- 1Branch for Bioresources, Fraunhofer Institute for Molecular Biology and Applied Ecology (IME), Giessen, Germany
- 2LOEWE Centre for Translational Biodiversity Genomics, Frankfurt am Main, Germany
- 3Institute for Insect Biotechnology, Justus Liebig University Giessen, Giessen, Germany
Spiders are ancient and highly successful predators, which use venom for both predation and defense. Their venoms are complex mixtures of potent biological molecules, emerging as a prolific source of biomolecular innovation in agriculture, biomedicine, and bioeconomy. While small cysteine-rich neurotoxins are typically considered the main components of spider venoms, recent research has shown that spider venoms also contain many high-molecular-weight proteins, especially enzymes. To date, very little is known about the diversity, biochemistry and ecology of these components. Here, we provide the first systematic overview of spider venom enzymes, describing all known examples in terms of their properties and functions in the spider venom system. We argue that the sheer diversity of these neglected spider venom compounds offers significant translational potential and holds great potential for the bioeconomy, reflecting a wide range of technical applications such as industrial production, food processing, and waste management.
1 Introduction
Spiders (order Araneae) are one of the most species-rich taxon of arthropods, abundant in most terrestrial ecosystems, and occurring on all continents except Antarctica (Selden and Penney, 2010; World Spider Catalog, 2024). They have evolved into highly effective predators that primarily hunt insects, deploying potent venoms to subjugate their prey (Lüddecke et al., 2022).
Spider venom is a complex mixture of proteins and peptides containing up to 3000 different bioactive molecules (Pineda et al., 2020). The main active principles of spider venoms are short neurotoxic peptides with an inhibitory cysteine knot (ICK) motif (King and Hardy, 2013). These interact with ion channels and receptors in the central nervous system of prey, facilitating rapid immobilization (Vassilevski et al., 2009; Kuhn-Nentwig et al., 2011; Langenegger et al., 2019). Many ICKs also target disease-related ion channels, and are therefore promising for biomedical applications (Saez et al., 2010). Accordingly, biodiscovery programs have investigated venom profiles across the spider tree of life, unveiling several different venom compositions, and enabling the characterization of many novel toxins. These studies mostly involved bioactivity-guided strategies which, due to methodological limitations, restricted the available range of examinable taxa to the few larger representatives (Herzig et al., 2019; Lüddecke et al., 2019). More recently, modern venomics technologies that combine genomics, transcriptomics and proteomics with biotechnology have allowed the rapid, cost-effective analysis of the smaller, less accessible spiders representing the majority of the global arachnofauna (Lüddecke et al., 2019).
Recently, several paradigm shifts in spider toxinology have been promoted, one of them being the unanticipated prevalence and potential biological significance of the high-molecular-weight components present in spider venoms, particularly enzymes (Lüddecke et al., 2022). Spider venoms were originally thought to comprise mainly ICK peptides, although members of the RTA-clade (i.e., wandering spiders) had also recruited a diverse range of small linear cytolytic venom peptides (Langenegger et al., 2019; Lüddecke et al., 2022). However, the expansion of venom taxonomic space facilitated by venomics has shown that many species also produce larger proteins and enzymes as major venom components. This includes CAP (cysteine-rich secretory proteins, antigen 5, and pathogenesis-related 1) proteins in orb-weaver spiders (family Araneidae) (Lüddecke et al., 2020), metalloproteases in the family Pholcidae (Zobel-Thropp et al., 2019), and phospholipase D in the family Sicariidae (Dantas et al., 2016).
Spider venom enzymes fulfill important functions in the context of venom system physiology, such as the cleavage of propeptides to activate venom components and facilitate protein maturation (Langenegger et al., 2018). They may also promote the synergistic “dual prey-inactivation strategy”, in which an initial wave of non-specific components attack a broad array of biochemical targets, followed by a specific neurochemical salvo based on highly specialized neurotoxins (Kuhn-Nentwig et al., 2019). In this framework, enzymes are the principal components of the first wave, interfering with various metabolic and physiological processes to support the rapid onset of neurotoxic symptoms in the second wave (Kuhn-Nentwig et al., 2019). Additionally, functional overlap between venom enzymes and enzymes involved in extra-intestinal digestion may also exist. Based on these insights, we recently conducted the first meta-analysis of spider venom databases paired with a re-analysis of all reported spider venom proteomes, to determine whether enzymes are more prominent than reported (Dresler et al., 2024). Our exploratory work identified 144 enzyme families that span all known enzyme classes in the EC system across 17 spider venom families (Dresler et al., 2024).
While the biological role of most spider venom enzymes remains unclear, 34 of them have been assigned to known venom functions (Figure 1). This initial functional assignment was based on a classification established for scorpion venom enzymes, and the following six functional classes are recognized: 1) toxic enzymes that are used in hunting and defense, 2) pre-digestive enzymes that break down tissue and support extra-intestinal digestion, 3) spreading factor enzymes that support the uptake of co-secreted toxins, 4) venom component activators that are involved in protein maturation, 5) preservative enzymes that defend the venom gland against noxious molecules, 6) multifunctional enzymes (Delgado-Prudencio et al., 2022). Furthermore, a wide variety of functionally uncharacterized molecules with potential enzymatic activity exits in spider venoms. The diverse biological processes mediated by enzymes suggest that these venom components are indeed prominent but almost completely neglected. Currently, little is known about the biochemical ecology (i.e., structure, chemistry, and function) of spider venom enzymes. However, given their biological refinement and functionality in chemically challenging environments, such as insect hemolymph, they may add to the translational potential in bioeconomy, including chemical production, waste reduction, or food processing (Singh et al., 2016; Mesbah, 2022). Exploiting such evolutionary innovations requires a comprehensive analysis of the different types of spider venom enzymes, their diversity, chemistry, and translational aspects.
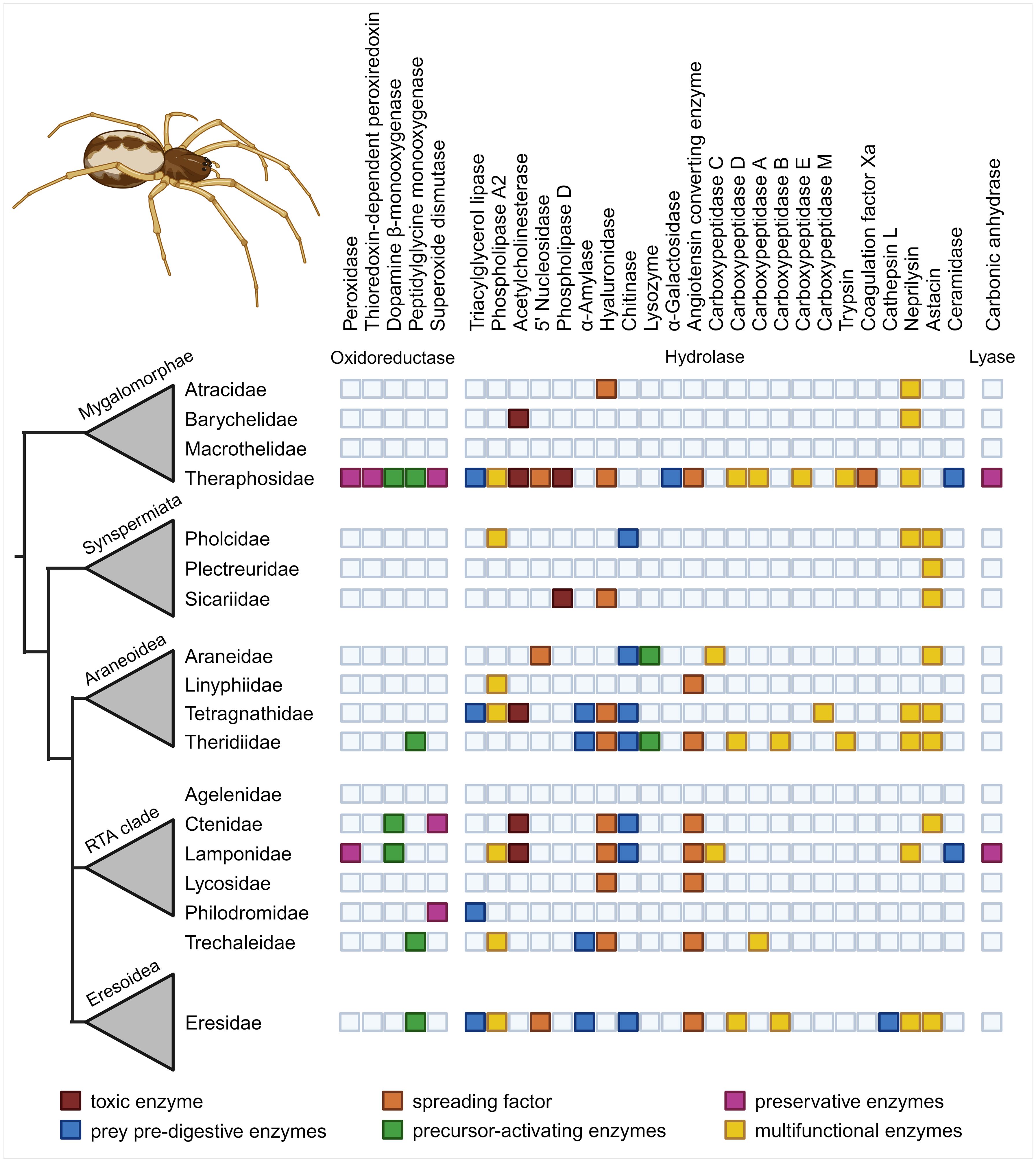
Figure 1. Enzymes found in spider venoms and their potential venom functions. Identified enzyme classes and putative function are plotted on a simplified cladogram showing major phylogenetic spider clades and families (Dresler et al., 2024).
We hereby provide the first systematic overview of known spider venom enzymes, categorizing and discussing them hierarchically, based on their putative functions and utilization potential in the bioeconomy. Our work adds to the body of literature highlighting the unanticipated chemical and functional diversity within spider venoms, and provides a basis for the future investigation of spider venom systems. As most spider venom enzymes have only been identified through proteo-transcriptomic analyses and lack experimental evidence for their bioactivity and, consequently, their biological function, we follow the functional assignment previously established for scorpions. This classification is currently the only available system for arachnid venom enzymes, and is thus considered a good starting point. However, future research on spider venom activity and function may require revising our initial classification based on novel insights.
2 Functional classes of spider venom enzymes
Although little is known about the function and biological roles of spider venom enzymes, much more information is available about the enzymes from non-spider arachnids, particularly scorpions (Ahmadi et al., 2020; Delgado-Prudencio et al., 2022). The principal role of spider and scorpion venom is similar (i.e., neurotoxic activity to facilitate prey capture; Ahmadi et al., 2020; Delgado-Prudencio et al., 2022) and the two groups are relatively closely phylogenetically related. Therefore, our functional assignment of spider venom enzymes is based on the classification established for scorpions (Delgado-Prudencio et al., 2022). However, this classification should be considered preliminary because experimental data is missing for most enzyme families. Accordingly, future work should validate the assignments described herein. To date, 34 of 144 described spider venom enzyme families have been assigned to the functional groups of toxicity, prey pre-digestion, spreading factors, venom component activation and venom preservation (Dresler et al., 2024). Whereas, 15 of the remaining 110 enzyme families have been assigned to cellular functions such as part of the metabolism or cellular components. However, due to the lack of further information, 95 enzyme families remain unclassified. This review explores only those enzyme families with assignments to venom functions. These are summarized in Table 1 and described in more detail below.
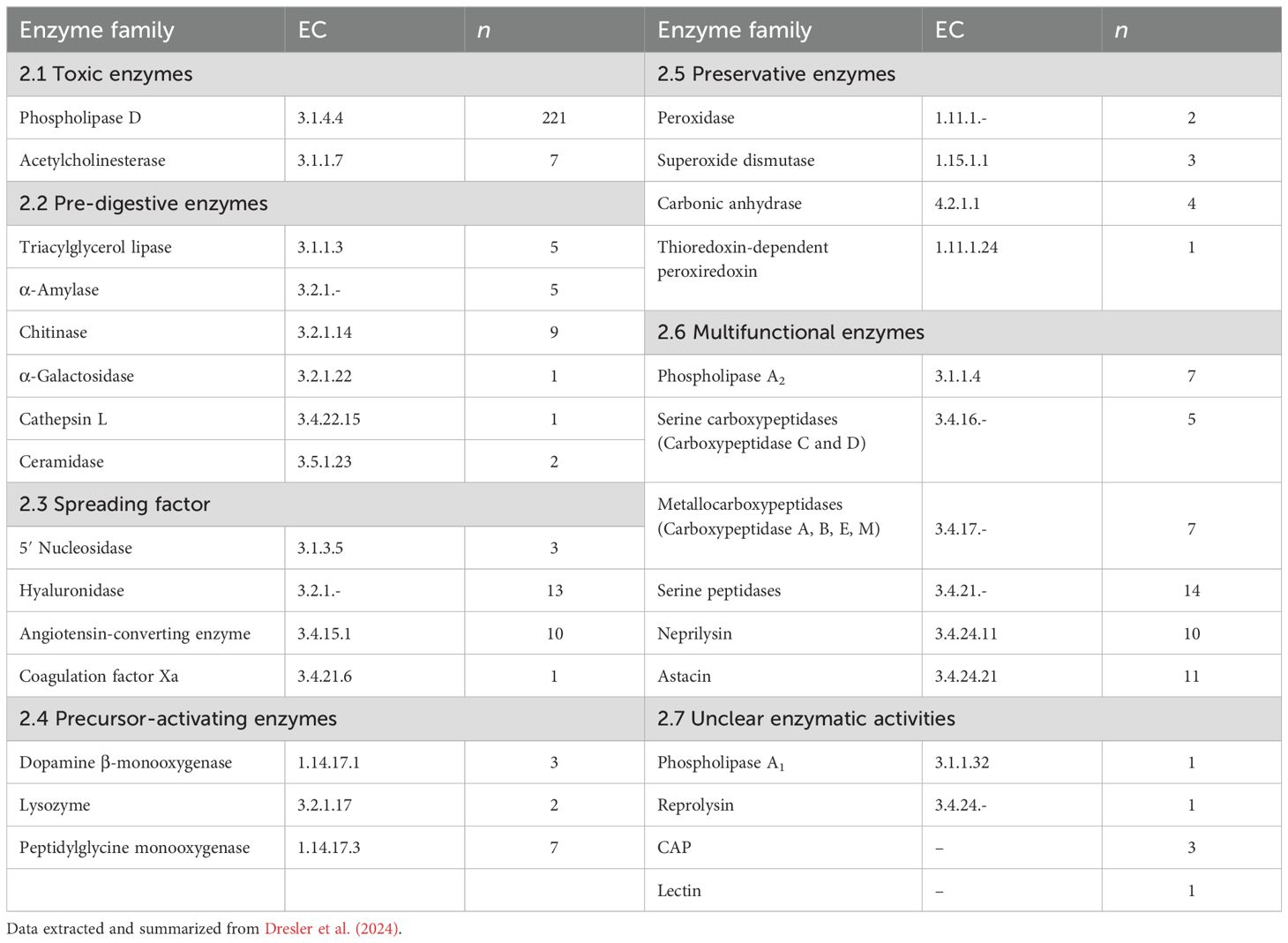
Table 1. Spider venom enzymes grouped by potential functional group, EC numbers, and number of identified proteins in spider venoms (n).
2.1 Toxic enzymes
Toxic enzymes are directly involved in envenomation (Delgado-Prudencio et al., 2022). Similarly to neurotoxins, these compounds facilitate the fast incapacitation of prey, and can cause a range of deleterious effects on tissues and/or physiological processes.
2.1.1 Phospholipase D
Phospholipase D (PLD, EC 3.1.4.4) from spider venom, also known as choline phosphodiesterases, is a family of phospholipases that catalyze cyclization, specifically transphophatidylation of phosphodiester bonds in phospholipids resulting in the formation of cyclic phosphatases (Lajoie et al., 2013). PLDs have been found in the venoms of sicariid spiders, such as the genera Loxosceles (Barbaro et al., 1996; Lee and Lynch, 2005; Kalapothakis et al., 2007; Catalán et al., 2011), Hexophtalma (Binford et al., 2009) and Sicarius (Binford et al., 2009; Lajoie et al., 2015). Recent studies also proposed the presence of PLDs in the venom of the theraphosid spider Pamphobeteus verdolaga (Estrada-Gómez et al., 2017, 2021). Earlier works on PLD evolution suggest that they originated via horizontal gene transfer in the deep evolutionary history of arthropods (Cordes and Binford, 2018).
