded by a second layer of the endothelial basement membrane and a third layer of the astrocytic glia limitans (Figure 2) (Abbott et al., 2010). The BBB plays a critical role in maintaining a stable fluid microenvironment for the vertebrate central nervous system (CNS) by protecting it from macromolecules and neurotoxic substances, by segregating neurotransmitters into separated pools, by regulating the concentration of specific ions and by facilitating the absorption of necessary nutrients and metabolites for nervous tissue (Abbott et al., 2010). Tight junctions significantly restrict the permeability of ions and polar solutes and block macromolecules from transversing via the paracellular diffusion pathway between endothelial cells from the blood to the brain fluid (Abbott et al., 2010). Lipid-mediated free diffusion is a common pathway for lipid soluble small molecule drugs with a molecular weight less than 400 Da to cross the BBB, while other water-soluble drugs may be transported within the BBB via carrier-mediated transport (CMT) system (Pardridge, 2012). In comparison, transcytosis via endocytic mechanisms provides the main route for proteins and peptides entering the CNS, which can be further divided into receptor-mediated transcytosis (RMT) and adsorptive-mediated transcytosis (AMT) (Abbott et al., 2010). In some particular pathological conditions, such as inflammation, the permeability of the BBB could be increased (Zhao et al., 2022).
3.1 Arachnid venom peptides crossing the vertebrate BBB
One spider and four scorpion venom peptides were reported to penetrate the vertebrate BBB. Chlorotoxin from Leiurus quinquestriatus quinquestriatus penetrates the BBB in tumor-bearing mice (Akcan et al., 2011; Cohen et al., 2018) and specifically binds to glioma cells (Annexin-2 and matrix metalloproteinase-2) and penetrates those cells by clathrin-mediated endocytosis (Wiranowska et al., 2011), thereby inhibiting their proliferation without affecting normal brain cells (Cohen et al., 2018; Deshane et al., 2003; Kesavan et al., 2010). Chlorotoxin is therefore more promising than using fluorescent 5-Aminolevulinic acid, which causes weaker tumour fluorescence and development of resistance (Ebrahimi et al., 2024). Besides, chlorotoxin is only toxic to small insects or invertebrates (i.e. cockroach and crayfish) (DeBin et al., 1993) while being safe for mammals, with its safety for human tumor therapy already being confirmed in clinical trials (Mamelak et al., 2006). HsTX1 from the scorpion Heterometrus spinnifer is a selective blocker of KV1.3, which is related to neurodegenerative diseases like Alzheimer’s and Parkinson’s disease. HsTX1 was reported to penetrate the mouse BBB under pathological conditions when tight junctions are disrupted, such as in Escherichia coli lipopolysaccharide-induced neuroinflammation (Reddiar et al., 2021). Therefore, HsTX1 was suggested for treating the above-mentioned diseases and secondary neuroinflammation via a novel pathway (Reddiar et al., 2021), although its therapeutic safety still needs to be assessed. In contrast, traditional neurotherapeutic drugs are delivered to the BBB via transporters, as exemplified in the CMT of L-3,4-dihydroxyphenylalanine for the treatment for Parkinson’s disease (Sweeney et al., 2018). However, vascular system changes in pathogenic regions during disease progression need to be considered, which could cause a disruption of normal drug distribution throughout the CNS, thereby preventing drugs from reaching their molecular targets (Sweeney et al., 2018). The scorpion peptides TsTX1 from Tityus serrulatus and AahII from Androctonus australis hector can penetrate the BBB, although only in newborn rodents and not at a later stage of brain development (Clot-Faybesse et al., 2000; Guidine et al., 2013; Nunan et al., 2004, 2003). However, this effect aligns with the observation that immature brains are commonly more sensitive to many chemicals and drugs (Schmitt et al., 2017). Furthermore, the therapeutic potential of both scorpion peptides is doubtful due to their reported toxicity to mammals. The spider toxin (δ-CNTX-Pn2a) from Phoneutria nigriventer was found to cross the rat BBB through both transcellular and paracellular routes, as evidenced by the pinocytic vesicles found in endothelial cells and tight junctions (de Paula Le Sueur et al., 2004, 2003). Microtubule-dependent vesicular transport was presumed to account for the Pn2a-induced increase in BBB permeability (de Paula Le Sueur et al., 2004), while increased release of the neurotransmitter L-glutamate in the CNS might be related to an abnormal expression of tight junctional proteins and other major components from brain capillary basement membranes, such as decreased expression of Zonula Occludens-1 and caveolin-1α, resulting in dysfunction of the BBB (Silva et al., 2018). In addition, arachnid venom peptides identified as therapeutic drug candidates need to be examined for possible immunogenicity, for example by employing T-cell or human leukocyte antigen binding assays (De Groot et al., 2023).
4 The invertebrate blood-brain barrier
Protection of neural tissue from disruption by toxins and other harmful substances forms the basis of the abundance and diversity of blood-brain barrier (BBB) types across different taxa (Dunton et al., 2021). While the traditional view is that the vertebrate BBB evolved from the invertebrate BBB, it is far more likely that these barriers developed convergently with different morphological structures and similar functions (Dunton et al., 2021). All four major arthropod subphyla (Hexapoda, Myriapoda, Chelicerata and Crustacea) have some form of BBB with further morphological disparity but generally containing the neural lamella, the perineurium, and the subperineurium (Figure 2) (Dunton et al., 2021). The neural lamella is a basement membrane of connective tissue, sitting between insect hemolymph and the perineurium (Dunton et al., 2021). The perineurium is a discontinuous layer of perineurial glia cells lined by gap junctions, which supports the neural lamella maintenance (Dunton et al., 2021). Overlayed by the perineurium, the subperineurium is formed by 4-6 sided polyploid subperineurial glia cells and their adjoining junctions (septate junctions, SJs) and functions as barrier, obstructing neurons from ions, molecules and polar solvents and blocking paracellular passage to neurons (Dunton et al., 2021; Limmer et al., 2014). The SJs can lead to increased resistance of insects against pesticides, as Drosophila larvae with higher expression of SJs showed higher resistance to insecticides from the Avermectin class (Chen et al., 2023).
The insect glia cells that are located between the subperineurium and neurons share many morphological and functional similarities with mammalian glia cells, albeit the neurons of insects and mammals are quite different (Spong et al., 2016). For example, insect neurons can withstand long periods of oxygen deprivation, allowing insects to survive adverse environmental conditions (Spong et al., 2016). Insect axons also lack myelination, although this does not apply to all other invertebrates, and the main excitatory transmitter of insect neurons is acetylcholine as compared to glutamate in vertebrates (Spong et al., 2016). The majority of arthropod BBB functional analysis was performed in the 1970’s and 80’s and largely focused on Drosophila (Dunton et al., 2021). However, Drosophila are somewhat unique in that pleated-sheet SJs are the dominant junction type and they lack tight junctions in their subperineurium (Dunton et al., 2021). Other Diptera, such as Musca (house fly) and Calliphora (blowfly) have both septate and tight junctions, while the lepidopteran Manduca sexta (hawkmoth) only have tight junctions (Dunton et al., 2021).
4.1 Arachnid venom peptides crossing the invertebrate BBB
The tight and septate junctions of the arthropod subperineurium function similar to tight junctions in vertebrate BBB by creating a selective permeability barrier (Dunton et al., 2021). This selective permeability barrier can be disrupted via K+/Na+-ATPase ion pumps, as reported for locust BBB (Spong et al., 2014). Using a high K+ solution or just direct disruption of these ion pumps can produce similar effects as environmental stressors like anoxia and hyper/hypothermia, namely paralysis or coma-like states that the locust recovers from once the stressor is removed (Spong et al., 2014). The drug Ouabain for example creates a build-up of extracellular K+ concentration by inhibition of Na/K-ATPase, resulting in spreading ionic disturbance and the associated reduction in neural activity in locusts (Spong et al., 2014).