Spider venom PLDs are typically monomeric proteins of 30–35 kDa (Chaim et al., 2006; Kalapothakis et al., 2007). They are expressed as preproproteins of 302–307 amino acids (see Figure 2) that are processed into the active protein by removing a 12-residue signal peptide and a 10-residue propeptide, leaving 280–285 residues that fold into the mature protein (Kalapothakis et al., 2002; Gremski et al., 2014). Their three-dimensional structure typically features eight α-helices encircling eight β-strands to form an (α/β)8 barrel or triose-phosphate isomerase (TIM) barrel (Masood et al., 2018). The active site includes the catalytically active amino acid residues His12 and His47, however Trp230 and Asp233 have been shown to also play important roles in substrate binding and catalytic activity (Murakami et al., 2005, 2006; Catalán et al., 2014). Based on their structure, spider venom PLDs can be assigned to class I, featuring three cysteine residues, two forming a disulfide bridge, and five residues in an extended flexible loop or class II, which features four cysteine residues forming two disulfide bridges, one connecting the catalytic region to a flexible loop and reducing the size of the active site cavity (Murakami et al., 2006; de Giuseppe et al., 2011).
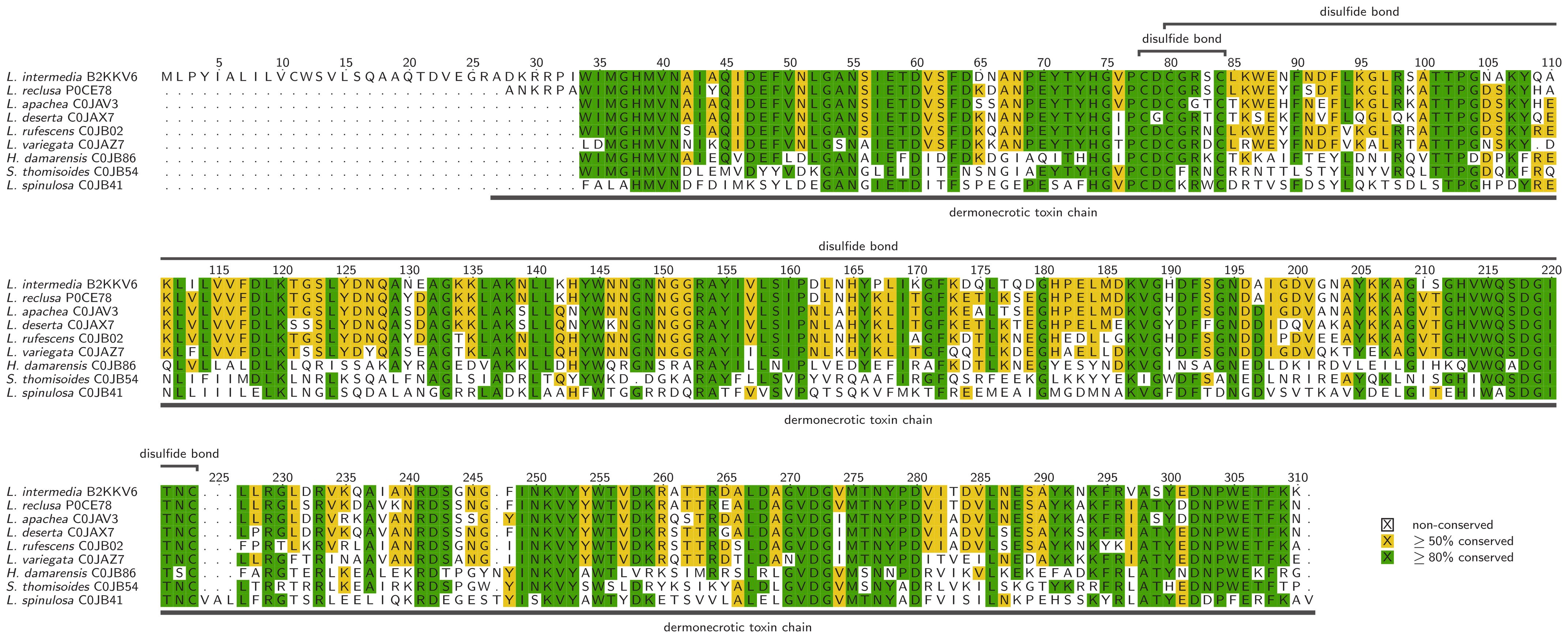
Figure 2. Alignment of selected phospholipase Ds, identified in spider venom with additional data corresponding to the UniProt entries. Alignments were visualized with TEXshade in identical mode (Beitz, 2000).
The chemical reaction catalyzed by PLD involves the cleavage of phosphodiester bonds in phospholipids, particularly lyso- and sphingolipids, yielding cyclic phosphatases phosphatases (Lajoie et al., 2013). Many lipids or lipid-derived products generated by phospholipases acting on membrane phospholipids are mediators or second messengers in signal transduction (Dennis et al., 1991). The formation of lipid metabolites such as ceramide 1-phosphate (C1P) or lysophosphatidic acid (LPA) may contribute to spider venom toxicity triggering pathophysiological changes (Anliker and Chun, 2004; Lee and Lynch, 2005). In contrast to most other spider venom enzymes, PLDs have been studied in detail because they are medically relevant. In Loxosceles venom, PLDs are the primary component responsible for severe dermo-necrosis and even death (Futrell, 1992; Senff-Ribeiro et al., 2008; Lajoie et al., 2013; Chaves-Moreira et al., 2019).
2.1.2 Acetylcholinesterase
Acetylcholinesterase (AChE, EC 3.1.1.7), also known as choline esterase I, cholinesterase, or true cholinesterase (Bairoch, 2000), is a single polypeptide ~550 amino acids in length. The first AChE structure to be described was from the Pacific electric ray Torpedo californica, featuring 14 α-helices surrounding a 12-stranded mixed β-sheet with an active site comprising a planar array formed by three residues in loops within highly conserved regions (Sussman et al., 1991; Dvir et al., 2010). AChE has been identified in the venom of three spider families: Barychelidae, Theraphosidae and Ctenidae (Undheim et al., 2013; Diniz et al., 2018; Zobel-Thropp et al., 2018; Câmara et al., 2020; Michálek et al., 2022; Nishiduka et al., 2022).
The physiological function of AChE is to catalyze the rapid hydrolysis of the neurotransmitter acetylcholine (ACh) into choline and acetate (Colovic et al., 2013). This leads to the termination of impulse transmission at cholinergic synapses, allowing cholinergic neurons to return to their resting state after activation, thus regulating normal cognitive and motor activities (Barnard et al., 1975; Colovic et al., 2013). AChE is found not only in spider venom (Undheim et al., 2013; Borges et al., 2016; Diniz et al., 2018; Khamtorn et al., 2022), but also in the venom of ants (Cai et al., 2022) and snakes (Frobert et al., 1997), suggesting a broad functional role in envenomation. High levels of AChE lead to the rapid depletion of ACh, negatively affecting the function of neuromuscular junctions in prey. The spider venom AChE is therefore likely to disrupt neuromuscular transmission in a similar manner to cholinotoxins, potentially triggering symptoms such as flaccid paralysis and respiratory depression/failure, ultimately facilitating its subjugation (de Roodt et al., 2017; Friedman et al., 2021). However, an AChE from spider venom has yet to be isolated or expressed, so the function of this enzyme has not been validated directly and is only in silico predicted.
2.2 Pre-digestive enzymes
Enzymes with digestive functions are important for extra-intestinal digestive processes. They are present in spider digestive fluids, but have also been identified repeatedly in their venoms (Fuzita et al., 2016; Walter et al., 2017; Valladão et al., 2023). This indicates that the extra-intestinal digestion begins soon after envenomation (Fuzita et al., 2016; Walter et al., 2017). Once injected, the enzymes begin to break down larger biomolecules, resulting in the rapid dissolution of prey tissue (Vanthournout et al., 2016). Insects, the main prey of spiders, are rich in proteins and lipids. In line with this, the digestive enzymes typically found in spider venoms are hydrolases such as proteases and lipases (Fuzita et al., 2016; Walter et al., 2017; Lüddecke et al., 2022; Valladão et al., 2023).
2.2.1 Triacylglycerol lipase
Triacylglycerol lipase (EC 3.1.1.3), also known as lipase, tributyrase and triglyceride lipase, is characterized by an α/β hydrolase fold and a conserved catalytic triad stabilized by a variable number of disulfide bonds (Casas-Godoy et al., 2012). The α/β fold exhibits a central β-sheet with eight β-strands, seven of them parallel and one (β2) antiparallel, and the α-helices are forming connections between β-strands β3–β8. The catalytic triad features serine, aspartate/glutamate and histidine residues similar to that of serine proteases (Brady et al., 1990). In contrast to the conserved catalytic triad, the substrate binding site is variable, but includes hydrophobic residues inside a pocket of the central β-sheet (Pleiss et al., 1998). Triacylglycerol lipases catalyze the cleavage of carboxylester bonds in long-chain alcylglycerols (>10 carbon atoms), specifically the hydrolytic cleavage of triacylglycerol into diacylglycerol and a fatty acid. This differentiates them from esterases, which cleave shorter chains (<10 carbon atoms) (Casas-Godoy et al., 2012). Depending on the thermodynamic conditions, lipases can also be involved in a variety of anabolic reactions, such as esterification and transesterification (Casas-Godoy et al., 2012) and may also feature additional cholesterol esterase, chitinase or amidase activities (Svendsen, 2000).
Triacylglycerol lipases have been found in the venom of Stegodyphus mimosarum, Tibellus oblongus, Tetragnatha versicolor and Acanthoscurria sp (Sanggaard et al., 2014; Zobel-Thropp et al., 2018; Nishiduka et al., 2022; Korolkova et al., 2023). In addition, potential triacylglycerol lipases have been retrieved from the venoms of Phoneutria nigriventer, Lampona sp., Acanthoscurria natalensis, Parasteatoda tepidariorum and Steatoda nobilis, but these require further analysis to validate their family-level assignment (Diniz et al., 2018; Haney et al., 2019; Câmara et al., 2020; Dunbar et al., 2020; Michálek et al., 2022). Lipases fulfill diverse physiological functions in lipid and lipoprotein metabolism, most importantly in the triglyceride catabolism (Sharma et al., 2001). In spiders, lipases are found in both digestive secretions and venom, indicating a role in extra-intestinal digestion (Walter et al., 2017).
2.2.2 α-amylase
The α-amylases (EC 3.2.1.1), also known as glycogenases, are hydrolases that are assigned to glycoside hydrolase family 13 (GH13), GH57 or GH119, depending on their sequence (Janecek et al., 2014). They contain seven conserved regions that form the characteristic (α/β)8 barrel and share a conserved catalytic triad (Asp206, Glu230 and Asp297) (Figure 3). These enzymes catalyze the cleavage of α-1,4-glycosidic bonds in polysaccharides, so their main function is carbohydrate catabolism (Da Lage, 2018; Kumar and Chakravarty, 2018). They have been found in the venom of several spiders, including Stegodyphus mimosarum, Tetragnatha versicolor, Parasteatoda tepidariorum, Steatoda nobilis and Cupiennius salei (Sanggaard et al., 2014; Walter et al., 2017; Zobel-Thropp et al., 2018; Haney et al., 2019; Kuhn-Nentwig et al., 2019; Dunbar et al., 2020).
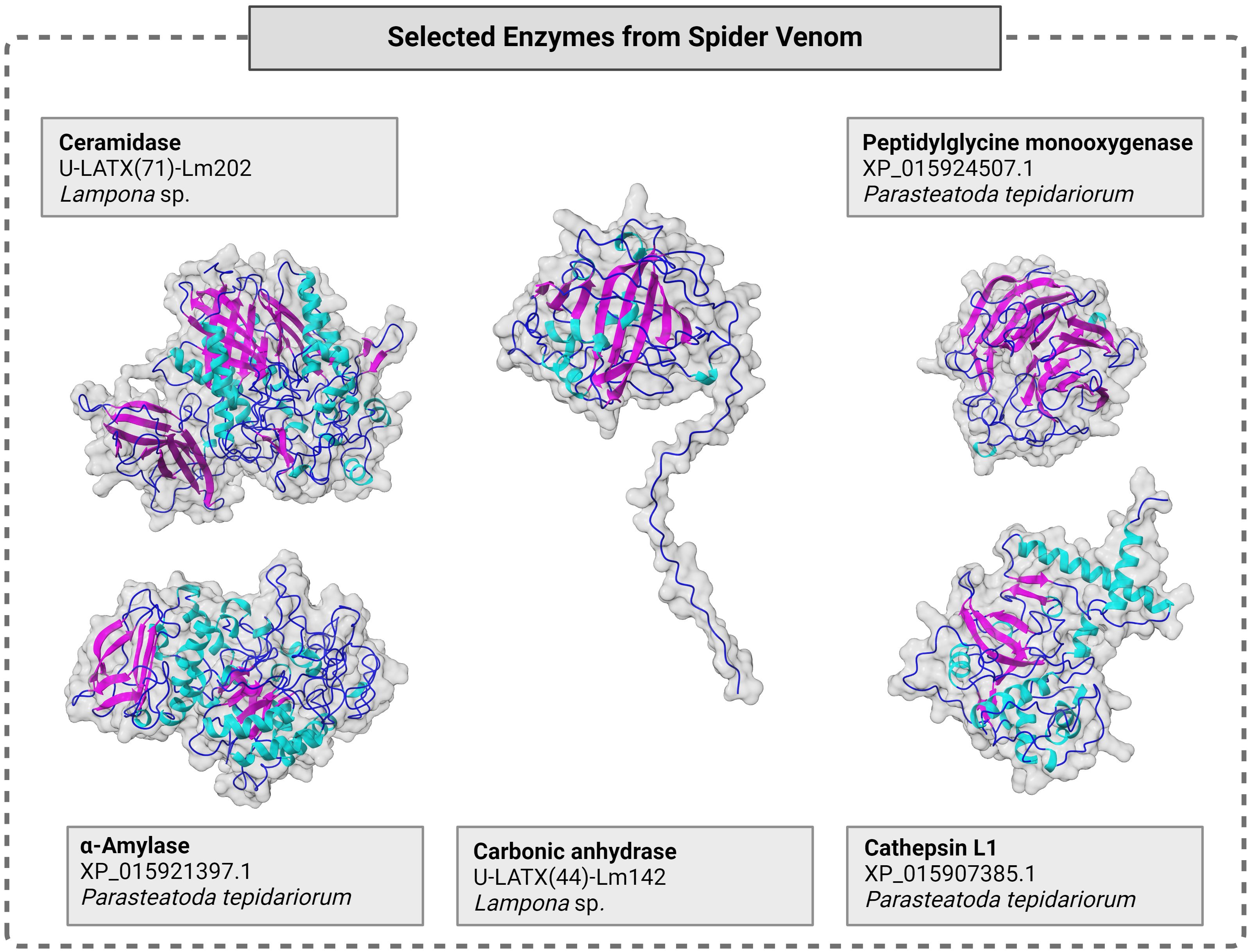
Figure 3. Molecular structure of selected spider venom enzymes with surface and cartoon presentation of secondary structures in cyan (helix), magenta (sheet), marine (loop). Sequences were taken from the literature (Haney et al., 2019; Michálek et al., 2022) and structures were modeled using Alphafold 3 (Abramson et al., 2024) and illustrated using chimera X under default settings. For each enzyme, the respective enzyme class, its identifier from the venomic dataset and the analyzed species are given. Full data, including sequences, are presented in Supplementary Table S1.
In addition to pre-digestion, α-amylases may help to digest pollen attached to insect prey (Fuzita et al., 2016; Walter et al., 2017; Valladão et al., 2023). Additionally, neofunctionalization may have conferred an active role in prey incapacitation as predicted by the dual prey-inactivation strategy (Kuhn-Nentwig et al., 2019). This may be achieved by releasing large amounts of glucose from glycogen stored in the hemolymph and muscles of insect prey, which disrupts energy homeostasis leading to potentially fatal hyperglycemia (Kuhn-Nentwig et al., 2019).
2.2.3 Chitinase
Chitinase (EC 3.2.1.14), also known as 1,4-β-poly-N-acetylglucosaminidase, chitodextrinase and poly-β-glucosaminidase (Bairoch, 2000), can refer to glycoside hydrolases representing families GH18, GH19 or GH20 (Henrissat and Bairoch, 1993) and are found in most living organisms (Patil et al., 2000). Their size ranges from 20–90 kDa (Bhattacharya et al., 2007) and they are diverse in terms of molecular structure, surface specificity and catalytic mechanism (Muzzarelli, 1999; Kasprzewska, 2003; Hamid et al., 2013). Chitinases have been found in venom from the araneomorph species Argiope bruennichi and Araneus ventricosus, Phoneutria nigriventer, Stegodyphus mimosarum, Lampona sp., Physocyclus mexicanus, Tetragnatha versicolor, Parasteatoda tepidariorum and Steatoda nobilis (Duan et al., 2013; Sanggaard et al., 2014; Diniz et al., 2018; Zobel-Thropp et al., 2018, 2019; Haney et al., 2019; Dunbar et al., 2020; Lüddecke et al., 2020; Michálek et al., 2022).
Given the diversity of the chitinase family, there is no general structure or mode of action, but many chitinases contain a serine/threonine-rich glycosylated domain and a cysteine-rich chitin-binding site as catalytic domains (Blaxter, 1996).
In invertebrates, a C-terminal structural motif containing six cysteine residues forms the chitin-binding domain (Venegas et al., 1996). Chitinases cleave internal bonds (endochitinases) or progressively remove external residues (exochitinases) to form small chito-oligomers (Yuli et al., 2004). Moreover, the degradation of chitinase products to N -acetylglucosamine dimers or monomers ultimately involves at least one additional enzyme, which has been identified as β- N -hexosaminidase in the digestive fluids of spiders (Mommsen, 1980; Harman et al., 1993; Sahai and Manocha, 1993).
Invertebrate chitinases facilitate ecdysis, which breaks down the cuticle into chito-oligomers, and may also provide a defensive tool against insect parasites (Koga et al., 1997). In arachnid venom, chitinases may facilitate prey capture and feeding by breaking down the exoskeletons of insect prey (Mommsen, 1980; Fuzita et al., 2016; Liberato et al., 2016; Walter et al., 2017; Delgado-Prudencio et al., 2022). Additionally, they may serve as immune response proteins, given their potency in attacking cell walls of bacteria and fungi (Walter et al., 2017). Similar key functions are reported from other arthropods as well (Bolognesi et al., 2005; Genta et al., 2006).
2.2.4 α-galactosidase
The α-galactosidases (EC 3.2.1.22), also known as melibiases (Bairoch, 2000), represent four families of glycoside hydrolases from bacteria, fungi, plants and animals (Anisha, 2017; Kote et al., 2020), although all animal enzymes are assigned to family GH27 (Weignerová et al., 2009). The enzymes range in size from 30–65 kDa (E et al., 2015). These enzymes have been found in the venom of the theraphosid spider Acanthoscurria geniculata (Sanggaard et al., 2014). They generally occur as homodimers, with each monomer containing an active site featuring a (β/α)8 domain and an antiparallel β domain (Garman and Garboczi, 2004; Golubev et al., 2004).
They catalyze the cleavage of glycoproteins, glycolipids and polysaccharides (Guce et al., 2010), as well as the cleavage of terminal α-d-galactopyranosyl residues from galactomannans and oligosaccharides (Golubev et al., 2004; Katrolia et al., 2014). They are typically associated with the degradation of larger polymeric substrates (Kim et al., 2011; Benzertiha et al., 2019).
2.2.5 Cathepsin L
Cathepsin L (EC 3.4.22.15) is a cysteine endopeptidase involved in multiple intracellular processes (Turk et al., 2012) but it is also secreted from lysosomes, suggesting an additional extracellular role (Yadati et al., 2020). This enzyme has been found in the venom of the eresid Stegodyphus mimosarum and theridiid Parasteatoda tepidariorum (Sanggaard et al., 2014; Haney et al., 2019), and have been predicted in the venoms of four other spiders (Diniz et al., 2018; Dunbar et al., 2020; Michálek et al., 2022; Nishiduka et al., 2022). The structure of cathepsin L (Figure 3) is similar to that of papain-like cysteine proteases and it is characterized by ERFNIN and GNFD motifs, as well as L and R domains featuring α-helices and β-sheets, respectively. These domains are separated in a V-shape that forms the catalytic site, which contains the key residues Cys25 and His163. The molecular weight of cathepsin L ranges from 25–35 kDa (Gunčar et al., 1999; Dong-hui, 2012). The hydrolytic function of the enzyme is similar to that of papain (BRENDA Enzyme Database, 2024).
Cathepsin L is the most abundant lysosomal protease and is known for its role in intracellular protein degradation, immune response, and metabolism (Yadati et al., 2020). It processes peptide neurotransmitters within secretory vesicles (Yadati et al., 2020) and is also a pheromone processing enzyme (Yasothornsrikul et al., 2003). The biological role of cathepsin L in spider venom is unclear, however it has been shown to be the most abundant enzyme in the digestive fluids of arachnids (Santamaría et al., 2012; Fuzita et al., 2015b). Additionally, cathepsin L is an important digestive enzyme in ticks (Franta et al., 2010), mites (Carrillo et al., 2011) and scorpions (Fuzita et al., 2015a). Therefore it may be involved in pre-digestion in spider venom or may act on neurological pathways in the prey or in the venom gland (Yasothornsrikul et al., 2003; Zhang et al., 2010; Fuzita et al., 2015b; Delgado-Prudencio et al., 2022).
2.2.6 Ceramidase
Ceramidases (EC 3.5.1.23), also known as acylsphingosine deacylases, are classified as acid, neutral (NCs) or alkaline ceramidases (ACERs) according to their optimal pH cleaving fatty acids from ceramides (Coant et al., 2017). The different subgroups show low degrees of sequence similarity and have different reaction mechanisms (Ito et al., 2014). A single NC has been found in spider venom thus far (Lampona sp.) and a putative NC/ACER has been predicted in Acanthoscurria natalensis (Câmara et al., 2020; Michálek et al., 2022). NCs consist of a catalytic N-terminal domain, a short linker and an immunoglobulin-like C-terminal domain (Figure 3) (Ito et al., 2014). They exist as soluble or single-pass transmembrane proteins (Ito et al., 2014). Their active site consists of a narrow hydrophobic pocket with a coordinated Zn2+ ion cleaving the amide bond of ceramides, producing sphingosine and a fatty acid (Ito et al., 2014). Sphingolipids were originally thought to act solely as structural components of cell membranes, but recently were recognized as bioactive lipids involved in processes such as growth, differentiation and angiogenesis (Coant et al., 2017). By converting ceramides to sphingosines, ceramidases may regulate the availability of these versatile bioactive lipids (Coant et al., 2017). The functional role of ceramidases in spider venom remains unknown, but they may contribute to prey digestion because ceramide is a key building block of insect cell membranes (Shi et al., 2021).
2.3 Spreading factors
Spreading factors target the extracellular matrix (ECM), disrupting the integrity of cells and tissues to promote the uptake and distribution of co-secreted toxins. This can be achieved via various mechanisms, including the cleavage of proteins, the degradation of lipid bilayers, or pore formation. Spreading factors are therefore important determinants of venom activity because they directly contribute to the rapid onset of envenomation symptoms, which is particularly important in the case of predatory venoms (Langenegger et al., 2019; Delgado-Prudencio et al., 2022).
2.3.1 5′ Nucleotidase
The 5′ nucleotidases (EC 3.1.3.5) are hydrolases that catalyze the hydrolysis of phosphodiester bonds in ribonucleotides. They have a monomeric mass of 60–100 kDa but are found as soluble or membrane-bound dimers and tetramers (Zimmermann, 1992). Many such enzymes feature disulfide bonds, glycosylation, coordinated Zn2+ ions, and (in the case of membrane-bound enzymes) ecto-5′ nucleotidase lipidation via a glycophosphatidylinositol (GPI) anchor (Zimmermann, 1992).
The physiological role of 5′ nucleotidases is to control the intra- and extracellular level of nucleoside 5′-monophosphates (Schetinger et al., 2007). Such enzymes have also been detected in the venom of insects, cnidarians and reptiles (Dhananjaya and D’Souza, 2010; Macrander et al., 2016; Walker et al., 2018; Fischer et al., 2023) as well as the spiders Argiope bruennichi, Stegodyphus mimosarum and Pamphobeteus verdolaga (Sanggaard et al., 2014; Lüddecke et al., 2020; Estrada-Gómez et al., 2021). Although their precise role is not clear, structurally related enzymes identified in fish-hunting bugs and snakes are known to inhibit ATP-induced thrombocyte aggregation (Trummal et al., 2015; Walker et al., 2018). Nucleotide-cleaving enzymes may also interfere with the prey’s nucleotide metabolism and nucleotide-dependent signaling (Aird, 2002; Hunsucker et al., 2005).
2.3.2 Hyaluronidase
Hyaluronidases (EC 3.2.1.35) are hydrolases that break down hyaluronic acid and chondroitin sulfate into oligosaccharides (Bordon et al., 2015). All hyaluronidases found thus far in spider venom belong to family GH56 and are monomers of 30–60 kDa, featuring disulfide bonds and glycans (Sutti et al., 2014). They have been found in venom from eight spider families, including Atracidae, Ctenidae and Sicariidae (Duan et al., 2008; Diniz et al., 2018; Zobel-Thropp et al., 2018; Haney et al., 2019; Kuhn-Nentwig et al., 2019; Câmara et al., 2020; Koua et al., 2020; Estrada-Gómez et al., 2021; Cardoso et al., 2022; Michálek et al., 2022; Nishiduka et al., 2022).
Hyaluronic acid is a key component of the vertebrate ECM (Yamada et al., 2011; Nagaraju, 2016). Accordingly, hyaluronidases are involved in a number of physiological processes that require ECM remodeling, such as cell migration, inflammation, and wound healing (Dos Santos et al., 2009). They are presumed to act as spreading factors when expressed in venom systems (Futrell, 1992; Ferrer et al., 2013) but the insect ECM lacks hyaluronic acid and the role of such enzymes must therefore be restricted to vertebrate prey (Hynes and Walton, 2000; Buhren et al., 2016). This is most likely the case for large spiders, which can predate small vertebrates (Gudger, 1931; Nyffeler and Knörnschild, 2013; Kuhn-Nentwig et al., 2019; Reyes-Olivares et al., 2020; Nyffeler and Gibbons, 2022). Furthermore, hyaluronidases may be utilized as a defensive mechanism against vertebrate predators, potentially facilitating the dispersal of other venom components (Girish and Kemparaju, 2007). Recent studies have demonstrated that, in addition to hyaluronic acid, chondroitin serves as a substrate for most spider venom hyaluronidase-like enzymes. Given that invertebrates contain chondroitin and its sulfated form (chondroitin sulfate) in place of hyaluronic acid, the degradation of these may present the spreading properties observed (Biner et al., 2015).
2.3.3 Angiotensin-converting enzyme
Angiotensin-converting enzyme (ACE, EC 3.4.15.1), also known as peptidyl-dipeptidase A, carboxycathepsin, dipeptidyl carboxypeptidase I, kininase II, peptidase P or peptidyl dipeptidase I, cleaves dipeptides from the C-terminus and occurs as two isoforms in mammals (Bairoch, 2000). Somatic ACE (150–180 kDa) has two catalytic sites, whereas testicular ACE (90–110 kDa) has only one (Langford et al., 1993; Kondoh et al., 2005; Zhang et al., 2013). Similar to carboxypeptidase A the catalysis is facilitated by the activation of a water nucleophile and an acid, leading to the cleavage of amide C-N bonds (Zhang et al., 2013). Yet there is little sequence similarity between these enzymes other than the conserved HEXXH Zn2+-binding motif (Zhang et al., 2013).
Invertebrate homologs of mammalian ACE are single-domain M2-type zinc metalloproteases lacking the C-terminal membrane anchor found in mammals, and are therefore soluble extracellular proteins (Coates et al., 2000; Salzet et al., 2001). Most invertebrate ACEs have a catalytic site similar to mammalian ACE, but some variants lack the catalytic domain (Turner and Hooper, 2002). Plesiotypic ACE is a key component of the renin-angiotensin system (RAS) that converts angiotensin to its active form thereby regulating e.g. blood pressure in vertebrates (Coates, 2003). In case of invertebrates, the RAS is involved in osmoregulation, memory processes, reproduction, and immune responses (Salzet et al., 2001).
ACE has been found in the venom of 10 spiders, including Phoneutria nigriventer, Acanthoscurria sp. and Parasteatoda tepidariorum (Sanggaard et al., 2014; Diniz et al., 2018; Haney et al., 2019; Nishiduka et al., 2022). The function of ACE in spider venom is unclear, but ACE-like venom compounds may induce hypertensive effects in vertebrate prey (Murthy and Vakil, 1988; Safavi-Hemami et al., 2013; Kuhn-Nentwig et al., 2019) and may cleave neuropeptides to compromise the physiological functions of prey, thus preventing escape (Cajado-Carvalho et al., 2016). ACE-like venom compounds may also be important for venom gland physiology, similar to other venom peptidases (Langenegger et al., 2018). Facing their activity in osmoregulation they may also be involved in trophic interactions and serving as unspecific effectors targeting the metabolism of invertebrate prey (Kuhn-Nentwig et al., 2019). Thus, although these enzymes have been primarily assigned as potential spreading factors, they may equally well serve as toxic components.
2.3.4 Coagulation factor Xa
Coagulation factor Xa (FXa, EC 3.4.21.6), sometimes described as Stuart factor, is the activated form of the zymogen factor X (FX) in the blood coagulation cascade. FXa is composed of a light chain and a heavy chain joined by a disulfide bond. The light chain features an N-terminal γ-carboxyglutamic acid domain that binds to negatively charged phospholipid membranes in the presence of Ca2+ ions, along with two epidermal growth factor (EGF)-like domains (Furie and Furie, 1988; Mann et al., 1990; Brown et al., 2013). The heavy chain contains a trypsin-like serine protease domain (Padmanabhan et al., 1993; Kamata et al., 1998).
FXa is an arginine-specific serine protease that activates prothrombin by hydrolytic cleavage, forming thrombin (Rabiet et al., 1986; Mann et al., 1990; Brufatto and Nesheim, 2003; Brown et al., 2013). By directly contributing to the production of thrombin, it plays a key role in the process of blood clotting. FXa also interacts with cell-surface receptors, triggering responses such as cell activation, gene expression, and mitosis (Borensztajn et al., 2007, 2009). Components potentiating the effect of FXa and other coagulation factors, as well as those with FXa-like pro-coagulant activity, have been identified in the venoms of spiders such as Hippasa agelenoides (Devaraja et al., 2010), Lycosa singoriensis (Li et al., 2020a) and Acanthoscurria natalensis (Câmara et al., 2020). Given its proteolytic activity, FXa has been assigned as a putative spreading factor but may serve other, as of yet unknown activities in insect prey envenoming.
2.4 Precursor-activating enzymes
Spider venom components are typically expressed as propeptides or prepropeptides that undergo enzymatic cleavage during maturation. The signal peptide is removed during translocation through the endoplasmic reticulum membrane, whereas additional maturation steps are required to convert the propeptide into a biologically active mature molecule (Kozlov and Grishin, 2007; Kuhn-Nentwig et al., 2019). Precursor-activating enzymes are part of this machinery, which involves not only limited proteolysis but also C-terminal amidation and other posttranslational modifications (Kozlov and Grishin, 2007; Langenegger et al., 2018; Kuhn-Nentwig et al., 2019).
2.4.1 Dopamine β-monooxygenase
Dopamine β-monooxygenase (EC 1.14.17.1), also known as dopamine β-hydroxylase (DBH) or (3,4-dihydroxy-phenethylamine) β-monooxygenase, is a Cu-containing enzyme (Bairoch, 2000). Vertebrate DBH contains a conserved dopamine-binding domain (Ponting, 2001) and a Cu2_monoonxygen domain that converts dopamine to noradrenaline (Menniti et al., 1986; Klinman, 2006). The enzyme is glycosylated, contains disulfide bonds, and requires not only copper as a cofactor, but also l-ascorbate as an electron donor and molecular oxygen (Kobayashi et al., 1989; Lewis et al., 1990).
Vertebrate DBH is required for the biosynthesis of neurotransmitters such as catecholamine, dopamine, norepinephrine and epinephrine (Cheng et al., 2016). Norepinephrine is the major neurotransmitter in vertebrates, promoting wakefulness, regulating aggression, and controlling autonomic functions such as the heartbeat (Kim et al., 2002; Marino et al., 2005; Singh et al., 2015). The release of norepinephrine in both vertebrates and invertebrates during stress influences the function of the immune system (De Barros et al., 2012; Gallo et al., 2016). Specifically in arthropods, norepinephrine reduces phenoloxidase and superoxide dismutase (SOD) activity in the hemolymph of Penaeus vannamei (Li et al., 2020b). DBH has been found in the venom of three spiders: the ctenid Phoneutria nigriventer, the lamponid Lampona sp. and the theraphosid Acanthoscurria juruenicola (Diniz et al., 2018; Michálek et al., 2022; Nishiduka et al., 2022). The characteristics and associated functions of spider DBH proteins remain unknown.