Many arachnid toxins with activity in insects target the peripheral nervous system (PNS), with some acting on voltage-gated sodium (NaV) channels in insect neuromuscular junctions (Johnson et al., 1998). This attack on the PNS typically causes rapid contractile or flaccid paralysis already described in many toxicity assays (Johnson et al., 1998). However, there are also examples of arachnid toxins crossing the insect BBB to act on the insect CNS. For example, ω-HXTX-Hv1a (Hv1a) from the Blue Mountains funnel-web spider Hadronyche versuta acts 18-times quicker in transected Drosophila CNS preparations that exhibit a disrupted BBB (Bloomquist, 2003). U1-agatoxin-Ta1b (Ta1b) from the spider Tegenaria agrestis causes slow developing convulsions in lepidopteran and dipteran larvae leading to death by dehydration and starvation (Johnson et al., 1998). Using electrophysiology, it was shown that Ta1b has no effect on neuromuscular junctions or any other part of the PNS in housefly larvae (Johnson et al., 1998), but directly acted on the CNS, explaining the slow-developing toxin effects due to Ta1b having to first penetrate the larval BBB (Johnson et al., 1998).
Previous studies confirmed some conservation in the mechanisms underlying the vertebrate and invertebrate BBB. For example, the brain efflux activity in both vertebrate and invertebrate is mediated by ATP-binding cassette transporters (i.e. P-glycoprotein) (Al-Qadi et al., 2015; Hindle and Bainton, 2014). This implies that invertebrate BBB models might be useful for studying certain aspects of the vertebrate BBB, which could help in reducing the numbers of vertebrates required for these experiments. In addition, invertebrate BBB models could be used to study the BBB crossing abilities of arachnid venom peptides when assessing their potential for agricultural applications. Transcriptomic and proteomic analysis could further be employed for identifying commonalties between the vertebrate and the invertebrate BBB (Featherstone, 2011). Understanding differences in the BBB between vertebrates and invertebrates may provide clues for engineering drugs and insecticides with better taxa-selectivity, avoiding potential adverse effects on off-target organisms.
5 The insect midgut epithelium
The insect digestive tract consists of three regions: foregut, midgut, and hindgut, each with distinct features (Caccia et al., 2019; Li et al., 2018). The midgut, as shown in Figure 2, is comprised of two parts, gastric caeca and ventriculus, and is vital for digestion and nutrient absorption (Li et al., 2018). Gastric caeca are finger-like projections in the initial midgut section, serving as additional sites for digestion and absorption. Ventriculus is the primary digestion and absorption site, lined with columnar cells featuring apical microvilli for secretion and scattered goblet cells for pH regulation. Goblet cells are abundant in lepidopteran larvae midguts, maintaining an alkaline environment to neutralize plant toxins (Berenbaum, 1980; Pentzold et al., 2014). Besides, endocrine cells also intercalate in columnar cells, and are responsible for secreting bioactive peptides (Caccia et al., 2019). In some insects, the peritrophic matrix (PM) is present that serves as a non-cellular barrier surrounding the inner side of the midgut, protecting the epithelium from mechanical and chemical damage and pathogen infection and improving the absorption of diluted nutrients (Lehane, 1997). The midgut is also believed to be the major site for penetration by most insecticidal compounds and orally active arachnid venom peptides (Chapman, 1998).
5.1 Arachnid venom peptides crossing the midgut?
Usually, transport of peptides across the midgut barrier can be either via transcellular (i.e. via endocytosis) or paracellular (i.e. via the septate junctions) pathways (Herzig et al., 2014; Jeffers and Michael Roe, 2008). The transcellular pathway is represented by CPPs that interact with plasma membranes by non-disruptive endocytosis or by membrane disruptive pore-formation (Rádis-Baptista, 2021). Another possible route for venom peptides to cross the midgut epithelium is via septate junctions (SJs) that are the intercellular junctions between the epithelial cells found in invertebrates (Denecke et al., 2018) and that comprise the invertebrate analogue of tight junctions in vertebrates. Peptides with a molecular weight of up to 5 kDa are thought to be able to pass through SJs via paracellular diffusion (Zhu et al., 2001). Furthermore, linear peptides are more likely to pass than structured peptides, putting most disulfide-bridged venom peptides at a disadvantage (Denecke et al., 2018).
There are currently eight arachnid venom peptides known to induce oral insecticidal activity, suggesting their capacity of crossing the midgut epithelium (Tables 1 and 2). Unfortunately, detailed studies on the exact mechanism by which arachnid venom peptides cross biological membranes are rather limited (Denecke et al., 2018; Ponnappan and Chugh, 2017). LMX is a knotted peptide with four disulfide bonds that was optimized based on the scorpion neurotoxin LqhIT2 for protecting rice leaves from attack by the rice leaf folder (Tianpei et al., 2014). The difference in nine residues (K5R, R6K, D8N, V12I, A13S, D22N, A27G, Y28F and G30A) makes LMX much more potent compared to native LqhIT2 (Tianpei et al., 2014). Of the six spider venom peptides with reported oral insecticidal activity, lycotoxin-I from Lycosa carolinensis is the only linear peptide. Lycotoxin-I is a pore-forming peptide comprising amphipathic alpha-helices, and its variant (K24P, and L25W) targets insect pests like corn earworms (Helicoverpa zea) and tobacco beetles (Lasioderma serricorne) (Johnson et al., 2014). The other 5 orally insecticidal spider peptides are all knotted peptides with 3-4 disulfide bonds. Hv1a was the first spider venom peptide with reported oral activity, targeting different arthropods like lone star ticks, fruit flies and sheep blowflies (Guo et al., 2018; Mukherjee et al., 2006). The most potent orally insecticidal spider peptide known to date is β-DGTX-Dc1a targeting both fruit flies and sheep blowflies (Guo et al., 2018). U1-TRTX-Sp1a (Sp1a) from the spider Selenotypus plumipes and JFTX-23 from the spider Selenocosmia jiafu both exhibit oral insecticidal activity against cotton bollworm (Helicoverpa armigera), while Sp1a was also orally active in mealworms (Hardy et al., 2013; Monfared et al., 2022). Spear®-T is the first commercialized spider peptide bioinsecticide containing the active ingredient GS-ω/κ-HXTX-Hv1a from Hadronyche versuta venom, which is used for the control of greenhouse pests such as aphids and spider mites (Sukiran et al., 2023). Another linear arachnid venom peptide (checacin 1) that was orally insecticidal against Acyrthosiphon pisum aphids was recently reported from the pseudoscorpion Chelifer cancroides (Krämer et al., 2022).
6 Alternative route for venom peptides reaching the hemolymph
Spiracles in the insect integument have been reported as an alternative route for insecticidal compounds to reach the insect hemolymph and CNS (Sugiura et al., 2008; Sumita et al., 2016). Spiracles are the external openings of the trachea located in the insect exoskeleton and thereby provide a means for entry into the insect respiration system (Figure 3). For peptides to penetrate the spiracles, they first need to cross a filter apparatus composed of cuticular setae that prevents the entry of dust and the loss of water. This is followed by the atrium and the delicate valve lids protected by the cuticular frame (Wasserthal and Fröhlich, 2017). The valve lids are lateral folds extending from the exterior tracheal wall, connecting a larger tracheal space (vestibulum) which then further splits up into a dorsal and a ventral tracheal trunk, a network of tracheoles and eventually terminal tracheoles, where the aerosol gets into contact with the hemolymph (Burrows, 1980; Hayashi and Kondo, 2018; Wasserthal and Fröhlich, 2017). The mesothoracic spiracles are likely the primary entry sites for some insecticides such as pyrethroids, as it provides the quickest route for insecticides to the CNS (Sumita et al., 2016). The commercialized spider venom peptide insecticide Spear®-T was designed as a contact foliar spray and is presumed to enter the insects through their respiratory system (King, 2019; Sukiran et al., 2023).