2.4.2 Lysozyme
Lysozyme (EC 3.2.1.17), also known as muramidase, occurs as a monomer and is found in all living organisms (Bairoch, 2000; Leśnierowski and Yang, 2021). The three major families are the chicken-type (c-type), goose-type (g-type) and invertebrate-type (i-type) lysozymes (Leśnierowski and Yang, 2021). The c-type and i-type typically have a molecular weight of 11–15 kDa, whereas the g-type lysozymes are slightly larger with 20–22 kDa (Wu et al., 2019). Only the c-type lysozymes have been found in spider venom, specifically in Araneus ventricosus and Latrodectus tredecimguttatus (Duan et al., 2006, 2013).
The c-type lysozyme features 129 amino acids that fold into two domains linked by a long α-helix and stabilized by four disulfide bonds (Leśnierowski and Yang, 2021). The active site is located between the two domains and comprises six sugar-binding motifs defined as A, B, C, D, E and F (Leśnierowski and Yang, 2021). Monomeric lysozyme mainly catalyzes the hydrolysis of β-(1,4) bonds between N-acetylmuramic acid and N-acetyl-d-glucosamine in peptidoglycans, the major component of the bacterial cell wall (Leśnierowski and Yang, 2021). Therefore, lysozymes are mainly antimicrobial proteins that inhibit the growth of Gram-positive bacteria, but they are also considered as digestive enzymes and anti-inflammatory factors (Leśnierowski and Yang, 2021). Importantly, they are not present in the digestive fluids but in the venom of spiders, suggesting a role in precursor activation rather than digestion (Sanggaard et al., 2014; Fuzita et al., 2016; Valladão et al., 2023).
2.4.3 Peptidylglycine monooxygenase
Peptidylglycine monooxygenase (EC 1.14.17.3) is a bifunctional enzyme also known as peptidyl α-amidating enzyme, peptidylglycine 3-hydroxylase or peptidylglycine α-amidating monooxygenase (PAM) (Bairoch, 2000). PAMs have been found in four spider families: Eresidae (Stegodyphus mimosarum), Theraphosidae (three species), Theridiidae (two species), and Trechaleidae (Cupennius salei) (Sanggaard et al., 2014; Haney et al., 2019; Kuhn-Nentwig et al., 2019; Câmara et al., 2020; Dunbar et al., 2020; Nishiduka et al., 2022).
PAMs consist of two catalytic domains that activate precursor peptides in a two-step reaction (Figure 3). The first step is catalyzed by the peptidylglycine α-hydroxylating monooxygenase (PHM) domain, which hydroxylates the C-terminal glycine of a precursor peptide together with cofactors l-ascoborabate, molecular oxygen and Cu2+ (McIntyre et al., 2010). The second step involves the peptidylglycine amidoglycolate lyase (PAL) domain, which catalyzes the Zn2+-dependent dealkylation of carbinolamide intermediates (McIntyre et al., 2010). The PHM domain shares mechanistic, sequence and structural homology with DBH: both require two coordinated Cu+ ions for catalytic activity and transfer a hydrogen atom from the substrate to an activated Cu/O species, which results in the hydroxylation of the product (Ash et al., 1984; Kulathila et al., 1994). Posttranslational modifications catalyzed by PAM, such as C-terminal amidation, are often the final step in protein/peptide maturation (Vassilevski et al., 2009; Kuhn-Nentwig et al., 2019). These data suggest that PAMs are precursor activator enzymes in spider venom.
2.5 Preservative enzymes
Preservative enzymes help regulate oxidative stress, for example by eliminating reactive oxygen species (ROS) and reactive nitrogen species (RNS) (Colinet et al., 2011; Borges et al., 2016; Su et al., 2018; Zhang et al., 2020). ROS are metabolic intermediates or end products that directly mediate oxidative stress and peptide degradation. Venom components are sensitive to ROS, which reduce their half-life, so preservative enzymes are required to maintain venom efficacy (Peiren et al., 2008).
2.5.1 Peroxidase
Peroxidases (EC 1.11.1.-) form a large family of enzymes that can be divided into heme- and non-heme-containing members, each with multiple subclasses that utilize different substrates and electron donors (O’Brien, 2000). These enzymes break down phenolic compounds and remove peroxides, and are therefore components of many metabolic pathways as well as stress-response, defense and detoxification systems (De Oliveira et al., 2021). The catalytic center was originally proposed as a triad composed of Gln, Trp and Sec/Cys residues, but was later redefined as a tetrad with an additional Asn (Tosatto et al., 2008; Brigelius-Flohé and Maiorino, 2013).
Peroxidases have been found in the venom of Lampona sp. and Acanthoscurria geniculata (Sanggaard et al., 2014; Michálek et al., 2022) and may contribute to the preservation of toxins by protecting them from oxidative damage in the venom glands (De Oliveira et al., 2021; Delgado-Prudencio et al., 2022).
2.5.2 Superoxide dismutase
Superoxide dismutase (SOD, EC 1.15.1.1) is the main enzymatic regulator of ROS, protecting cells and tissues against oxidative stress by converting superoxide radicals to oxygen and hydrogen peroxide (Finkel and Holbrook, 2000; Serra et al., 2003). In most species, SOD is a homotetramer or-dimer of ~30 kDa subunits (Keele et al., 1971). SOD can be found inside cells but is also released into the ECM (Nozik-Grayck et al., 2005). They can be classified based on their metal cofactor requirements (Fe, Mn, or Cu/Zn), cellular location and fold, which leads to three distinct classes (Perry et al., 2010). Secreted SOD is usually connected to the ECM via the C-terminal region, which consists mostly of basic amino acids (Hjalmarsson et al., 1987; Bowler et al., 2002).
SOD has been found in the venom of three spider species, namely Phoneutria nigriventer, Tibellus oblongus and Acanthoscurria geniculata (Sanggaard et al., 2014; Diniz et al., 2018; Korolkova et al., 2023), where it may contribute to venom conservation (Delgado-Prudencio et al., 2022).
2.5.3 Carbonic anhydrase
Carbonic anhydrase (EC 4.2.1.1) is a zinc-dependent carbonate hydrolyase also known as carbonate dehydratase or carbonic dehydratase (Bairoch, 2000). Seven isoforms have been isolated from mammals, one of which is a membrane-bound protein while the others are free enzymes found in erythrocytes or in the mitochondrial matrix (Dodgson et al., 1991; Stams et al., 1996). Carbonic anhydrase II has one of the highest turnover numbers in nature (Dodgson et al., 1991; Stams et al., 1996). The human isoforms I, II, III and IV are homologous, with a β-sheet superstructure and an active site that is 15 Å deep, but the structure surrounding the active site differs between individual enzymes resulting in a 103-fold difference in catalytic activity (Figure 3) (Dodgson et al., 1991; Stams et al., 1996).
Carbonic anhydrase catalyzes the reversible reaction of hydrogen ions with hydrogen carbonate (HCO3-) to form carbon dioxide (CO2) and water (H2O) (Stams et al., 1996; Bairoch, 2000). In the spider Nephila clavipes, carbonic anhydrase regulates the pH gradient in the silk glands (Andersson et al., 2014). The enzyme is also found in the venom of the Brazilian tarantula Grammostola iheringi and is functionally annotated as a regulatory protein (Borges et al., 2016). Carbonic anhydrase is also found in the venom of Lampona sp. and Acanthoscurria sp (Sanggaard et al., 2014; Câmara et al., 2020; Michálek et al., 2022; Nishiduka et al., 2022).
2.5.4 Thioredoxin-dependent peroxidase
Thioredoxin-dependent peroxidase (EC 1.11.1.24), or thioredoxin peroxidase (Bairoch, 2000), is a ubiquitous cysteine-based peroxidase that exists as a 10–25 kDa monomer in its reduced state (Zhang et al., 2020) and functions as a highly efficient reductase (Reyes et al., 2016). It contains a highly conserved redox-active cysteine motif, which from the catalytic center to interact with peroxide substrate (Reyes et al., 2016; Zhang et al., 2020).
Thioredoxin-dependent peroxidases catalyze the conversion of hydroperoxide, alkyl hydroperoxides and peroxinitrite into water and corresponding alcohols (Sen, 2000; Su et al., 2018). Thioredoxin peroxidase has been found in the venom of a single theraphosid spider, Acanthoscurria geniculata (Sanggaard et al., 2014), where it may counter oxidative stress in the venom gland, thus contributing to venom conservation (Delgado-Prudencio et al., 2022).
2.6 Multifunctional enzymes
Venom enzymes described as multifunctional have been assigned several of the functions discussed above. For example, proteases are often multifunctional because they can facilitate the processing and activation of other venom components, diversify the toxin repertoire, and digest captured prey (Sarras, 1996; Borges et al., 2016). The majority of venom enzymes may have multiple functions, enhancing their ecological significance.
2.6.1 Phospholipase A2
Phospholipase A2 (PLA2, EC 3.1.1.4), also known as lecithinase A, phosphatidase, phosphatidolipase, and phosphatidylcholine 2-acylhydrolase, is a common venom component in its secretory form, sPLA2 (Kini, 2003; Dennis et al., 2011; Kuhn-Nentwig et al., 2011). The protein typically has a molecular weight of 14–18 kDa, features 6–8 disulfide bonds, and often requires Ca2+ as a cofactor. Many sPLA2 proteins feature three elongated α-helices and two antiparallel β-sheets (often described as β-wings) stabilized by disulfide bonds, and a conserved Ca2+ binding loop (Dennis et al., 2011). However, some sPLA2 lack β-wings, contain only α-helical motifs or enzymatically fully inactive (Matoba et al., 2002; Guy et al., 2009; Harris and Scott-Davey, 2013).
Secreted PLA2 enzymes catalyze the hydrolysis of phospholipids at the glycerin β-C-atom. They are prominent constituents of many snake venoms (Kini, 2003; Murakami et al., 2011) and have multiple physiological effects, including neurotoxic symptoms (Wernicke et al., 1975; Ritonja and Gubenek, 1985), the inhibition of blood coagulation (Boffa and Boffa, 1976), and the modulation of platelet functions (Landucci et al., 1994). In spider venoms, PLA2 enzymes are thought to promote coagulopathy (Usmanov and Nuritova, 1994), cell lysis and apoptosis (Kuhn-Nentwig et al., 2011). Such enzymes have been found in the venoms of seven spiders (Dresler et al., 2024), including Hylyphantes graminicola, Physocyclus mexicanus and Cupiennius salei (Zobel-Thropp et al., 2018; Kuhn-Nentwig et al., 2019; Zhu et al., 2022), but their functions have yet to be characterized in detail.
2.6.2 S10 serine carboxypeptidase family
The S10 serine carboxypeptidase family (EC 3.4.16.-) consists of the carboxypeptidases C and D, which are the only serine peptidases active at acidic pH (Rawlings and Salvesen, 2013c). Their secondary structure features β-strands and α-helices bracketing the three residues of the active site. The tertiary structure is unlike any other serine peptidase, mainly consisting of parallel β-sheets forming β/α/β units (α/β-hydrolase fold). Both serine carboxypeptidases require serine as a cofactor but differ in their specificity, mirroring the specificity of M14 metallocarboxypeptidases (Rawlings and Salvesen, 2013c). Serine carboxypeptidases have been found in spider venom (see 2.6.2.1 and 2.6.2.2) (Hayashi et al., 1973; Rawlings and Salvesen, 2013c). They are involved in multiple physiological and cellular processes, ranging from protein digestion in animals to the mobilization of seed storage proteins in plants, and the post-translational processing of other enzymes (Mikola, 1986; Galjart et al., 1990; Bown et al., 1998; Valladão et al., 2023). Based on these diverse activities, serine carboxypeptidases in spider venom may also fulfil multiple functions, although more work is required to achieve experimental confirmation.
2.6.2.1 Carboxypeptidase C
The carboxypeptidase C (EC 3.4.16.5) is also known as carboxypeptidase Y, cathepsin A, lysosomal protective protein and serine-type carboxypeptidase I (Bairoch, 2000; Rawlings and Salvesen, 2013a). The best-characterized member is yeast carboxypeptidase Y, a single-chain 65 kDa protein glycosylated at four positions (Johansen et al., 1976; Winther et al., 1991). It is produced as a prepropeptide that is modified during maturation (Bairoch, 2000; Rawlings and Salvesen, 2013c). Carboxypeptidase C has been found in the venom of the spiders Argiope bruennichi and Lampona sp (Lüddecke et al., 2020; Michálek et al., 2022). The ancestral enzyme might have been essential for the correct assembly and function of a proteins containing β-galactosidase and neuraminidase in the lysosomal compartment (Rawlings and Salvesen, 2013c). In spider venom, carboxypeptidase C may activate precursors by processing their C-termini and play a role in pre-digestion as it is also found in the digestive fluids of Nephilengis cruentata (Fuzita et al., 2016).
2.6.2.2 Carboxypeptidase D
Carboxypeptidase D (EC 3.4.16.6) is also known as carboxypeptidase KEX1 or carboxypeptidase S1. It is exclusively found in eukaryotes and is ubiquitous in higher organisms (Rawlings and Salvesen, 2013c). It preferentially cleaves C-terminal arginine or lysine residues (Xin et al., 1997; Bairoch, 2000; Rawlings and Salvesen, 2013c). The enzyme is typically a 50–70 kDa glycoprotein with one or two chains interconnected by disulfide bonds (Rawlings and Salvesen, 2013c). In plants and mammals, this exopeptidase is broadly distributed in many tissues and is thought to process secreted proteins (Xin et al., 1997) and peptides (Galjart et al., 1990; Lehfeldt et al., 2000; Rawlings and Salvesen, 2013c). Carboxypeptidase D has been detected in the venom of the spiders Stegodyphus mimosarum, Acanthoscurria geniculata and Parasteatoda tepidariorum (Sanggaard et al., 2014; Haney et al., 2019).
2.6.3 M14 metallocarboxypeptidase family
The M14 metallocarboxypeptidase family (EC 3.4.17.-) includes carboxypeptidases A, B, M and E, as well as bacterial carboxypeptidase T and γ-d-glutamyl-(l)-meso-diaminopilmelate peptidase I (Rawlings and Salvesen, 2013a). The M14 family is partially subdivided into subfamilies. Subfamily M14A contains carboxypeptidases A and B, which in mammals are digestive enzymes, while subfamily M14B comprises a number of carboxypeptidases, including carboxypeptidases E, which are regulatory proteins that determine the availability of peptide mediators and hormones (Rawlings and Salvesen, 2013a). Although most members of the M14 family are soluble, carboxypeptidase M is a membrane-bound enzyme. All M14 peptidases feature an α/β/α sandwich with an antiparallel β-sheet of eight strands, similar to the α/β hydrolases, including the S10 family mentioned above (Rawlings and Salvesen, 2013a). These enzymes require a Zn2+ ion that is tetrahedrally coordinated by a water molecule, two histidine residues and glutamate (Rawlings and Salvesen, 2013a). M14 peptidases in spider venom are yet to be validated on functional level and could belong to several subfamilies with diverse functions.
2.6.3.1 Carboxypeptidase A
Carboxypeptidase A (EC 3.4.17.1), also known as carboxypolypeptidase (Bairoch, 2000; Kuhn-Nentwig et al., 2019) is produced as an inactive propeptide. The crystal structure of monomeric porcine procarboxypepdiase A1 consists of two domains: a N-terminal 95-amino-acid propeptide activation segment and a 308-amino-acid enzyme domain (Guasch et al., 1992). The monomeric enzyme is found in most species, but the proenzyme sometimes occurs as a binary or ternary complex with other proteases (Vendrell et al., 2000). Carboxypeptidase A catalyzes the cleavage of C-terminal peptide or ester bonds (Davies et al., 1968). Physiologically, it is an important exopeptidase involved in the degradation of diet proteins (Rawlings and Salvesen, 2013a). Carboxypeptidase A has been found in the venom of the spiders Acanthoscurria natalensis and Cupiennius salei, where it may be involved in pre-digestion and precursor activation (Kuhn-Nentwig et al., 2019; Câmara et al., 2020).
2.6.3.2 Carboxypeptidase B
Carboxypeptidase B (EC 3.4.17.2), also known as protaminase (Bairoch, 2000; Barrett et al., 2012), is structurally similar to the carboxypeptidase A (Villegas et al., 1995; Bairoch, 2000; Barrett et al., 2012) and features conserved Zn-coordinated residues (Avilés et al., 1993). In humans, the enzyme consists of an activation and an enzyme domain, including a 94-amino-acid N-terminal propeptide that functions as a chaperone to assist with folding (Billeter et al., 1992; Rawlings and Salvesen, 2013a). Carboxypeptidase B is an exopeptidase with moderate affinity for C-terminal, non-basic amino acids such as arginine, valine, leucine and isoleucine, among others (Nishihira et al., 1995; Villegas et al., 1995; Bairoch, 2000). This enzyme is important for the digestion of food (Barrett et al., 2012) but also breaks down effector molecules (Villegas et al., 1995). It has been found in the venom of the spider Parasteatoda tepidariorum and, like carboxypeptidase A, may be involved in pre-digestion and precursor activation (Haney et al., 2019).