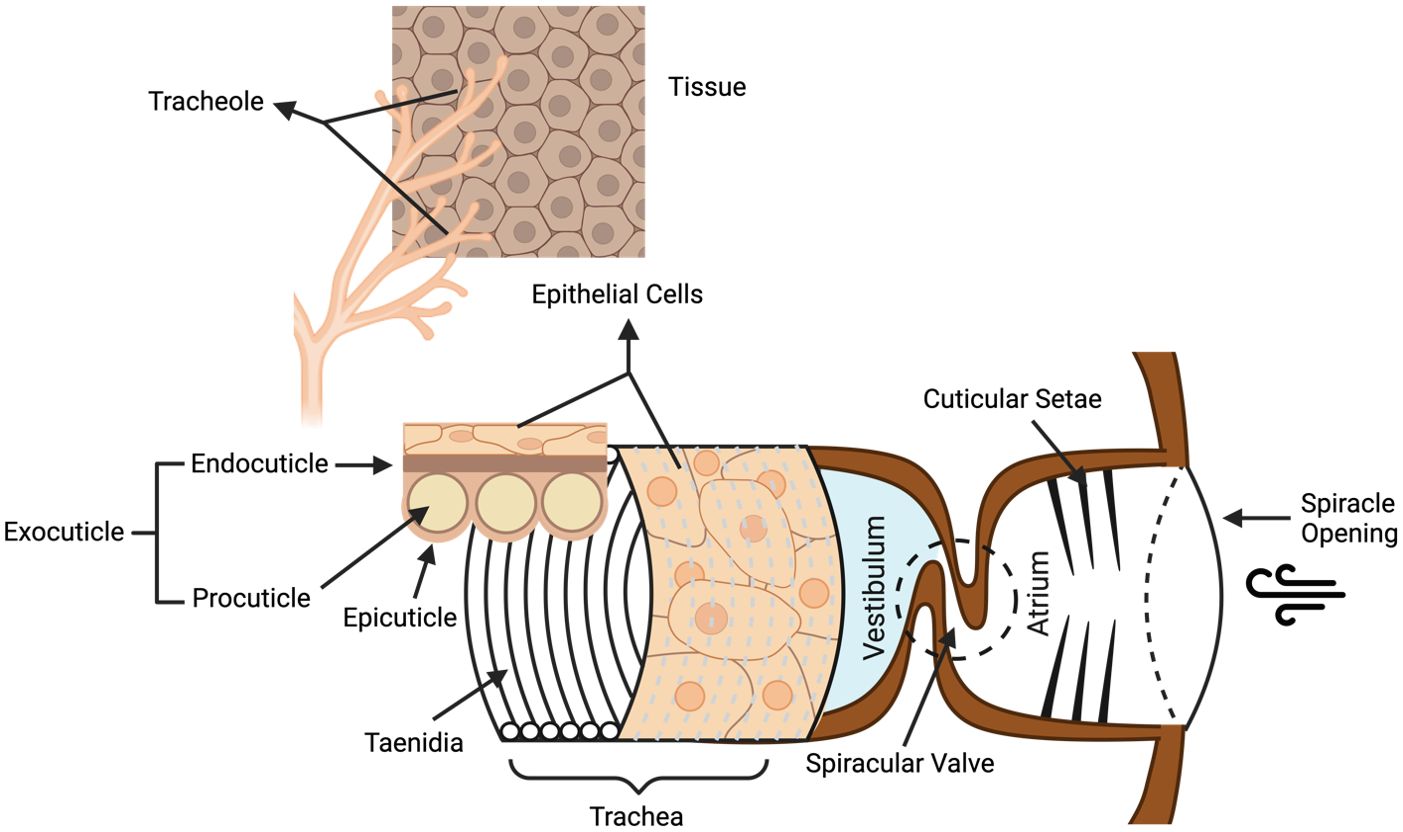
Figure 3. Structure of the insect respiratory system. Venom peptides are presumed to enter the insect respiratory tract via the spiracle openings, then crossing the cuticular setae filter and the spiracular valve to enter the atrium. From there, venom peptides might then penetrate the epithelial barriers of the trachea and tracheoles to reach the hemolymph. Taenidia are the ring structures forming the interior tracheal wall and protect the trachea from collapse when the internal pressure is reduced. Figure modified from previous references (Wasserthal and Fröhlich, 2017; Webster et al., 2015) and according to the website https://cronodon.com/BioTech/insect_respiration.html. Figure created with BioRender.com.
7 Physico-chemical characteristics of membrane-permeating peptides
CPPs usually consist of positively charged amino acids, such as lysine (K) and arginine (R), and have a considerable level of amphipathicity and cationicity, which provides CPPs with high affinity for the negatively charged lipid membranes (Rádis-Baptista, 2021). Previous research has demonstrated that cell surface binding for arginine-rich CPPs is more efficient than for lysine-rich CPPs (Amand et al., 2008, 2012; Pan et al., 2021a). Additionally, Chen et.al, found that arginine-rich peptides are able to penetrate insect cell membranes efficiently (Chen et al., 2012). However, although arginine residues dominate in most cationic non-arachnid CPPs, arachnid venom CPPs on the other hand preferentially utilize lysine over arginine residues (Madani et al., 2011). Additionally, most arachnid-derived BBB penetrating peptides and midgut penetrating peptides also contain more lysine than arginine residues, with only a few exceptions such as the scorpion peptide chlorotoxin, and the spider peptides Sp1a, JFTX- 23 and GS‐ω/κ‐HXTX‐Hv1a. Overall, lysine plays a dominant role in 18 of the 23 membrane-crossing arachnid peptides listed in Table 1. The underlying reason for the prevalence of lysine in these peptides, however, remains unclear. One potential explanation is that, although the absorption of arginine-rich peptides is higher than that of lysine-rich peptides during endocytosis, lysine-rich peptides are more potent in causing liposome leakage. This increased leakage allows lysine-rich peptides to more easily reach the hemolymph compared to arginine-rich peptides (Strömstedt et al., 2009). Additionally, lysine residues may contribute to the toxicity of arachnid venom peptides. For example, lysine substitutions can enhance the activity of scorpion antimicrobial venom peptides, which are the critical defensive peptides in host innate immunity (Li et al., 2022). Another possible explanation could be due to the differences in composition of invertebrate vs. vertebrate membranes (Alberts B and Lewis, 2002; Marheineke et al., 1998), which lysine or arginine being more effective in either one or the other type of membrane.
Besides the distribution of positively charged amino acids, the unique disulfide-rich secondary structure is also believed to contribute to the activity of the venom neurotoxins, providing toxins with extraordinary thermal and chemical stability (Herzig and King, 2015). All the BBB-penetrating peptides in Table 1 are disulfide-rich, providing them with high enzymatic stability in vertebrate serum and improving their half-life in the CNS (Saez et al., 2010). Nevertheless, 3 of 8 CPPs and 2 of 8 orally active peptides (Table 1) are linear peptides with no disulfide bonds. Unfortunately, in vitro experiments to determine the membrane-permeating ability of venom peptides are generally conducted in the absence of proteases, renal clearance or off-target binding. It therefore remains to be determined whether the membrane-permeating peptides listed in Table 1 are also suitable in vivo. For orally active peptides, stability towards enzymatic degradation is crucial because they are required to survive for a sufficient time in both insect midgut and hemolymph. Surprisingly, two linear orally active peptides, lycotoxin I variant and checacin1 exhibited moderate oral insecticidal activity. Both linear orally active peptides not only show insecticidal activity but also antimicrobial/cytotoxic activity as pore-forming peptides (Krämer et al., 2022; Yan and Adams, 1998). Therefore, it is possible that the oral activity of the lycotoxin I variant and checacin1 is due to their pore-forming ability in midgut epithelial cells. Apart from these two linear pore-forming peptides, the other six orally activity peptides are all disulfide-rich (five of them being ICK peptides).
8 Methods for increasing membrane-permeability of peptides
High content of lysine and arginine are crucial for enhancing the cell membrane permeability of cationic CPPs. Therefore, poly-lysine or poly-arginine tags have been suggested as carrier vectors to facilitate the permeability of cargo through the cell membrane (Chen et al., 2012; Pan et al., 2021a; Yaroslavov et al., 2003).
To enhance midgut penetration rate of the arachnid venom peptides, delivery vectors with well-known membrane penetration mechanisms have been used such as carrier proteins (i.e. GNA) (Fitches et al., 2004) and insect-specific entomopathogens (e.g. viruses: luteoviruses or baculoviruses; fungi: Beauveria or Metarhizium) (Bonning et al., 2014; Rajput et al., 2023). The big advantage of insect-specific entomopathogens is their phyletic selectivity which limits their effects to the targeted pests (e.g. the fungus Metarhizium acridum is selective for grasshoppers of the family Acrididae) (Herzig et al., 2014; Lomer et al., 2001). Co-application of venom peptides with Cry toxins isolated from the bacteria Bacillus thuringiensis (Bt) is also commonly used to enhance the oral toxicity, as Cry toxins can directly damage the lining of the gut and form pores, which helps venom peptides to pass through (Pan et al., 2021b). Genetically modified crops expressing Cry toxins have been globally introduced (Gassmann and Hutchison, 2012), and pyramiding of arachnid venom peptides with Cry toxins could be another option delivering peptides into the hemolymph (Moar and Anilkumar, 2007). Nanoformulation provides a new approach for peptide delivery (Wei et al., 2022), as well as a novel nano-vehicles containing only viral coat protein without genetical materials, which is considered safer for transporting insecticidal peptides compared to the intact virus (Xue et al., 2024).
The BBB permeability can also be enhanced by using different delivery vectors, such as cationized albumin that reaches the brain via adsorptive-mediated endocytosis and mAb OX26 that recognizes transferrin receptors expressed on brain capillaries (Temsamani et al., 2001). Nanoparticles, such as liposomes are also considered an attractive vector because of their unique physicochemical characteristics compatible with hydrophilic, lipophilic, and hydrophobic therapeutic agents for delivery across the BBB (Lai et al., 2013). Cell-penetrating peptides (i.e. R11, TD, TAT, CTP and LNP) can also be employed as carriers via co-engineering with venom peptides for crossing the BBB (Li et al., 2017; Yao et al., 2015; Zou et al., 2013).
9 Conclusions
The multifaceted potential applications of arachnid venom peptides in pharmacology and agronomy underscore the importance of understanding both their ability and the underlying mechanisms for crossing biological barriers, including cell membranes, the midgut and respiratory epithelium and the BBB. Evidence suggests that cationic arachnid venom peptides permeate cell membranes via the endocytosis pathway. Within the midgut epithelium, venom peptides may utilize either transcellular pathways via endocytosis or paracellular pathways via septate junctions. Similarly, in crossing the BBB, arachnid venom peptides demonstrate diverse mechanisms, including transcytosis via endocytosis pathways. Notably, lysine and arginine residues appear to play significant roles in membrane interactions, with lysine-rich peptides being predominant in arachnid peptides penetrating cell membranes, the midgut epithelium and the BBB. For peptides with no inherent membrane-permeating capabilities, utilizing delivery vectors, such as nanoparticles, entomopathogens or cell-penetrating peptides presents promising strategies to enhance their membrane permeability. Overall, elucidating the intricate mechanisms of arachnid venom peptides in penetrating lipid bilayer membranes not only enhances our understanding of venom biology, but also holds immense promise for the development of novel therapeutic drugs and bioinsecticides.
Author contributions
YW: Writing – original draft. SG: Writing – original draft. KJ: Writing – original draft. VH: Conceptualization, Funding acquisition, Supervision, Writing – review & editing.
Funding
The author(s) declare that financial support was received for the research, authorship, and/or publication of this article. VH was funded by the Australian Research Council (FT190100482).
Conflict of interest
The authors declare that the research was conducted in the absence of any commercial or financial relationships that could be construed as a potential conflict of interest.
The author(s) declared that they were an editorial board member of Frontiers, at the time of submission. This had no impact on the peer review process and the final decision.
Publisher’s note
All claims expressed in this article are solely those of the authors and do not necessarily represent those of their affiliated organizations, or those of the publisher, the editors and the reviewers. Any product that may be evaluated in this article, or claim that may be made by its manufacturer, is not guaranteed or endorsed by the publisher.
References
Abbott N. J., Patabendige A. A., Dolman D. E., Yusof S. R., Begley D. J. (2010). Structure and function of the blood-brain barrier. Neurobiol. Dis. 37, 13–25. doi: 10.1016/j.nbd.2009.07.030
Akcan M., Stroud M. R., Hansen S. J., Clark R. J., Daly N. L., Craik D. J., et al. (2011). Chemical re-engineering of chlorotoxin improves bioconjugation properties for tumor imaging and targeted therapy. J. Med. Chem. 54, 782–787. doi: 10.1021/jm101018r
Alberts B J. A., Lewis J. (2002). The lipid bilayer, Molecular Biology of the Cell, 4th ed (New York: Garland Science).
Al-Qadi S., Schiøtt M., Hansen S. H., Nielsen P. A., Badolo L. (2015). An invertebrate model for CNS drug discovery: Transcriptomic and functional analysis of a mammalian P-glycoprotein ortholog. Biochim. Biophys. Acta 1850, 2439–2451. doi: 10.1016/j.bbagen.2015.09.002
Amand H. L., Fant K., Nordén B., Esbjörner E. K. (2008). Stimulated endocytosis in penetratin uptake: effect of arginine and lysine. Biochem. Biophys. Res. Commun. 371, 621–625. doi: 10.1016/j.bbrc.2008.04.039
Amand H. L., Rydberg H. A., Fornander L. H., Lincoln P., Nordén B., Esbjörner E. K. (2012). Cell surface binding and uptake of arginine- and lysine-rich penetratin peptides in absence and presence of proteoglycans. Biochim. Biophys. Acta 1818, 2669–2678. doi: 10.1016/j.bbamem.2012.06.006
Berenbaum M. (1980). Adaptive significance of midgut pH in larval lepidoptera. Am. Nat. 115, 138–146. doi: 10.1086/283551
Bloomquist J. R. (2003). Mode of action of atracotoxin at central and peripheral synapses of insects. Invert Neurosci. 5, 45–50. doi: 10.1007/s10158-003-0027-z
Bonning B. C., Pal N., Liu S., Wang Z., Sivakumar S., Dixon P. M., et al. (2014). Toxin delivery by the coat protein of an aphid-vectored plant virus provides plant resistance to aphids. Nat. Biotechnol. 32, 102–105. doi: 10.1038/nbt.2753
Burrows M. (1980). The tracheal supply to the central nervous system of the locust. Proc. R Soc. B 207, 63–78. doi: 10.1098/rspb.1980.0014
Caccia S., Casartelli M., Tettamanti G. (2019). The amazing complexity of insect midgut cells: types, peculiarities, and functions. Cell Tissue Res. 377, 505–525. doi: 10.1007/s00441-019-03076-w
Chapman R. F. (1998). “Alimentary canal, digestion and absorption,” in The insects: structure and function, 4. Ed. Chapman R. F. (Cambridge University Press, Cambridge), 38–68.