2.6.3.3 Carboxypeptidase E
Carboxypeptidase E (EC 3.4.17.10), also known as carboxypeptidase H or enkephalin convertase (Fricker, 1988; Bairoch, 2000), is closely related to carboxypeptidases A, B and D and may share the amino acids required for catalytic activity and substrate binding (Fricker et al., 1986; Aloy et al., 2001). It consists of a ~300-amino-acid carboxypeptidase A/B-like and an ~80-amino-acid transthyretin-like domain, both of which are present in all members of the carboxypeptidase E subfamily (Rawlings and Salvesen, 2013a). In contrast to other metallocarboxypeptidases, carboxypeptidase E contains a unique C-terminal region of ~40 amino acids required for pH-dependent peripheral association with membranes (Varlamov and Fricker, 1996). In mammals, this enzyme has a high specificity for the release of C-terminal arginine or lysine residues from polypeptides, and needs Co+ as a cofactor. It is associated with the biosynthesis of peptidic neurotransmitters and hormones (Fricker, 1988). The evolutionary relationship between carboxypeptidases E and B suggests that both proteins were originally digestive enzymes (Fricker, 1988). Carboxypeptidase E has been found in the venom of Acanthoscurria juruenicola and may, like its relatives described above, be involved in pre-digestion and precursor activation (Nishiduka et al., 2022).
2.6.3.4 Carboxypeptidase M
Carboxypeptidase M (EC 3.4.17.12) is the only membrane-bound member of the M14 peptidase family. The human enzyme is a single polypeptide chain with a molecular weight of 62 kDa (Skidgel et al., 1989). It consists of an N-terminal catalytic domain and a GPI membrane anchor attached to the C-terminal extension of the protein (Blake and Oatley, 1977; Stams et al., 1996; Reverter et al., 2004). Carboxypeptidase M catalyzes the C-terminal hydrolysis behind of arginine or lysine residues from peptides such as bradykinin and kallidin and controls peptide hormone activity at the cell surface, process extracellular proteins and process extracellular prohormones (Skidgel, 1988; Skidgel and Erdös, 1998), as well as facilitating the inflammatory response by activating inflammatory mediators such as bradykinin and kallidin (Bhoola et al., 1992; Leeb-Lundberg et al., 2005). It has been detected only once in spider venom, in Tetragnatha versicolor (Zobel-Thropp et al., 2018). The structure of carboxypeptidase M in spider venom is unknown, so it may be a soluble protein without a GPI anchor and may be involved in precursor activation and pre-digestion.
2.6.4 Serine peptidases
Serine peptidases (EC 3.4.21.-), also known as S1 serine proteases, are endopeptidases characterized by conserved catalytic triad comprising residues of histidine and aspartate and the name giving serine (Bairoch, 2000; Hedstrom, 2002). They have a distinctive structure, typically featuring a single domain with a “chymotrypsin fold” consisting of several α-helices and β-sheets in different arrangements (Hedstrom, 2002). Their classification as trypsin-like, chymotrypsin-like and elastase-like enzymes is based on substrate specificity (Ovaere et al., 2009). S1 proteases disrupt the physiology of envenomed prey by interfering with multiple processes (Devaraja et al., 2011), thus enhancing the overall efficacy and potency of the venom (Sannaningaiah et al., 2014; Langenegger et al., 2019) or promoting toxin maturation (Langenegger et al., 2018).
S1 serine peptidases have been identified in many spider venoms, including Argiope bruennichi, Phoneutria nigriventer and Tibellus oblongus (Diniz et al., 2018; Lüddecke et al., 2020; Korolkova et al., 2023), but have yet to be classified to a specific enzyme. Furthermore, trypsin homologs (EC 3.4.21.4) have been identified in the venom of Acanthoscurria geniculate, Steatoda nobilis, Cyriopagopus schmidti and Lampona sp (Yuan et al., 2007; Sanggaard et al., 2014; Dunbar et al., 2020; Michálek et al., 2022). This enzyme has a molecular weight of 23 kDa and has different activated forms mainly α-trypsin and β-trypsin (Bartunik et al., 1989). Trypsin cleaves highly specific the peptide bond C-terminal of the positive charged amino acids lysine and arginine (Olsen et al., 2004). In addition to its digestive function, trypsin contributes to physiological processes such as the regulation of immunity, blood coagulation, and tissue remodeling through the activation of protease-activated receptors and the modulation of signaling pathways (Yin, 1964; Ossovskaya and Bunnett, 2004; Wang et al., 2008).
2.6.5 Neprilysin
Neprilysin (EC 3.4.24.11), also known as endopeptidase-2, enkephalinase, kidney-brush-border neutral protease, membrane metalloendopeptidase and neutral endopeptidase (Bairoch, 2000), is a 70kDa zinc metalloendopeptidase with several disulfide bonds and predicted glycosylation sites (Turner et al., 2001). Like many other metallopeptidases, neprilysin is ubiquitous in mammals and cleaves a broad range of targets, including pain-regulating enkephalins, tachykinin neuropeptides, and proteins involved in tissue development (Nalivaeva et al., 2020). Although this enzyme is often found as a membrane-bound glycoprotein, it is also present (presumably without a membrane anchor) in venoms (Do Nascimento et al., 2022). It has been detected in the venoms of multiple spiders, including Hadronyche sp., Trittame loki, Acanthoscurria sp (Undheim et al., 2013; Abreu et al., 2017; Câmara et al., 2020; Cardoso et al., 2022; Nishiduka et al., 2022). and several others (Dresler et al., 2024).
In spider venom, it may facilitate the spreading of other venom components by breaking up proteins in the ECM, or it may be involved in the paralysis of envenomated prey by degrading peptide neurotransmitters and neuromodulators (Casewell et al., 2009; Undheim et al., 2013; Liu et al., 2015; Zobel-Thropp et al., 2019; Yoon et al., 2020). In the venom of the mygalomorph spider Avicularia juruensis, Neprilysins showed caseinolytic activity, supporting its role as a spreading factor (Do Nascimento et al., 2022).
2.6.6 Astacin
Astacin (EC 3.4.24.21), also known as astacus protease and crayfish small-molecule protease (Bairoch, 2000), is a small (20–30 kDa) metalloprotease from the M12 family, featuring a single zinc-dependent metallopeptidase domain, intramolecular disulfide bonds and several predicted glycosylation sites (Trevisan-Silva et al., 2010; Gremski et al., 2021; Gomis-Rüth and Stöcker, 2023). Astacins have been found in the venoms of the spiders Argiope bruennichi, Phoneutria nigriventer and Loxosceles sp (Trevisan-Silva et al., 2010; Lüddecke et al., 2020; Cardoso et al., 2022). among others (Dresler et al., 2024). Loxosceles astacin breaks down ECM components such as fibronectin, fibrinogen and gelatin, potentially acting as spreading factors to enhance the systemic effect of toxins (da Silveira et al., 2007; Gremski et al., 2021). Astacins also digest the proteins of captured prey, facilitating the process of liquefaction (Gremski et al., 2021; Gomis-Rüth and Stöcker, 2023). Selected spider venom Astacin sequences are illustrated in Figure 4.
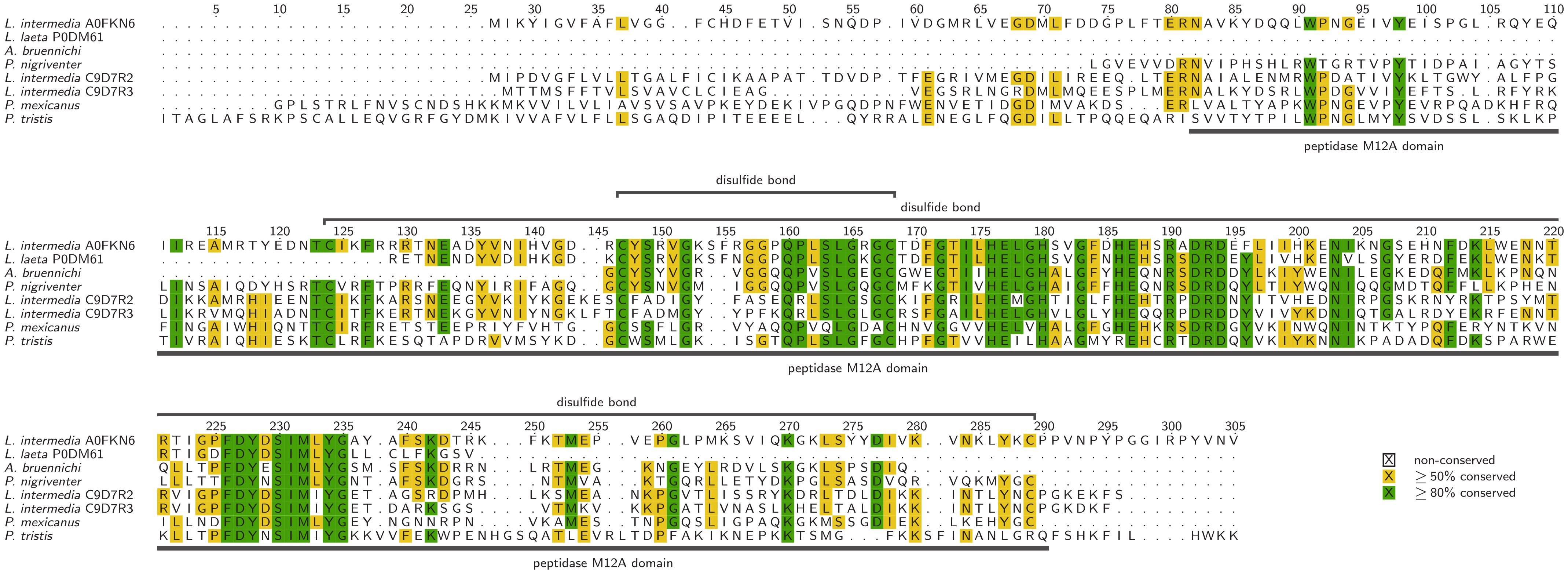
Figure 4. Alignment of selected astacins, identified in spider venom with additional data corresponding to the UniProt entries. Sequences lacking an UniProt identifier have been described in Zobel-Thropp et al., 2014, 2019; Lüddecke et al., 2020, and Cardoso et al., 2023. Alignments were visualized with TEXshade in identical mode (Beitz, 2000).
2.7 Venom enzymes without functional assignment or with unclear enzymatic activities
In addition to the spider venom enzymes that have been functionally classified based on knowledge from other arachnids, many additional, unclassified enzymes were identified that show similarity to venom components from other animals (Dresler et al., 2024). Future work should focus on the functional analysis of these molecules to shed light on the ecology of spider venoms.
For example, phospholipase A1 (PLA1) proteins (EC 3.1.1.32) are widely distributed across metazoan and protozoan organisms, and are often regarded as ubiquitous, although their functions are not clearly understood (Richmond and Smith, 2011). PLA1 proteins have been found in the venom of the spider Chilobrachys guangxiensis, but their targets and overall role are still unknown (Liao et al., 2007). Reprolysin (EC 3.4.24.-), another member of the M12 metallopeptidase family, is also occasionally found in spider venoms. These enzymes are dependent on a catalytic Zn2+ ion and are stabilized by disulfide bonds (Rawlings and Salvesen, 2013b). The related enzyme astacin (see section 2.6.6) belongs to the M12A subfamily, whereas reprolysin represents the M12B subfamily (Bjarnason and Fox, 1994). Interestingly, not all reprolysins are active proteases (Rawlings and Salvesen, 2013b). They have multiple domains, including C-terminal disintegrin, EGF-like, cysteine-rich, and transmembrane domains (Rawlings and Salvesen, 2013b). In snake venoms, these added domains can act as independent toxins after maturation (Olaoba et al., 2020). One reprolysin has been detected in the venom of the spider Hippasa partita (Nagaraju et al., 2007). As components of snake and spider venoms, reprolysins can proteolytically degrade ECM components, inducing hemorrhage and tissue necrosis (Zigrino et al., 2002; Fox and Serrano, 2005; Nagaraju et al., 2007). Reprolysin may therefore act as a spreading factor in spider venom, and/or facilitate the pre-digestion of prey.
Besides known enzymatic components, a range of biomolecules is known to occur in spider venoms that are awaiting clarification of their potential enzymatic activities. This specifically includes two protein groups, namely CAP proteins and lectins. Both are generally not enzymatic, but some of them display strong similarity to known enzymes or, in rare cases, include enzymatic isoforms.
The CAP superfamily contains Cysteine-rich secretory proteins (CRISPs), as well as Antigen 5 and Pathogenesis-related protein 1, and is commonly found in animal venoms (Gibbs et al., 2008; Kuhn-Nentwig et al., 2019). Outside the conserved region, the structure of CAPs is diverse, as is their target specificity and therefore their biological function (Gibbs et al., 2008). CAPs have been detected in the venom of multiple spiders (Dresler et al., 2024) including Lycosa singoriensis, Trittame loki and Argiope bruennichi (Zhang et al., 2010; Undheim et al., 2013; Lüddecke et al., 2020). The venom of the wasp spider Argiope bruennichi contains CAP proteins that are similar to proteolytic cone snail CAPs and lack the C-terminal cysteine-rich domain that correlates with neurotoxic activity in snake venom CAPs (Lüddecke et al., 2020). Based on this similarity with a putatively enzymatic CAP from Conus textile, some spider venom CAPs are speculated to be proteases as well (Lüddecke et al., 2020). However, so far none of these proteins has ever been functionally characterized. Based on their putative proteolytic activity, the CAP superfamily in spider venom may degrade prey enzymes, act as a spreading factor, contribute to prey digestion, and/or facilitate precursor activation, as well as trigger allergic effects after envenomation.
The other important group, the lectin family, is a widely-studied superfamily of carbohydrate-binding glycoproteins (Manning et al., 2017; Chettri et al., 2021). They can be classified by source, localization, carbohydrate-binding specificity, number of binding sites, sequence, structure, and evolutionary similarity (Chettri et al., 2021). In functional terms, these compounds enable cell–environment interactions by binding to carbohydrate structures on the cell surface, for transport or for storage (Berg et al., 2002; Santos et al., 2014; Nizet et al., 2015; Chettri et al., 2021). Unlike other carbohydrate-binding proteins, lectins have the unique ability to agglutinate cells (Mel’nikova et al., 2000; Olsnes and Kozlov, 2001; Manning et al., 2017). Most lectins have a single carbohydrate-binding domain, but some bacterial and plant lectins have an additional enzymatic domain linked by a disulfide bond (Mel’nikova et al., 2000; Olsnes and Kozlov, 2001; Manning et al., 2017). Other polyvalent glycosidases can agglutinate cells under certain conditions and are therefore considered to “act as a lectin” (Barondes, 1981). Lectins have been identified in five spider venoms (Dresler et al., 2024), including species of the genera Phoneutria and Acanthoscurria (Sanggaard et al., 2014; Diniz et al., 2018; Nishiduka et al., 2022). In snake venoms two groups with robust C-type lectin domains (CTLD) are known, the sugar-binding C-type lectins (CTLs) and the second group lacking sugar-binding capabilities C-type lectin-related proteins, referred as CLRPs or snaclecs (Sartim and Sampaio, 2015; Eble, 2019). This toxin class form supramolecular structures up to heterooctameric (αβ)4 complexes and even larger, as known from convulxin and has a highly promiscuous ligand spectrum (Eble, 2019). They target various receptors and clotting factors, e.g. glycoprotein (GP) Ib, integrin α2β1, and von Willebrand factor, with a strong translational potential, like the GPIb-blocking SV-CLRP agkicetin (anfibatide) from Agkistridon acutus (Eble, 2019). As with the CAP proteins described above, the presence of catalytically active sites must be validated before we can confirm that these putative venom components are functional enzymes.
3 Spider venom enzymes as catalysts for bioeconomic innovation
Spiders are one of the most prolific sources of venom-derived bioactive molecules suitable for a wide range of applications (Saez et al., 2010; King and Hardy, 2013). Based on the potent and highly selective interaction with targets in the central nervous system, spider toxins have been studied intensively as drug leads and agrochemical candidates to combat insect pests (King and Hardy, 2013). The recent discovery of diverse enzymes in spider venoms adds a novel dimension to their translational potential in the bioeconomy. Enzymes are widely used in the pharmaceutical, analytical, and food and feed industries. For example, they are employed as catalysts for the synthesis of biopolymers, for waste treatment, or as detergents (Singh et al., 2016; Mesbah, 2022). Due to their high selectivity, low by-product formation, and eco-friendly characteristics, enzyme utilization has increased over the years. Consequently, the global market for enzymes with specialized applications is experiencing rapid growth (Singh et al., 2016).