Chen L. P., Jiang H. Q., Luo L., Qiu J., Xing X. J., Hou R. Y., et al. (2023). The role of intercellular junction proteins in the penetration resistance of Drosophila larvae to avermectin. Comp. Biochem. Physiol. C 266, 109557. doi: 10.1016/j.cbpc.2023.109557
Chen Y. J., Liu B. R., Dai Y. H., Lee C. Y., Chan M. H., Chen H. H., et al. (2012). A gene delivery system for insect cells mediated by arginine-rich cell-penetrating peptides. Gene 493, 201–210. doi: 10.1016/j.gene.2011.11.060
Clot-Faybesse O., Guieu R., Rochat H., Devaux C. (2000). Toxicity during early development of the mouse nervous system of a scorpion neurotoxin active on sodium channels. Life Sci. 66, 185–192. doi: 10.1016/S0024-3205(99)00579-2
Cockcroft S. (2021). Mammalian lipids: structure, synthesis and function. Essays Biochem. 65, 813–845. doi: 10.1042/EBC20200067
Cohen G., Burks S. R., Frank J. A. (2018). Chlorotoxin-A multimodal imaging platform for targeting glioma tumors. Toxins 10, 496. doi: 10.3390/toxins10120496
DeBin J. A., Maggio J. E., Strichartz G. R. (1993). Purification and characterization of chlorotoxin, a chloride channel ligand from the venom of the scorpion. Am. J. Physiol. 264, C361–C369. doi: 10.1152/ajpcell.1993.264.2.C361
De Groot A. S., Roberts B. J., Mattei A., Lelias S., Boyle C., Martin W. D. (2023). Immunogenicity risk assessment of synthetic peptide drugs and their impurities. Drug Discovery Today 28, 103714. doi: 10.1016/j.drudis.2023.103714
De Lima M. E., Figueiredo S. G., Pimenta A. M. C., Santos D. M., Borges M. H., Cordeiro M. N., et al. (2007). Peptides of arachnid venoms with insecticidal activity targeting sodium channels. Comp. Biochem. Physiol. Part - C: Toxicol. Pharmacol. 146, 264–279. doi: 10.1016/j.cbpc.2006.10.010
Denecke S., Swevers L., Douris V., Vontas J. (2018). How do oral insecticidal compounds cross the insect midgut epithelium? Insect Biochem. Mol. Biol. 103, 22–35. doi: 10.1016/j.ibmb.2018.10.005
de Paula Le Sueur L., Collares-Buzato C. B., da Cruz-Höfling M. A. (2004). Mechanisms involved in the blood-brain barrier increased permeability induced by Phoneutria nigriventer spider venom in rats. Brain Res. 1027, 38–47. doi: 10.1016/j.brainres.2004.08.055
de Paula Le Sueur L., Kalapothakis E., da Cruz-Höfling M. A. (2003). Breakdown of the blood-brain barrier and neuropathological changes induced by Phoneutria nigriventer spider venom. Acta Neuropathol. 105, 125–134. doi: 10.1007/s00401-002-0623-8
Deshane J., Garner C. C., Sontheimer H. (2003). Chlorotoxin inhibits glioma cell invasion via matrix metalloproteinase-2. J. Biol. Chem. 278, 4135–4144. doi: 10.1074/jbc.M205662200
Deshayes S., Morris M. C., Divita G., Heitz F. (2006). Interactions of amphipathic CPPs with model membranes. Biochim. Biophys. Acta 1758, 328–335. doi: 10.1016/j.bbamem.2005.10.004
Duchardt F., Fotin-Mleczek M., Schwarz H., Fischer R., Brock R. (2007). A comprehensive model for the cellular uptake of cationic cell-penetrating peptides. Traffic 8, 848–866. doi: 10.1111/j.1600-0854.2007.00572.x
Dunton A. D., Göpel T., Ho D. H., Burggren W. (2021). Form and function of the vertebrate and invertebrate blood-brain barriers. Int. J. Mol. Sci. 22, 12111. doi: 10.3390/ijms222212111
Ebrahimi S., Khaleghi Ghadiri M., Stummer W., Gorji A. (2024). Enhancing 5-ALA-PDT efficacy against resistant tumor cells: Strategies and advances. Life Sci. 351, 122808. doi: 10.1016/j.lfs.2024.122808
El-Andaloussi S., Holm T., Langel U. (2005). Cell-penetrating peptides: mechanisms and applications. Curr. Pharm. Des. 11, 3597–3611. doi: 10.2174/138161205774580796
Fajloun Z., Kharrat R., Chen L., Lecomte C., Di Luccio E., Bichet D., et al. (2000). Chemical synthesis and characterization of maurocalcine, a scorpion toxin that activates Ca2+ release channel/ryanodine receptors. FEBS Lett. 469, 179–185. doi: 10.1016/S0014-5793(00)01239-4
Featherstone D. E. (2011). Glial solute carrier transporters in drosophila and mice. Glia 59, 1351–1363. doi: 10.1002/glia.21085
Fitches E., Audsley N., Gatehouse J. A., Edwards J. P. (2002). Fusion proteins containing neuropeptides as novel insect contol agents: snowdrop lectin delivers fused allatostatin to insect haemolymph following oral ingestion. Insect Biochem. Mol. Biol. 32, 1653–1661. doi: 10.1016/S0965-1748(02)00105-4
Fitches E., Edwards M. G., Mee C., Grishin E., Gatehouse A. M. R., Edwards J. P., et al. (2004). Fusion proteins containing insect-specific toxins as pest control agents: snowdrop lectin delivers fused insecticidal spider venom toxin to insect haemolymph following oral ingestion. J. Insect Physiol. 50, 61–71. doi: 10.1016/j.jinsphys.2003.09.010
Fitches E., Gatehouse J. A. (1998). A comparison of the short and long term effects of insecticidal lectins on the activities of soluble and brush border enzymes of tomato moth larvae (Lacanobia oleracea). J. Insect Physiol. 44, 1213–1224. doi: 10.1016/S0022-1910(98)00090-0
Fitches E., Woodhouse S. D., Edwards J. P., Gatehouse J. A. (2001). In vitro and in vivo binding of snowdrop (Galanthus nivalis agglutinin; GNA) and jackbean (Canavalia ensiformis; Con A) lectins within tomato moth (Lacanobia oleracea) larvae; mechanisms of insecticidal action. J. Insect Physiol. 47, 777–787. doi: 10.1016/S0022-1910(01)00068-3
Frankel A. D., Pabo C. O. (1988). Cellular uptake of the tat protein from human immunodeficiency virus. Cell 55, 1189–1193. doi: 10.1016/0092-8674(88)90263-2
Fu A., Wang Y., Zhan L., Zhou R. (2012). Targeted delivery of proteins into the central nervous system mediated by rabies virus glycoprotein-derived peptide. Pharm. Res. 29, 1562–1569. doi: 10.1007/s11095-012-0667-y
Gassmann A. J., Hutchison W. D. (2012). Bt crops and insect pests. GM Crops Food 3, 139–139. doi: 10.4161/gmcr.21778
Gatehouse A. M., Gatehouse J. A., Bharathi M., Spence J., Powell K. S. (1998). Immunohistochemical and developmental studies to elucidate the mechanism of action of the snowdrop lectin on the rice brown planthopper, Nilaparvata lugens (Stal). J. Insect Physiol. 44, 529–539. doi: 10.1016/S0022-1910(98)00054-7
Green M., Ishino M., Loewenstein P. M. (1989). Mutational analysis of HIV-1 Tat minimal domain peptides: identification of trans-dominant mutants that suppress HIV-LTR-driven gene expression. Cell 58, 215–223. doi: 10.1016/0092-8674(89)90417-0
Guidine P. A. M., Cash D., Drumond L. E., de Souza e Rezende G. H., Massensini A. R., Williams S. C. R., et al. (2013). Brainstem structures are primarily affected in an experimental model of severe scorpion envenomation. Toxicol. Sci. 137, 147–157. doi: 10.1093/toxsci/kft231
Guo S., Herzig V., King G. F. (2018). Dipteran toxicity assays for determining the oral insecticidal activity of venoms and toxins. Toxicon 150, 297–303. doi: 10.1016/j.toxicon.2018.06.077
Gurrola G. B., Capes E. M., Zamudio F. Z., Possani L. D., Valdivia H. H. (2010). Imperatoxin A, a cell-penetrating peptide from scorpion venom, as a probe of Ca-release channels/ryanodine receptors. Pharmaceuticals 3, 1093–1107. doi: 10.3390/ph3041093
Harayama T., Riezman H. (2018). Understanding the diversity of membrane lipid composition. Nat. Rev. Mol. Cell Biol. 19, 281–296. doi: 10.1038/nrm.2017.138
Hardy M. C., Daly N. L., Mobli M., Morales R. A. V., King G. F. (2013). Isolation of an orally active insecticidal toxin from the venom of an Australian tarantula. PloS One 8, e73136–e73136. doi: 10.1371/journal.pone.0073136
Hayashi S., Kondo T. (2018). Development and function of the Drosophila tracheal system. Genetics 209, 367–380. doi: 10.1534/genetics.117.300167
Herzig V., Bende N. S., Alam M. S., Tedford H. W., Kennedy R. M., King G. F. (2014). “Methods for deployment of spider venom peptides as bioinsecticides,” in Advances in insect physiology. Eds. Dhadialla T. S., Gill S. S. (Cambridge, Massachusetts, USA: Academic Press), 389–411.