Venom enzymes are active under diverse environmental conditions, and catalyze rapid reactions to prevent the prey from escaping or retaliating. These are desirable properties for industrial applications (Lorenz and Eck, 2005). Chitinases, serine peptidases and astacins are already used in the pharmaceutical, food processing, and plant protection industries (Puente et al., 2005; Trevisan-Silva et al., 2010; Rathore and Gupta, 2015; Chakraborty et al., 2017). For example, spider chitinases break down the chitin in fungal cell walls, insect exoskeletons and crustacean shells, and could therefore, similar to other chitinases, be used to protect plants against fungal diseases and insect pests, and to convert shellfish waste into useful products (Rathore and Gupta, 2015). The toxic enzyme phospholipase D could be used as a positive control reagent in laboratory protocols investigating the aggregation of platelets, in hemolytic assays, or as a model to study responses to anti-inflammatory and endothelial-modulating drug candidates (Senff-Ribeiro et al., 2008). Many spider venom enzymes serve digestive purposes and belong to classes used within the food and feed industry, such as lipases, amylases, proteases, and α-galactosidases. These enzymes improve the digestibility and nutritional value of various products including bread, beer, and fruit juices (Singh et al., 2016). Detergents for dishwashing, laundry and industrial cleaning make up almost 30% of sales in the industrial enzyme sector, by far the largest segment of this market (Singh et al., 2016). Most such enzymes are hydrolases (e.g., proteases, amylases, lipases) that are used to remove protein, starch, oil, and fat stains or food particles, reducing the use of phosphates and bleaching agents, which are harmful to the public and environment (Singh et al., 2016; Mesbah, 2022). Pre-digestive enzymes from spider venom may be of interest for waste management, the upcycling of industrial side streams, or as detergents. Furthermore, hyaluronidases and FXa homologs could be used to develop pharmacological assays (Borensztajn et al., 2007, 2009; Senff-Ribeiro et al., 2008). Precursor-activating enzymes such as some carboxypeptidases, DBH and PAM may be useful in bioassays or biochemical reactions (Menniti et al., 1986; Klinman, 2006; McIntyre et al., 2010). FXa homologs and the carboxypeptidases A and Y, for instance, are already applied for the cleavage of fusion proteins (Jenny et al., 2003; Wu et al., 2022). Preservative enzymes such as peroxidases are already used in the textile industry as antimicrobial agents or to remove excess dye (Kirk et al., 2002), whereas carbonic anhydrases are used to remove CO2 in industrial processes including carbon sequestration and biofuel production (González and Fisher, 2014). The sourcing of robust, specific, and fast-acting enzymes from spider venoms representing all of the industrial categories discussed above could therefore help improve existing industrial processes and develop new strategies for the recycling of industrial side streams and waste. Figure 5 illustrates the potential areas of application versus those of traditionally investigated components.
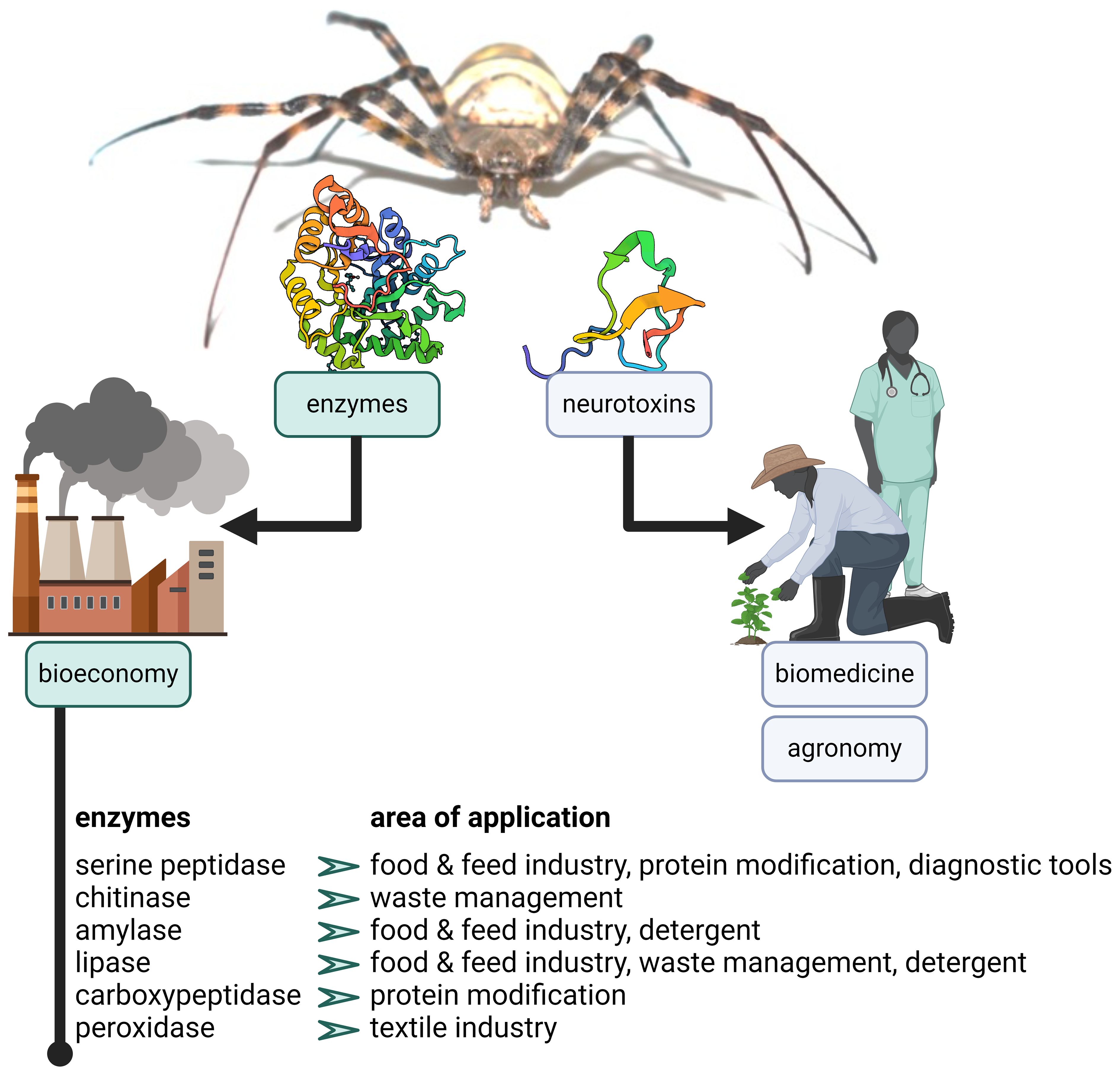
Figure 5. The value of spider venom enzymes. The image depicts the areas of application of traditionally investigated spider venom neurotoxins versus those of enzymes. For selected enzymatic classes, examples of potential areas of application are outlined.
4 Conclusion
This review provides the first systematic overview of spider venom enzymes and their potential functions in venom systems. Following the classification available for other arachnids (Delgado-Prudencio et al., 2022; Dresler et al., 2024), we have described the 34 of 144 families of enzymes that could be assigned to potential venom functions. With the exception of phospholipase D, which has been studied extensively due to its clinical relevance, spider venom enzymes have been largely overlooked by the industrial and research communities. In addition to their natural functions, these enzymes may have the potential to act in diverse industrial applications including waste management, cleaning, plant protection, and pharmaceutical assays. The experimental validation of structure and functions of such enzymes will not only improve our understanding of their natural functions and ecological roles, but also facilitate the development of novel enzyme-based products and processes to enhance the bioeconomy.
Author contributions
JD: Data curation, Investigation, Project administration, Visualization, Writing – original draft. IA: Data curation, Investigation, Writing – original draft. MD: Data curation, Investigation, Writing – original draft. LD: Data curation, Investigation, Writing – original draft. JK: Data curation, Investigation, Writing – original draft. AV: Conceptualization, Funding acquisition, Project administration, Writing – original draft. TL: Conceptualization, Project administration, Resources, Supervision, Writing – original draft.
Funding
The author(s) declare financial support was received for the research, authorship, and/or publication of this article. This work was funded by the Hessian Ministry of Higher Education, Research and the Arts via the LOEWE Center for Translational Biodiversity Genomics granted to AV. MD was funded by the Deutsche Forschungsgemeinschaft (DFG; ref. 540833593).
Acknowledgments
The authors are grateful to the Editorial Board of Frontiers in Arachnid Science, and in particular to Volker Herzig, for the generous invitation to include this article in the horizon series. We also thank Sabine Hurka for her bioinformatic support in data visualization and Richard M. Twyman for manuscript language editing.
Conflict of interest
The authors declare that the research was conducted in the absence of any commercial or financial relationships that could be construed as a potential conflict of interest.
The author(s) declared that they were an editorial board member of Frontiers, at the time of submission. This had no impact on the peer review process and the final decision.
Publisher’s note
All claims expressed in this article are solely those of the authors and do not necessarily represent those of their affiliated organizations, or those of the publisher, the editors and the reviewers. Any product that may be evaluated in this article, or claim that may be made by its manufacturer, is not guaranteed or endorsed by the publisher.
Supplementary material
The Supplementary Material for this article can be found online at: https://www.frontiersin.org/articles/10.3389/frchs.2024.1445500/full#supplementary-material
References
Abramson J., Adler J., Dunger J., Evans R., Green T., Pritzel A., et al. (2024). Accurate structure prediction of biomolecular interactions with AlphaFold 3. Nature 630, 493–500. doi: 10.1038/s41586-024-07487-w
Abreu T. F., Sumitomo B. N., Nishiyama M. Y., Oliveira U. C., Souza G. H. M. F., Kitano E. S., et al. (2017). Peptidomics of Acanthoscurria gomesiana spider venom reveals new toxins with potential antimicrobial activity. J. Proteom 151, 232–242. doi: 10.1016/j.jprot.2016.07.012
Ahmadi S., Knerr J. M., Argemi L., Bordon K. C. F., Pucca M. B., Cerni F. A., et al. (2020). Scorpion Venom: detriments and benefits. Biomedicines 8, 118. doi: 10.3390/biomedicines8050118
Aird S. D. (2002). Ophidian envenomation strategies and the role of purines. Toxicon 40, 335–393. doi: 10.1016/S0041-0101(01)00232-X
Aloy P., Companys V., Vendrell J., Aviles F. X., Fricker L. D., Coll M., et al. (2001). The crystal structure of the inhibitor-complexed carboxypeptidase D domain II and the modeling of regulatory carboxypeptidases. J. Biol. Chem. 276, 16177–16184. doi: 10.1074/jbc.M011457200
Andersson M., Chen G., Otikovs M., Landreh M., Nordling K., Kronqvist N., et al. (2014). Carbonic anhydrase generates CO2 and H+ that drive spider silk formation via opposite effects on the terminal domains. PloS Biol. 12, e1001921. doi: 10.1371/journal.pbio.1001921
Anisha G. S. (2017). “α-galactosidases,” in Current Developments in Biotechnology and Bioengineering (Amsterdam, Netherlands: Elsevier), 369–394. doi: 10.1016/B978-0-444-63662-1.00016-6
Anliker B., Chun J. (2004). Lysophospholipid G pprotein-coupled receptors. J. Biol. Chem. 279, 20555–20558. doi: 10.1074/jbc.R400013200
Ash D. E., Papadopoulos N. J., Colombo G., Villafranca J. J. (1984). Kinetic and spectroscopic studies of the interaction of copper with dopamine β-hydroxylase. J. Biol. Chem. 259, 3395–3398. doi: 10.1016/S0021-9258(17)43105-X
Avilés F. X., Vendrell J., Guasch A., Coll M., Huber R. (1993). Advances in metallo-procarboxypeptidases: emerging details on the inhibition mechanism and on the activation process. Eur. J. Biochem. 211, 381–389. doi: 10.1111/j.1432-1033.1993.tb17561.x
Bairoch A. (2000). The ENZYME database in 2000. Nucleic Acids Res. 28, 304–305. doi: 10.1093/nar/28.1.304
Barbaro K. C., Sousa M. V., Morhy L., Eickstedt V. R. D., Mota I. (1996). Compared chemical properties of dermonecrotic and lethal toxins from spiders of the genus Loxosceles (Araneae). J. Protein Chem. 15, 337–343. doi: 10.1007/BF01886859
Barnard E. A., Dolly J. O., Porter C. W., Albuquerque E. X. (1975). The acetylcholine receptor and the ionic conductance modulation system of skeletal muscle. Exp. Neurol. 48, 1–28. doi: 10.1016/0014-4886(75)90219-8
Barondes S. H. (1981). Lectins: their multiple endogenous cellular functions. Annu. Rev. Biochem. 50, 207–231. doi: 10.1146/annurev.bi.50.070181.001231
Barrett A. J., Rawlings N. D., Woessner J. F., Woessner J. F. (2012). Handbook of proteolytic enzymes (San Diego: Elsevier Science & Technology).
Bartunik H. D., Summers L. J., Bartsch H. H. (1989). Crystal structure of bovine β-trypsin at 1.5 Å resolution in a crystal form with low molecular packing density: active site geometry, ion pairs and solvent structure. J. Mol. Biol. 210, 813–828. doi: 10.1016/0022-2836(89)90110-1
Beitz E. (2000). TeXshade: shading and labeling of multiple sequence alignments using LaTeX2e. Bioinformatics 16, 135–139. doi: 10.1093/bioinformatics/16.2.135
Benzertiha A., Kierończyk B., Rawski M., Józefiak A., Kozłowski K., Jankowski J., et al. (2019). Tenebrio molitor and Zophobas morio full-fat meals in broiler chicken diets: effects on nutrients digestibility, digestive enzyme activities, and cecal microbiome. Animals 9, 1128. doi: 10.3390/ani9121128
Berg J. M., Tymoczko J. L., Stryer L. (2002). “Lectins are specific carbohydrate-binding proteins,” in Biochemistry (Freeman, New York), 333–335.
Bhattacharya D., Nagpure A., Gupta R. K. (2007). Bacterial chitinases: properties and potential. Crit. Rev. Biotechnol. 27, 21–28. doi: 10.1080/07388550601168223
Bhoola K. D., Figueroa C. D., Worthy K. (1992). Bioregulation of kinins: kallikreins, kininogens, and kininases. Pharmacol. Rev. 44, 1–80.
Billeter M., Vendrell J., Wider G., Avilés F. X., Coll M., Guasch A., et al. (1992). Comparison of the NMR solution structure with the X-ray crystal structure of the activation domain from procarboxypeptidase B. J. Biomol NMR 2, 1–10. doi: 10.1007/BF02192796
Biner O., Trachsel C., Moser A., Kopp L., Langenegger N., Kämpfer U., et al. (2015). Isolation, N-glycosylations and function of a hyaluronidase-like enzyme from the venom of the spider Cupiennius salei. PloS One 10, e0143963. doi: 10.1371/journal.pone.0143963
Binford G. J., Bodner M. R., Cordes M. H. J., Baldwin K. L., Rynerson M. R., Burns S. N., et al. (2009). Molecular evolution, functional variation, and proposed nomenclature of the gene family that includes sphingomyelinase D in sicariid spider venoms. Mol. Biol. Evol. 26, 547–566. doi: 10.1093/molbev/msn274
Bjarnason J. B., Fox J. W. (1994). Hemorrhagic metalloproteinases from snake venoms. Pharmacol. Therapeut 62, 325–372. doi: 10.1016/0163-7258(94)90049-3
Blake C. C. F., Oatley S. J. (1977). Protein–DNA and protein–hormone interactions in prealbumin: a model of the thyroid hormone nuclear receptor? Nature 268, 115–120. doi: 10.1038/268115a0
Blaxter M. (1996). Protein motifs in filarial chitinases. Parasitol. Today 12, 42. doi: 10.1016/0169-4758(96)80647-8
Boffa M.-C., Boffa G. A. (1976). A phospholipase A2 with anticoagulant activity II. Inhibition of the phospholipid activity in coagulation. Biochim. Biophys. Acta Enzymol. 429, 839–852. doi: 10.1016/0005-2744(76)90330-2
Bolognesi R., Arakane Y., Muthukrishnan S., Kramer K. J., Terra W. R., Ferreira C. (2005). Sequences of cDNAs and expression of genes encoding chitin synthase and chitinase in the midgut of Spodoptera frugiperda. Insect Biochem. Mol. Biol. 35, 1249–1259. doi: 10.1016/j.ibmb.2005.06.006
Bordon K. C. F., Wiezel G. A., Amorim F. G., Arantes E. C. (2015). Arthropod venom hyaluronidases: biochemical properties and potential applications in medicine and biotechnology. J. Venom Anim. Toxins Incl Trop. Dis. 21, 43. doi: 10.1186/s40409-015-0042-7
Borensztajn K. S., Bijlsma M. F., Groot A. P., Brüggemann L. W., Versteeg H. H., Reitsma P. H., et al. (2007). Coagulation factor Xa drives tumor cells into apoptosis through BH3-only protein bim up-regulation. Exp. Cell Res. 313, 2622–2633. doi: 10.1016/j.yexcr.2007.04.014
Borensztajn K., Bijlsma M. F., Reitsma P. H., Peppelenbosch M. P., Spek C. A. (2009). Coagulation factor Xa inhibits cancer cell migration via protease-activated receptor-1 activation. Thromb. Res. 124, 219–225. doi: 10.1016/j.thromres.2009.01.015
Borges M. H., Figueiredo S. G., Leprevost F. V., De Lima M. E., Cordeiro M., do N., et al. (2016). Venomous extract protein profile of Brazilian tarantula Grammostola iheringi: searching for potential biotechnological applications. J. Proteom 136, 35–47. doi: 10.1016/j.jprot.2016.01.013
Bowler R. P., Nicks M., Olsen D. A., Thøgersen I. B., Valnickova Z., Højrup P., et al. (2002). Furin proteolytically processes the heparin-binding region of extracellular superoxide dismutase. J. Biol. Chem. 277, 16505–16511. doi: 10.1074/jbc.M105409200
Bown D. P., Wilkinson H. S., Gatehouse J. A. (1998). Midgut carboxypeptidase from Helicoverpa armigera (Lepidoptera: Noctuidae) larvae: enzyme characterisation, cDNA cloning and expression. Insect Biochem. Mol. Biol. 28, 739–749. doi: 10.1016/S0965-1748(98)00067-8
Brady L., Brzozowski A. M., Derewenda Z. S., Dodson E., Dodson G., Tolley S., et al. (1990). A serine protease triad forms the catalytic centre of a triacylglycerol lipase. Nature 343, 767–770. doi: 10.1038/343767a0
BRENDA Enzyme Database (2024). Available online at: https://www.brenda-enzymes.org/enzyme.php?ecno=3.4.22.15 (Accessed April 26, 2024).