Herzig V., de Araujo A. D., Greenwood K. P., Chin Y. K., Windley M. J., Chong Y., et al. (2018). Evaluation of chemical strategies for improving the stability and oral toxicity of insecticidal peptides. Biomedicines 6, 90. doi: 10.3390/biomedicines6030090
Herzig V., King G. F. (2015). The cystine knot is responsible for the exceptional stability of the insecticidal spider toxin ω-Hexatoxin-Hv1a. Toxins 7, 4366–4380. doi: 10.3390/toxins7104366
Hindle S. J., Bainton R. J. (2014). Barrier mechanisms in the Drosophila blood-brain barrier. Front. Neurosci. 8, 414. doi: 10.3389/fnins.2014.00414
Islam M. Z., Sharmin S., Moniruzzaman M., Yamazaki M. (2018). Elementary processes for the entry of cell-penetrating peptides into lipid bilayer vesicles and bacterial cells. Appl. Microbiol. Biotechnol. 102, 3879–3892. doi: 10.1007/s00253-018-8889-5
Jeffers L. A., Michael Roe R. (2008). The movement of proteins across the insect and tick digestive system. J. Insect Physiol. 54, 319–332. doi: 10.1016/j.jinsphys.2007.10.009
Jeffers L. A., Thompson D. M., Ben-Yakir D., Roe R. M. (2005). Movement of proteins across the digestive system of the tobacco budworm, Heliothis virescens. Entomol Exp. Appl. 117, 135–146. doi: 10.1111/j.1570-7458.2005.00342.x
Jiao C. Y., Delaroche D., Burlina F., Alves I. D., Chassaing G., Sagan S. (2009). Translocation and endocytosis for cell-penetrating peptide internalization. J. Biol. Chem. 284, 33957–33965. doi: 10.1074/jbc.M109.056309
Johnson J. H., Bloomquist J. R., Krapcho K. J., Kral R. M. Jr., Trovato R., Eppler K. G., et al. (1998). Novel insecticidal peptides from Tegenaria agrestis spider venom may have a direct effect on the insect central nervous system. Arch. Insect Biochem. Physiol. 38, 19–31. doi: 10.1002/(SICI)1520-6327(1998)38:1<19::AID-ARCH3>3.0.CO;2-Q
Johnson E. T., Dowd P. F., Hughes S. R. (2014). Expression of a wolf spider toxin in tobacco inhibits the growth of microbes and insects. Biotechnol. Lett. 36, 1735–1742. doi: 10.1007/s10529-014-1536-z
Jumper J., Evans R., Pritzel A., Green T., Figurnov M., Ronneberger O., et al. (2021). Highly accurate protein structure prediction with AlphaFold. Nature 596, 583–589. doi: 10.1038/s41586-021-03819-2
Kesavan K., Ratliff J., Johnson E. W., Dahlberg W., Asara J. M., Misra P., et al. (2010). Annexin A2 is a molecular target for TM601, a peptide with tumor-targeting and anti-angiogenic effects. J. Biol. Chem. 285, 4366–4374. doi: 10.1074/jbc.M109.066092
King G. F. (2019). Tying pest insects in knots: the deployment of spider-venom-derived knottins as bioinsecticides. Pest Manag Sci. 75, 2437–2445. doi: 10.1002/ps.v75.9
Krämer J., Lüddecke T., Marner M., Maiworm E., Eichberg J., Hardes K., et al. (2022). Antimicrobial, insecticidal and cytotoxic activity of linear venom peptides from the pseudoscorpion Chelifer cancroides. Toxins 14, 58. doi: 10.3390/toxins14010058
Lai F., Fadda A. M., Sinico C. (2013). Liposomes for brain delivery. Expert Opin. Drug Delivery 10, 1003–1022. doi: 10.1517/17425247.2013.766714
Lehane M. J. (1997). Peritrophic matrix structure and function. Annu. Rev. Entomol 42, 525–550. doi: 10.1146/annurev.ento.42.1.525
Li S., Shui Y., Ma J., Yuan Y., Jiang W., Xu C., et al. (2022). Antimicrobial activity of CT-K3K7, a modified peptide by lysine substitutions from ctry2459 - A Chaerilus tryznai scorpion venom peptide. Toxicon 218, 88–98. doi: 10.1016/j.toxicon.2022.09.004
Li Q., Xu M., Cui Y., Huang C., Sun M. (2017). Arginine-rich membrane-permeable peptides are seriously toxic. Pharmacol. Res. Perspect. 5, 1–5. doi: 10.1002/prp2.2017.5.issue-5
Li K., Zhang J. H., Yang Y. J., Han W., Yin H. (2018). Morphology and fine organization of the midgut of Gampsocleis gratiosa (Orthoptera: Tettigoniidae). PloS One 13, e0200405. doi: 10.1371/journal.pone.0200405
Limmer S., Weiler A., Volkenhoff A., Babatz F., Klämbt C. (2014). The Drosophila blood-brain barrier: development and function of a glial endothelium. Front. Neurosci. 8, 365. doi: 10.3389/fnins.2014.00365
Lin King J. V., Emrick J. J., Kelly M. J. S., Herzig V., King G. F., Medzihradszky K. F., et al. (2019). A cell-penetrating scorpion toxin enables mode-specific modulation of TRPA1 and pain. Cell 178, 1362–1374.e1316. doi: 10.1016/j.cell.2019.07.014
Liu Z., Deng M., Xiang J., Ma H., Hu W., Zhao Y., et al. (2012). A novel spider peptide toxin suppresses tumor growth through dual signaling pathways. Curr. Mol. Med. 12, 1350–1360. doi: 10.2174/156652412803833643
Lomer C. J., Bateman R. P., Johnson D. L., Langewald J., Thomas M. (2001). Biological control of locusts and grasshoppers. Annu. Rev. Entomol 46, 667–702. doi: 10.1146/annurev.ento.46.1.667
Lüddecke T., Herzig V., von Reumont B. M., Vilcinskas A. (2022). The biology and evolution of spider venoms. Biol. Rev. 97, 163–178. doi: 10.1111/brv.12793
Madani F., Lindberg S., Langel U., Futaki S., Gräslund A. (2011). Mechanisms of cellular uptake of cell-penetrating peptides. J. Biophys. 2011, 414729. doi: 10.1155/2011/414729
Magzoub M., Pramanik A., Gräslund A. (2005). Modeling the endosomal escape of cell-penetrating peptides: transmembrane pH gradient driven translocation across phospholipid bilayers. Biochemistry 44, 14890–14897. doi: 10.1021/bi051356w
Mamelak A. N., Rosenfeld S., Bucholz R., Raubitschek A., Nabors L. B., Fiveash J. B., et al. (2006). Phase I single-dose study of intracavitary-administered iodine-131-TM-601 in adults with recurrent high-grade glioma. J. Clin. Oncol. 24, 3644–3650. doi: 10.1200/JCO.2005.05.4569
Marheineke K., Grünewald S., Christie W., Reiländer H. (1998). Lipid composition of Spodoptera frugiperda (Sf9) and Trichoplusia ni (Tn) insect cells used for baculovirus infection. FEBS Lett. 441, 49–52. doi: 10.1016/S0014-5793(98)01523-3
Moar W. J., Anilkumar K. J. (2007). The power of the pyramid. Science 318, 1561–1562. doi: 10.1126/science.1151313
Monfared N., Ahadiyat A., Fathipour Y., Mianroodi R. A. (2022). Evaluation of recombinant toxin JFTX-23, an oral-effective anti-insect peptide from the spider Selenocosmia jiafu venom gland proteome. Toxicon 217, 78–86. doi: 10.1016/j.toxicon.2022.08.003
Mukherjee A. K., Sollod B. L., Wikel S. K., King G. F. (2006). Orally active acaricidal peptide toxins from spider venom. Toxicon 47, 182–187. doi: 10.1016/j.toxicon.2005.10.011
Nakase I., Niwa M., Takeuchi T., Sonomura K., Kawabata N., Koike Y., et al. (2004). Cellular uptake of arginine-rich peptides: roles for macropinocytosis and actin rearrangement. Mol. Ther. 10, 1011–1022. doi: 10.1016/j.ymthe.2004.08.010
Nunan E. A., Arya V., Hochhaus G., Cardoso V. N., Moraes-Santos T. (2004). Age effects on the pharmacokinetics of tityustoxin from Tityus serrulatus scorpion venom in rats. Braz. J. Med. Biol. Res. 37, 385–390. doi: 10.1590/S0100-879X2004000300016
Nunan E. A., Moraes M. F., Cardoso V. N., Moraes-Santos T. (2003). Effect of age on body distribution of Tityustoxin from Tityus serrulatus scorpion venom in rats. Life Sci. 73, 319–325. doi: 10.1016/S0024-3205(03)00264-9
Pallaghy P. K., Nielsen K. J., Craik D. J., Norton R. S. (1994). A common structural motif incorporating a cystine knot and a triple-stranded beta-sheet in toxic and inhibitory polypeptides. Protein Sci. 3, 1833–1839. doi: 10.1002/pro.5560031022
Pan Y. C., Barba-Bon A., Tian H. W., Ding F., Hennig A., Nau W. M., et al. (2021a). An amphiphilic sulfonatocalix[5]arene as an activator for membrane transport of lysine-rich peptides and proteins. Angew Chem. Int. Ed 60, 1875–1882. doi: 10.1002/anie.202011185
Pan Z.-Z., Xu L., Liu B., Chen Q.-X., Zhu Y.-J. (2021b). Key residues of Bacillus thuringiensis Cry2Ab for oligomerization and pore-formation activity. AMB Express 11, 112–112. doi: 10.1186/s13568-021-01270-0
Pardridge W. M. (2012). Drug transport across the blood-brain barrier. J. Cereb Blood Flow Metab. 32, 1959–1972. doi: 10.1038/jcbfm.2012.126
Pentzold S., Zagrobelny M., Roelsgaard P. S., Møller B. L., Bak S. (2014). The multiple strategies of an insect herbivore to overcome plant cyanogenic glucoside defence. PloS One 9, e91337. doi: 10.1371/journal.pone.0091337
Ponnappan N., Chugh A. (2017). Cell-penetrating and cargo-delivery ability of a spider toxin-derived peptide in mammalian cells. Eur. J. Pharm. Biopharm 114, 145–153. doi: 10.1016/j.ejpb.2017.01.012
Rádis-Baptista G. (2021). Cell-penetrating peptides derived from animal venoms and toxins. Toxins 13, 147. doi: 10.3390/toxins13020147
Rajput S., Suroshe S. S., Yadav P. R., Kumar A., Saini G. K. (2023). Bioefficacy of engineered Beauveria bassiana with scorpion neurotoxin, LqqIT1 against cotton mealybug, Phenacoccus solenopsis and cowpea aphid, Aphis craccivora. PeerJ 11, e16030. doi: 10.7717/peerj.16030
Reddiar S. B., Jin L., Wai D. C. C., Csoti A., Panyi G., Norton R. S., et al. (2021). Lipopolysaccharide influences the plasma and brain pharmacokinetics of subcutaneously-administered HsTX1[R14A], a KV1.3-blocking peptide. Toxicon 195, 29–36. doi: 10.1016/j.toxicon.2021.03.002
Saez N. J., Herzig V. (2019). Versatile spider venom peptides and their medical and agricultural applications. Toxicon 158, 109–126. doi: 10.1016/j.toxicon.2018.11.298
Saez N. J., Senff S., Jensen J. E., Er S. Y., Herzig V., Rash L. D., et al. (2010). Spider-venom peptides as therapeutics. Toxins 2, 2851–2871. doi: 10.3390/toxins2122851
Schmitt G., Parrott N., Prinssen E., Barrow P. (2017). The great barrier belief: The blood–brain barrier and considerations for juvenile toxicity studies. Reprod. Toxicol. 72, 129–135. doi: 10.1016/j.reprotox.2017.06.043
Schwartz E. F., Capes E. M., Diego-García E., Zamudio F. Z., Fuentes O., Possani L. D., et al. (2009). Characterization of hadrucalcin, a peptide from Hadrurus gertschi scorpion venom with pharmacological activity on ryanodine receptors. Br. J. Pharmacol. 157, 392–403. doi: 10.1111/j.1476-5381.2009.00147.x
Silva C. N. D., Lomeo R. S., Torres F. S., Borges M. H., Nascimento M. C., Rapôso C., et al. (2018). PnTx2-6 (or δ-CNTX-Pn2a), a toxin from Phoneutria nigriventer spider venom, releases l-glutamate from rat brain synaptosomes involving Na+ and Ca2+ channels and changes protein expression at the blood-brain barrier. Toxicon 150, 280–288. doi: 10.1016/j.toxicon.2018.06.073
Skotland T., Iversen T. G., Torgersen M. L., Sandvig K. (2015). Cell-penetrating peptides: possibilities and challenges for drug delivery in vitro and in vivo. Molecules 20, 13313–13323. doi: 10.3390/molecules200713313
Spong K. E., Andrew R. D., Robertson R. M. (2016). Mechanisms of spreading depolarization in vertebrate and insect central nervous systems. J. Neurophysiol. 116, 1117–1127. doi: 10.1152/jn.00352.2016
Spong K. E., Rochon-Terry G., Money T. G., Robertson R. M. (2014). Disruption of the blood-brain barrier exacerbates spreading depression in the locust CNS. J. Insect Physiol. 66, 1–9. doi: 10.1016/j.jinsphys.2014.05.009
Strömstedt A. A., Pasupuleti M., Schmidtchen A., Malmsten M. (2009). Oligotryptophan-tagged antimicrobial peptides and the role of the cationic sequence. Biochim. Biophys. Acta 1788, 1916–1923. doi: 10.1016/j.bbamem.2009.06.001
Sugiura M., Horibe Y., Kawada H., Takagi M. (2008). Insect spiracle as the main penetration route of pyrethroids. Pestic Biochem. Phys. 91, 135–140. doi: 10.1016/j.pestbp.2008.03.001
Sukiran N. A., Pyati P., Willis C. E., Brown A. P., Readshaw J. J., Fitches E. C. (2023). Enhancing the oral and topical insecticidal efficacy of a commercialized spider venom peptide biopesticide via fusion to the carrier snowdrop lectin (Galanthus nivalis agglutinin). Pest Manag Sci. 79, 284–294. doi: 10.1002/ps.v79.1
Sumita Y., Kawada H., Minakawa N. (2016). Mode of entry of a vaporized pyrethroid knockdown agent into the body of the housefly, Musca domestica (Diptera: Muscidae). Appl. Entomol Zool 51, 653–659. doi: 10.1007/s13355-016-0443-2
Sweeney M. D., Sagare A. P., Zlokovic B. V. (2018). Blood-brain barrier breakdown in Alzheimer disease and other neurodegenerative disorders. Nat. Rev. Neurol. 14, 133–150. doi: 10.1038/nrneurol.2017.188