Brigelius-Flohé R., Maiorino M. (2013). Glutathione peroxidases. Biochim. Biophys. Acta Gen. Subj. 1830, 3289–3303. doi: 10.1016/j.bbagen.2012.11.020
Brown M. A., Stenberg L. M., Stenflo J. (2013). “Coagulation factor xa,” in Handbook of Proteolytic Enzymes (Amsterdam, Netherlands: Elsevier), 2908–2915. doi: 10.1016/B978-0-12-382219-2.00642-6
Brufatto N., Nesheim M. E. (2003). Analysis of the kinetics of prothrombin activation and evidence that two equilibrating forms of prothrombinase are involved in the process. J. Biol. Chem. 278, 6755–6764. doi: 10.1074/jbc.M206413200
Buhren B. A., Schrumpf H., Hoff N.-P., Bölke E., Hilton S., Gerber P. A. (2016). Hyaluronidase: from clinical applications to molecular and cellular mechanisms. Eur. J. Med. Res. 21, 5. doi: 10.1186/s40001-016-0201-5
Cai L., Yang F., Wang Y., Yang J., Zhu Y., Ma X., et al. (2022). A combined protein toxin screening based on the transcriptome and proteome of Solenopsis invicta. Proteome Sc 20, 15. doi: 10.1186/s12953-022-00197-z
Cajado-Carvalho D., Kuniyoshi A., Duzzi B., Iwai L., Oliveira Ú., Junqueira De Azevedo I., et al. (2016). Insights into the hypertensive effects of Tityus serrulatus scorpion venom: purification of an angiotensin-converting enzyme-like peptidase. Toxins 8, 348. doi: 10.3390/toxins8120348
Câmara G. A., Nishiyama-Jr M. Y., Kitano E. S., Oliveira U. C., da Silva P. I., Junqueira-de-Azevedo I. L., et al. (2020). A multiomics approach unravels new toxins with possible in silico antimicrobial, antiviral, and antitumoral activities in the venom of Acanthoscurria rondoniae. Front. Pharmacol. 11. doi: 10.3389/fphar.2020.01075
Cardoso F. C., Pineda S. S., Herzig V., Sunagar K., Shaikh N. Y., Jin A.-H., et al. (2022). The deadly toxin arsenal of the tree-dwelling Australian funnel-web spiders. Int. J. Mol. Sci. 23, 13077. doi: 10.3390/ijms232113077
Cardoso F. C., Walker A. A., King G. F., Gomez M. V. (2023). Holistic profiling of the venom from the Brazilian wandering spider Phoneutria nigriventer by combining high-throughput ion channel screens with venomics. Front. Mol. Biosci. 10. doi: 10.3389/fmolb.2023.1069764
Carrillo L., Martinez M., Ramessar K., Cambra I., Castañera P., Ortego F., et al. (2011). Expression of a barley cystatin gene in maize enhances resistance against phytophagous mites by altering their cysteine-proteases. Plant Cell Rep. 30, 101–112. doi: 10.1007/s00299-010-0948-z
Casas-Godoy L., Duquesne S., Bordes F., Sandoval G., Marty A. (2012). “Lipases: an overview,” in Lipases and Phospholipases. Ed. Sandoval G. (Humana Press, Totowa, NJ), 3–30. doi: 10.1007/978-1-61779-600-5_1
Casewell N. R., Harrison R. A., Wüster W., Wagstaff S. C. (2009). Comparative venom gland transcriptome surveys of the saw-scaled vipers (Viperidae: Echis) reveal substantial intra-family gene diversity and novel venom transcripts. BMC Genom 10, 564. doi: 10.1186/1471-2164-10-564
Catalán A., Cortés W., Muñoz C., Araya J. E. (2014). Tryptophan and aspartic acid residues present in the glycerophosphoryl diester phosphodiesterase (GDPD) domain of the Loxosceles laeta phospholipase D are essential for substrate recognition. Toxicon 81, 43–47. doi: 10.1016/j.toxicon.2014.01.011
Catalán A., Cortes W., Sagua H., González J., Araya J. E. (2011). Two new phospholipase D isoforms of Loxosceles laeta: cloning, heterologous expression, functional characterization, and potential biotechnological application. J. Biochem. Mol. Toxicol. 25, 393–403. doi: 10.1002/jbt.20399
Chaim O. M., Sade Y. B., da Silveira R. B., Toma L., Kalapothakis E., Chávez-Olórtegui C., et al. (2006). Brown spider dermonecrotic toxin directly induces nephrotoxicity. Toxicol. Appl. Pharmacol. 211, 64–77. doi: 10.1016/j.taap.2005.05.015
Chakraborty S., Bera A. K., Chaudhuri A., Sen S. (2017). “Metalloproteases and human diseases: the astacin family,” in Proteases in Human Diseases. Eds. Chakraborti S., Chakraborti T., Dhalla N. S. (Springer Singapore, Singapore). doi: 10.1007/978-981-10-3162-5_11
Chaves-Moreira D., Matsubara F. H., Schemczssen-Graeff Z., De Bona E., Heidemann V. R., Guerra-Duarte C., et al. (2019). Brown spider (Loxosceles) venom toxins as potential biotools for the development of novel therapeutics. Toxins 11, 355. doi: 10.3390/toxins11060355
Cheng W., Ka Y.-W., Chang C.-C. (2016). Dopamine β-hydroxylase participate in the immunoendocrine responses of hypothermal stressed white shrimp, Litopenaeus vannamei. Fish Shellfish Immunol. 59, 166–178. doi: 10.1016/j.fsi.2016.10.036
Chettri D., Boro M., Sarkar L., Verma A. K. (2021). Lectins: biological significance to biotechnological application. Carbohydr Res. 506, 108367. doi: 10.1016/j.carres.2021.108367
Coant N., Sakamoto W., Mao C., Hannun Y. A. (2017). Ceramidases, roles in sphingolipid metabolism and in health and disease. Adv. Biol. Regul. 63, 122–131. doi: 10.1016/j.jbior.2016.10.002
Coates D. (2003). The angiotensin converting enzyme (ACE). Int. J. Biochem. Cell Biol. 35, 769–773. doi: 10.1016/S1357-2725(02)00309-6
Coates D., Isaac R. E., Cotton J., Siviter R., Williams T. A., Shirras A., et al. (2000). Functional conservation of the active sites of human and Drosophila angiotensin I-converting enzyme. Biochem 39, 8963–8969. doi: 10.1021/bi000593q
Colinet D., Cazes D., Belghazi M., Gatti J.-L., Poirié M. (2011). Extracellular superoxide dismutase in insects: characterization, function, and interspecific variation in parasitoid wasp venom. J. Biol. Chem. 286, 40110–40121. doi: 10.1074/jbc.M111.288845
Colovic M. B., Krstic D. Z., Lazarevic-Pasti T. D., Bondzic A. M., Vasic V. M. (2013). Acetylcholinesterase inhibitors: pharmacology and toxicology. Curr. Neuropharmacol 11, 315–335. doi: 10.2174/1570159X11311030006
Cordes M. H. J., Binford G. J. (2018). Evolutionary dynamics of origin and loss in the deep history of phospholipase D toxin genes. BMC Evol. Biol. 18, 194. doi: 10.1186/s12862-018-1302-2
Da Lage J.-L. (2018). The amylases of insects. Int. J. Insect Sci. 10, 1179543318804783. doi: 10.1177/1179543318804783
Dantas A. E., Carmo A. O., Horta C. C. R., Leal H. G., Oliveira-Mendes B. B. R., Martins A. P. V., et al. (2016). Description of loxtox protein family and identification of a new group of phospholipases D from Loxosceles similis venom gland. Toxicon 120, 97–106. doi: 10.1016/j.toxicon.2016.08.002
da Silveira R. B., Wille A. C. M., Chaim O. M., Appel M. H., Silva D. T., Franco C. R. C., et al. (2007). Identification, cloning, expression and functional characterization of an astacin-like metalloprotease toxin from Loxosceles intermedia (Brown spider) venom. Biochem. J. 406, 355–363. doi: 10.1042/BJ20070363
Davies R. C., Auld D. S., Vallee B. L. (1968). The effect of modifiers on the hydrolysis of esters and peptides by carboxypeptidase A. Biochemand Biophys. Res. Commun. 31, 628–633. doi: 10.1016/0006-291X(68)90525-1
De Barros C. M., De Abreu Mello A., Allodi S. (2012). Norepinephrine depresses the nitric oxide production in the ascidian hemocytes. J. Invertebr Pathol. 111, 182–185. doi: 10.1016/j.jip.2012.07.002
de Giuseppe P. O., Ullah A., Silva D. T., Gremski L. H., Wille A. C. M., Chaves Moreira D., et al. (2011). Structure of a novel class II phospholipase D: catalytic cleft is modified by a disulphide bridge. Biochemand Biophys. Res. Commun. 409, 622–627. doi: 10.1016/j.bbrc.2011.05.053
Delgado-Prudencio G., Cid-Uribe J. I., Morales J. A., Possani L. D., Ortiz E., Romero-Gutiérrez T. (2022). The enzymatic core of scorpion venoms. Toxins 14, 248. doi: 10.3390/toxins14040248
Dennis E. A., Cao J., Hsu Y.-H., Magrioti V., Kokotos G. (2011). Phospholipase A2 enzymes: physical structure, biological function, disease implication, chemical inhibition, and therapeutic intervention. Chem. Rev. 111, 6130–6185. doi: 10.1021/cr200085w
Dennis E. A., Rhee S. G., Billah M. M., Hannun Y. A. (1991). Role of phospholipases in generating lipid second messengers in signal transduction. FASEB J. 5, 2068–2077. doi: 10.1096/fasebj.5.7.1901288
De Oliveira F. K., Santos L. O., Buffon J. G. (2021). Mechanism of action, sources, and application of peroxidases. Food Res. Int. 143, 110266. doi: 10.1016/j.foodres.2021.110266
de Roodt A. R., Lanari L. C., Laskowicz R. D., Costa de Oliveira V., Irazu L. E., González A., et al. (2017). Toxicity of the venom of Latrodectus (Araneae: Theridiidae) spiders from different regions of Argentina and neutralization by therapeutic antivenoms. Toxicon 130, 63–72. doi: 10.1016/j.toxicon.2017.02.029
Devaraja S., Girish K. S., Devaraj V. R., Kemparaju K. (2010). Factor Xa-like and fibrin(ogen)olytic activities of a serine protease from Hippasa agelenoides spider venom gland extract. J. Thromb. Thrombolysis 29, 119–126. doi: 10.1007/s11239-009-0341-3
Devaraja S., Girish K. S., Gowtham Y. N. J., Kemparaju K. (2011). The Hag-protease-II is a fibrin(ogen)ase from Hippasa agelenoides spider venom gland extract: purification, characterization and its role in hemostasis. Toxicon 57, 248–258. doi: 10.1016/j.toxicon.2010.11.018
Dhananjaya B. L., D’Souza C. J. M. (2010). The pharmacological role of nucleotidases in snake venoms. Cell Biochem. Funct. 28, 171–177. doi: 10.1002/cbf.1637
Diniz M. R. V., Paiva A. L. B., Guerra-Duarte C., Nishiyama M. Y. Jr, Mudadu M. A., Oliveira U., et al. (2018). An overview of Phoneutria nigriventer spider venom using combined transcriptomic and proteomic approaches. PloS One 13, e0200628. doi: 10.1371/journal.pone.0200628
Dodgson S. J., Tashian R. E., Gros G., Carter N. D. (Eds.) (1991). The Carbonic anhydrases: cellular physiology and molecular genetics (Boston, MA: Springer US). doi: 10.1007/978-1-4899-0750-9
Do Nascimento S. M., De Oliveira U. C., Nishiyama-Jr M. Y., Tashima A. K., Silva Junior P. I. D. (2022). Presence of a neprilysin on Avicularia juruensis (Mygalomorphae: Theraphosidae) venom. Toxin Rev. 41, 370–379. doi: 10.1080/15569543.2021.1878226
Dong-hui Y. (2012). The structure and function of cathepsin L. Chin. J. Biochem. Mol. Biol. 28, 1093–1099.