Takechi-Haraya Y., Saito H. (2018). Current understanding of physicochemical mechanisms for cell membrane penetration of arginine-rich cell penetrating peptides: role of glycosaminoglycan interactions. Curr. Protein Pept. Sci. 19, 623–630. doi: 10.2174/1389203719666180112100747
Tan H., Huang Y., Xu J., Chen B., Zhang P., Ye Z., et al. (2017). Spider toxin peptide lycosin-I functionalized gold nanoparticles for in vivo tumor targeting and therapy. Theranostics 7, 3168–3178. doi: 10.7150/thno.19780
Tang X., Yu D., Wang H., Lei Z., Zhai Y., Sun M., et al. (2022). Synaptotagmin 1-mediated cell membrane penetration and dopamine release enhancement by latroeggtoxin-VI. Int. J. Biol. Macromol 216, 906–915. doi: 10.1016/j.ijbiomac.2022.07.208
Temsamani J., Rousselle C., Rees A. R., Scherrmann J. M. (2001). Vector-mediated drug delivery to the brain. Expert Opin. Biol. Ther. 1, 773–782. doi: 10.1517/14712598.1.5.773
Tianpei X., Zhu Y., Li S. (2014). Optimized scorpion polypeptide LMX: a pest control protein effective against rice leaf folder. PloS One 9, e100232. doi: 10.1371/journal.pone.0100232
Trabulo S., Cardoso A. L., Mano M., De Lima M. C. (2010). Cell-penetrating peptides-mechanisms of cellular uptake and generation of delivery systems. Pharmaceuticals 3, 961–993. doi: 10.3390/ph3040961
Varadi M., Bertoni D., Magana P., Paramval U., Pidruchna I., Radhakrishnan M., et al. (2024). AlphaFold Protein Structure Database in 2024: providing structure coverage for over 214 million protein sequences. Nucleic Acids Res. 52, D368–D375. doi: 10.1093/nar/gkad1011
Wasserthal L. T., Fröhlich A. S. (2017). Structure of the thoracic spiracular valves and their contribution to unidirectional gas exchange in flying blowflies Calliphora vicina. J. Exp. Biol. 220, 208–219. doi: 10.1242/jeb.149013
Webster M. R., Socha J. J., Teresi L., Nardinocchi P., De Vita R. (2015). Structure of tracheae and the functional implications for collapse in the American cockroach. Bioinspir Biomim 10, 066011. doi: 10.1088/1748-3190/10/6/066011
Wei Z., Xu J., Peng X., Yuan Z., Zhao C., Guo K., et al. (2022). Preparation and performance characteristics of spider venom peptide nanocapsules. Pest Manag Sci. 78, 4261–4267. doi: 10.1002/ps.v78.10
Windley M. J., Herzig V., Dziemborowicz S. A., Hardy M. C., King G. F., Nicholson G. M. (2012). Spider-venom peptides as bioinsecticides. Toxins 4, 191–227. doi: 10.3390/toxins4030191
Wiranowska M., Colina L. O., Johnson J. O. (2011). Clathrin-mediated entry and cellular localization of chlorotoxin in human glioma. Cancer Cell Int. 11, 27. doi: 10.1186/1475-2867-11-27
Xiao L., Gurrola G. B., Zhang J., Valdivia C. R., SanMartin M., Zamudio F. Z., et al. (2016). Structure-function relationships of peptides forming the calcin family of ryanodine receptor ligands. J. Gen. Physiol. 147, 375–394. doi: 10.1085/jgp.201511499
Xue Q., Swevers L., Taning C. N. T. (2024). Drosophila X virus-like particles as delivery carriers for improved oral insecticidal efficacy of scorpion Androctonus australis peptide against the invasive fruit fly, Drosophila suzukii. Insect Sci. 31, 847–858. doi: 10.1111/1744-7917.13271
Yan L., Adams M. E. (1998). Lycotoxins, antimicrobial peptides from venom of the wolf spider Lycosa carolinensis. J. Biol. Chemsqs 273, 2059–2066. doi: 10.1074/jbc.273.4.2059
Yao H., Wang K., Wang Y., Wang S., Li J., Lou J., et al. (2015). Enhanced blood-brain barrier penetration and glioma therapy mediated by a new peptide modified gene delivery system. Biomaterials 37, 345–352. doi: 10.1016/j.biomaterials.2014.10.034
Yaroslavov A. A., Kuchenkova O. Y., Okuneva I. B., Melik-Nubarov N. S., Kozlova N. O., Lobyshev V. I., et al. (2003). Effect of polylysine on transformations and permeability of negative vesicular membranes. Biochim. Biophys. Acta 1611, 44–54. doi: 10.1016/S0005-2736(02)00701-0
Zhang P., Yan Y., Wang J., Dong X., Zhang G., Zeng Y., et al. (2020). An anti-cancer peptide LVTX-8 Inhibits the proliferation and migration of lung tumor cells by regulating causal genes’ expression in p53-related pathways. Toxins 12, 367. doi: 10.3390/toxins12060367
Zhao Y., Gan L., Ren L., Lin Y., Ma C., Lin X. (2022). Factors influencing the blood-brain barrier permeability. Brain Res. 1788, 147937. doi: 10.1016/j.brainres.2022.147937
Zhou X., Smith Q. R., Liu X. (2021). Brain penetrating peptides and peptide-drug conjugates to overcome the blood-brain barrier and target CNS diseases. WIREs Nanomed Nanobiotechnol 13, e1695. doi: 10.1002/wnan.v13.4
Zhu W., Vandingenen A., Huybrechts R., Vercammen T., Baggerman G., De Loof A., et al. (2001). Proteolytic breakdown of the Neb-trypsin modulating oostatic factor (Neb-TMOF) in the hemolymph of different insects and its gut epithelial transport. J. Insect Physiol. 47, 1235–1242. doi: 10.1016/S0022-1910(01)00086-5
Keywords: lipid bilayer, cell-penetrating peptides, blood-brain barrier, insect midgut, membrane-penetrating peptides, spider, scorpion, pseudoscorpion
Citation: Wang Y, Guo S, Jee KF and Herzig V (2024) Thou shalt not pass - arachnid venom peptides interacting with biological membranes. Front. Arachn. Sci. 3:1490313. doi: 10.3389/frchs.2024.1490313
Received: 03 September 2024; Accepted: 31 October 2024;
Published: 19 November 2024.
Edited by:
Tim Lüddecke, Fraunhofer Society (FHG), GermanyReviewed by:
Teresa Romero-Gutiérrez, University of Guadalajara, MexicoDavid Eagles, The University of Queensland, Australia
Copyright © 2024 Wang, Guo, Jee and Herzig. This is an open-access article distributed under the terms of the Creative Commons Attribution License (CC BY). The use, distribution or reproduction in other forums is permitted, provided the original author(s) and the copyright owner(s) are credited and that the original publication in this journal is cited, in accordance with accepted academic practice. No use, distribution or reproduction is permitted which does not comply with these terms.
*Correspondence: Volker Herzig, dmhlcnppZ0B1c2MuZWR1LmF1
†These authors have contributed equally to this work