Dos Santos L., Dias N., Roberto J., Pinto A., Palma M. (2009). Brown recluse spider venom: proteomic analysis and proposal of a putative mechanism of action. Protein Pept. Lett. 16, 933–943. doi: 10.2174/092986609788923383
Dresler J., Herzig V., Vilcinskas A., Lüddecke T. (2024). Enlighting the toxinological dark matter of spider venom enzymes. Biorxiv. doi: 10.1101/2024.02.27.582330
Duan Z., Cao R., Jiang L., Liang S. (2013). A combined de novo protein sequencing and cDNA library approach to the venomic analysis of Chinese spider Araneus ventricosus. J. Proteom 78, 416–427. doi: 10.1016/j.jprot.2012.10.011
Duan Z., Yan X., Cao R., Liu Z., Wang X., Liang S. (2008). Proteomic analysis of Latrodectus tredecimguttatus venom for uncovering potential latrodectism-related proteins. J. Biochem. Mol. Toxicol. 22, 328–336. doi: 10.1002/jbt.20244
Duan Z. G., Yan X. J., He X. Z., Zhou H., Chen P., Cao R., et al. (2006). Extraction and protein component analysis of venom from the dissected venom glands of Latrodectus tredecimguttatus. Comp. Biochem. Physiol. B Biochem. Mol. Biol. 145, 350–357. doi: 10.1016/j.cbpb.2006.08.006
Dunbar J. P., Fort A., Redureau D., Sulpice R., Dugon M. M., Quinton L. (2020). Venomics approach reveals a high proportion of Lactrodectus-Like toxins in the venom of the noble false widow spider Steatoda nobilis. Toxins 12, 402. doi: 10.3390/toxins12060402
Dvir H., Silman I., Harel M., Rosenberry T. L., Sussman J. L. (2010). Acetylcholinesterase: from 3D structure to function. Chem. Biol. Interact. 187, 10–22. doi: 10.1016/j.cbi.2010.01.042
E S., Potumarthi R., A N., Mangamoori L. N. (2015). Purification and characterisation of intracellular alpha-galactosidases from Acinetobacter sp. 3 Biotech. 5, 925–932. doi: 10.1007/s13205-015-0290-9
Eble J. (2019). Structurally robust and functionally highly versatile—C-type lectin (-related) proteins in snake venoms. Toxins 11, 136. doi: 10.3390/toxins11030136
Estrada-Gómez S., Vargas-Muñoz L. J., Segura C., Saldarriaga M., Arenas C. (2021). Analysis of high molecular mass compounds from the spider Pamphobeteus verdolaga venom gland. A Ttanscriptomic and MS ID approach. Toxins 13, 453. doi: 10.3390/toxins13070453
Estrada-Gómez S., Vargas-Muñoz L. J., Saldarriaga-Córdoba M., Cifuentes Y., Perafan C. (2017). Identifying different transcribed proteins in the newly described Theraphosidae Pamphobeteus verdolaga. Toxicon 129, 81–88. doi: 10.1016/j.toxicon.2017.02.004
Ferrer V. P., Mari T. L., Gremski L. H., Silva D. T., Silveira R.B.d., Gremski W., et al. (2013). A novel hyaluronidase from brown spider (Loxosceles intermedia) venom (Dietrich’s hyaluronidase): from cloning to functional characterization. PloS Negl. Trop. Dis. 7, e2206. doi: 10.1371/journal.pntd.0002206
Finkel T., Holbrook N. J. (2000). Oxidants, oxidative stress and the biology of ageing. Nature 408, 239–247. doi: 10.1038/35041687
Fischer M. L., Yepes Vivas S. A., Wielsch N., Kirsch R., Vilcinskas A., Vogel H. (2023). You are what you eat—ecological niche and microhabitat influence venom activity and composition in aquatic bugs. Proc. Roy Soc. B Biol. Sci. 290, 20222064. doi: 10.1098/rspb.2022.2064
Fox J. W., Serrano S. M. T. (2005). Structural considerations of the snake venom metalloproteinases, key members of the M12 reprolysin family of metalloproteinases. Toxicon 45, 969–985. doi: 10.1016/j.toxicon.2005.02.012
Franta Z., Frantová H., Konvičková J., Horn M., Sojka D., Mareš M., et al. (2010). Dynamics of digestive proteolytic system during blood feeding of the hard tick Ixodes ricinus. Parasit Vectors 3, 119. doi: 10.1186/1756-3305-3-119
Fricker L. D. (1988). Carboxypeptidase E. Annu. Rev. Physiol. 50, 309–321. doi: 10.1146/annurev.ph.50.030188.001521
Fricker L. D., Evans C. J., Esch F. S., Herbert E. (1986). Cloning and sequence analysis of cDNA for bovine carboxypeptidase E. Nature 323, 461–464. doi: 10.1038/323461a0
Friedman E. R., Seidel S., Heiser S., Prybys K. (2021). Silently suffering: a pediatric black widow spider envenomation. J. Emerg. Med. 61, e151–e154. doi: 10.1016/j.jemermed.2021.02.035
Frobert Y., Créminon C., Cousin X., Rémy M.-H., Chatel J.-M., Bon S., et al. (1997). Acetylcholinesterases from Elapidae snake venoms: biochemical, immunological and enzymatic characterization. Biochim. Biophys. Acta Protein Struct. Mol. Enzymol. 1339, 253–267. doi: 10.1016/S0167-4838(97)00009-5
Furie B., Furie B. C. (1988). The molecular basis of blood coagulation. Cell 53, 505–518. doi: 10.1016/0092-8674(88)90567-3
Futrell J. M. (1992). Loxoscelism. Am. J. Med. Sci. 304, 261–267. doi: 10.1097/00000441-199210000-00008
Fuzita F. J., Pinkse M. W. H., Patane J. S. L., Juliano M. A., Verhaert P. D. E. M., Lopes A. R. (2015a). Biochemical, transcriptomic and proteomic analyses of digestion in the scorpion Tityus serrulatus: insights into function and evolution of digestion in an ancient arthropod. PloS One 10, e0123841. doi: 10.1371/journal.pone.0123841
Fuzita F. J., Pinkse M. W. H., Patane J. S. L., Verhaert P. D. E. M., Lopes A. R. (2016). High throughput techniques to reveal the molecular physiology and evolution of digestion in spiders. BMC Genom 17, 716. doi: 10.1186/s12864-016-3048-9
Fuzita F. J., Pinkse M. W. H., Verhaert P. D. E. M., Lopes A. R. (2015b). Cysteine cathepsins as digestive enzymes in the spider Nephilengys cruentata. Insect Biochem. Mol. Biol. 60, 47–58. doi: 10.1016/j.ibmb.2015.03.005
Galjart N. J., Gillemans N., Meijer D., d’Azzo A. (1990). Mouse “protective protein”. cDNA cloning, sequence comparison, and expression. J. Biol. Chem. 265, 4678–4684. doi: 10.1016/S0021-9258(19)39616-4
Gallo V. P., Accordi F., Chimenti C., Civinini A., Crivellato E. (2016). “Catecholaminergic system of invertebrates: comparative and evolutionary aspects in comparison with the octopaminergic system,” in International Review of Cell and Molecular Biology (Amsterdam, Netherlands: Elsevier), 363–394. doi: 10.1016/bs.ircmb.2015.12.006
Garman S. C., Garboczi D. N. (2004). The molecular defect leading to fabry disease: structure of human α-Galactosidase. J. Mol. Biol. 337, 319–335. doi: 10.1016/j.jmb.2004.01.035
Genta F. A., Blanes L., Cristofoletti P. T., do Lago C. L., Terra W. R., Ferreira C. (2006). Purification, characterization and molecular cloning of the major chitinase from Tenebrio molitor larval midgut. Insect Biochem. Mol. Biol. 36, 789–800. doi: 10.1016/j.ibmb.2006.07.007
Gibbs G. M., Roelants K., O’Bryan M. K. (2008). The CAP superfamily: cysteine-rich secretory proteins, antigen 5, and pathogenesis-related 1 proteins—roles in reproduction, cancer, and immune defense. Endocr. Rev. 29, 865–897. doi: 10.1210/er.2008-0032
Girish K. S., Kemparaju K. (2007). The magic glue hyaluronan and its eraser hyaluronidase: a biological overview. Life Sci. 80, 1921–1943. doi: 10.1016/j.lfs.2007.02.037
Golubev A. M., Nagem R. A. P., Brandão Neto J. R., Neustroev K. N., Eneyskaya E. V., Kulminskaya A. A., et al. (2004). Crystal structure of α-galactosidase from Trichoderma reesei and its complex with galactose: implications for catalytic mechanism. J. Mol. Biol. 339, 413–422. doi: 10.1016/j.jmb.2004.03.062
Gomis-Rüth F. X., Stöcker W. (2023). Structural and evolutionary insights into astacin metallopeptidases. Front. Mol. Biosci. 9. doi: 10.3389/fmolb.2022.1080836
González J. M., Fisher S. Z. (2014). Carbonic anhydrases in industrial applications. Subcell Biochem. 75, 405–426. doi: 10.1007/978-94-007-7359-2_20
Gremski L. H., Matsubara F. H., Justa H. C., Schemczssen-Graeff Z., Baldissera A. B., de C. P. H., et al. (2021). Brown spider venom toxins: what are the functions of astacins, serine proteases, hyaluronidases, allergens, TCTP, serpins and knottins? J. Venom Anim. Toxins Incl Trop. Dis. 27, e20200188. doi: 10.1590/1678-9199-JVATITD-2020-0188
Gremski L. H., Trevisan-Silva D., Ferrer V. P., Matsubara F. H., Meissner G. O., Wille A. C. M., et al. (2014). Recent advances in the understanding of brown spider venoms: from the biology of spiders to the molecular mechanisms of toxins. Toxicon 83, 91–120. doi: 10.1016/j.toxicon.2014.02.023
Guasch A., Coll M., Avilés F. X., Huber R. (1992). Three-dimensional structure of porcine pancreatic procarboxypeptidase A. J. Mol. Biol. 224, 141–157. doi: 10.1016/0022-2836(92)90581-4
Guce A. I., Clark N. E., Salgado E. N., Ivanen D. R., Kulminskaya A. A., Brumer H., et al. (2010). Catalytic mechanism of human α-galactosidase. J. Biol. Chem. 285, 3625–3632. doi: 10.1074/jbc.M109.060145
Gudger E. W. (1931). More spider hunters accounts of arachnids which attack and devour vertebrates other than fishes. Sci. Monthly 32, 422–433.
Gunčar G., Pungerčič G., Klemenčič I., Turk V., Turk D. (1999). Crystal structure of MHC class II-associated p41 Ii fragment bound to cathepsin L reveals the structural basis for differentiation between cathepsins L and S. EMBO J. 18, 793–803. doi: 10.1093/emboj/18.4.793
Guy J. E., Ståhl U., Lindqvist Y. (2009). Crystal structure of a class XIB phospholipase A2 (PLA2). J. Biol. Chem. 284, 19371–19379. doi: 10.1074/jbc.M109.008466
Hamid R., Khan M. A., Ahmad M., Ahmad M. M., Abdin M. Z., Musarrat J., et al. (2013). Chitinases: an update. J. Pharm. Bioallied Sci. 5, 21. doi: 10.4103/0975-7406.106559
Haney R. A., Matte T., Forsyth F. S., Garb J. E. (2019). Alternative transcription at venom genes and its role as a complementary mechanism for the generation of venom complexity in the common house spider. Front. Ecol. Evol. 7. doi: 10.3389/fevo.2019.00085
Harman G. E., Hayes C. K., Broadway R. M., Di Pietro A., Peterbauer C., Tronsmo A. (1993). Chitinolytic enzymes of Trichoderma harzianum: purification of chitobiosidase and endochitinase. Phytopathology 83, 313–318. doi: 10.1094/Phyto-83-313
Harris J., Scott-Davey T. (2013). Secreted phospholipases A2 of snake venoms: effects on the peripheral neuromuscular system with comments on the role of phospholipases A2 in disorders of the CNS and their uses in industry. Toxins 5, 2533–2571. doi: 10.3390/toxins5122533
Hayashi R., Moore S., Stein W. H. (1973). Serine at the active center of yeast carboxypeptidase. J. Biol. Chem. 248, 8366–8369. doi: 10.1016/S0021-9258(19)43142-6
Hedstrom L. (2002). Serine protease mechanism and specificity. Chem. Rev. 102, 4501–4524. doi: 10.1021/cr000033x
Henrissat B., Bairoch A. (1993). New families in the classification of glycosyl hydrolases based on amino acid sequence similarities. Biochem. J. 293, 781–788. doi: 10.1042/bj2930781
Herzig V., King G. F., Undheim E. A. B. (2019). Can we resolve the taxonomic bias in spider venom research? Toxicon 1, 100005. doi: 10.1016/j.toxcx.2018.100005
Hjalmarsson K., Marklund S. L., Engström A., Edlund T. (1987). Isolation and sequence of complementary DNA encoding human extracellular superoxide dismutase. Proc. Natl. Acad. Sci. U.S.A. 84, 6340–6344. doi: 10.1073/pnas.84.18.6340
Hunsucker S. A., Mitchell B. S., Spychala J. (2005). The 5′-nucleotidases as regulators of nucleotide and drug metabolism. Pharmacol. Therapeut 107, 1–30. doi: 10.1016/j.pharmthera.2005.01.003
Hynes W. L., Walton S. L. (2000). Hyaluronidases of gram-positive bacteria. FEMS Microbiol. Lett. 183, 201–207. doi: 10.1111/j.1574-6968.2000.tb08958.x
Ito M., Okino N., Tani M. (2014). New insight into the structure, reaction mechanism, and biological functions of neutral ceramidase. Biochim. Biophys. Acta Mol. Cell Biol. Lipids 1841, 682–691. doi: 10.1016/j.bbalip.2013.09.008
Janeček Š., Svensson B., MacGregor E. A. (2014). α-Amylase: an enzyme specificity found in various families of glycoside hydrolases. Cell Mol. Life Sci. 71, 1149–1170. doi: 10.1007/s00018-013-1388-z
Jenny R. J., Mann K. G., Lundblad R. L. (2003). A critical review of the methods for cleavage of fusion proteins with thrombin and factor Xa. Protein Expr Purif 31, 1–11. doi: 10.1016/S1046-5928(03)00168-2
Johansen J. T., Breddam K., Ottesen M. (1976). Isolation of carboxypeptidase Y by affinity chromatography. Carlsberg Res. Commun. 41, 1–14. doi: 10.1007/BF02908689
Kalapothakis E., Araujo S. C., de Castro C. S., Mendes T. M., Gomez M. V., Mangili O. C., et al. (2002). Molecular cloning, expression and immunological properties of LiD1, a protein from the dermonecrotic family of Loxosceles intermedia spider venom. Toxicon 40, 1691–1699. doi: 10.1016/S0041-0101(02)00201-5
Kalapothakis E., Chatzaki M., Gonçalves-Dornelas H., de Castro C. S., Silvestre F. G., Laborne F. V., et al. (2007). The Loxtox protein family in Loxosceles intermedia (Mello-Leitão) venom. Toxicon 50, 938–946. doi: 10.1016/j.toxicon.2007.07.001
Kamata K., Kawamoto H., Honma T., Iwama T., Kim S.-H. (1998). Structural basis for chemical inhibition of human blood coagulation factor Xa. Proc. Natl. Acad. Sci. U.S.A. 95, 6630–6635. doi: 10.1073/pnas.95.12.6630
Kasprzewska A. (2003). Plant chitinases - regulation and function. Cell Mol. Biol. Lett. 8, 809–824.
Katrolia P., Rajashekhara E., Yan Q., Jiang Z. (2014). Biotechnological potential of microbial α-galactosidases. Crit. Rev. Biotechnol. 34, 307–317. doi: 10.3109/07388551.2013.794124
Keele B. B., McCord J. M., Fridovich I. (1971). Further characterization of bovine superoxide dismutase and Its isolation from bovine heart. J. Biol. Chem. 246, 2875–2880. doi: 10.1016/S0021-9258(18)62263-X
Khamtorn P., Peigneur S., Amorim F. G., Quinton L., Tytgat J., Daduang S. (2022). De novo transcriptome analysis of the venom of Latrodectus geometricus with the discovery of an insect-selective Na channel modulator. Molecules 27, 47. doi: 10.3390/molecules27010047
Kim W., Bae S., Park K., Lee S., Choi Y., Han S., et al. (2011). Biochemical characterization of digestive enzymes in the black soldier fly, Hermetia illucens (Diptera: Stratiomyidae). J. Asia Pac Entomol 14, 11–14. doi: 10.1016/j.aspen.2010.11.003
Kim C., Zabetian C. P., Cubells J. F., Cho S., Biaggioni I., Cohen B. M., et al. (2002). Mutations in the dopamine β-hydroxylase gene are associated with human norepinephrine deficiency. Am. J. Med. Genet. 108, 140–147. doi: 10.1002/ajmg.10196
King G. F., Hardy M. C. (2013). Spider-venom peptides: structure, pharmacology, and potential for control of insect pests. Annu. Rev. Entomol 58, 475–496. doi: 10.1146/annurev-ento-120811-153650
Kini M. R. (2003). Excitement ahead: structure, function and mechanism of snake venom phospholipase A2 enzymes. Toxicon 42, 827–840. doi: 10.1016/j.toxicon.2003.11.002
Kirk O., Borchert T. V., Fuglsang C. C. (2002). Industrial enzyme applications. Curr. Opin. Biotechnol. 13, 345–351. doi: 10.1016/S0958-1669(02)00328-2
Klinman J. P. (2006). The copper-enzyme family of dopamine β-monooxygenase and peptidylglycine α-hydroxylating monooxygenase: resolving the chemical pathway for substrate hydroxylation. J. Biol. Chem. 281, 3013–3016. doi: 10.1074/jbc.R500011200
Kobayashi K., Kurosawa Y., Fujita K., Nagatsu T. (1989). Human dopamine β-hydroxylase gene: two mRNA types having different 3’terminal regions are produced through alternative polyadenylation. Nucleic Acids Res. 17, 1089–1102. doi: 10.1093/nar/17.3.1089
Koga D., Sasaki Y., Uchiumi Y., Hirai N., Arakane Y., Nagamatsu Y. (1997). Purification and characterization of Bombyx mori chitinases. Insect Biochem. Mol. Biol. 27, 757–767. doi: 10.1016/S0965-1748(97)00058-1
Kondoh G., Tojo H., Nakatani Y., Komazawa N., Murata C., Yamagata K., et al. (2005). Angiotensin-converting enzyme is a GPI-anchored protein releasing factor crucial for fertilization. Nat. Med. 11, 160–166. doi: 10.1038/nm1179
Korolkova Y., Mikov A., Lobas A., Solovyeva E., Surin A., Andreev Y., et al. (2023). Venom-gland transcriptomics and venom proteomics of the Tibellus oblongus spider. Sci. Data 10, 820. doi: 10.1038/s41597-023-02703-0
Kote N., Manjula A. C., Vishwanatha T., Patil A. G. G. (2020). High-yield production and biochemical characterization of α-galactosidase produced from locally isolated Penicillium sp. Bull. Natl. Res. Cent 44, 168. doi: 10.1186/s42269-020-00420-x
Koua D., Mary R., Ebou A., BarraChina C., El Koulali K., Cazals G., et al. (2020). Proteotranscriptomic insights into the venom composition of the wolf spider Lycosa tarantula. Toxins 12, 501. doi: 10.3390/toxins12080501
Kozlov S. A., Grishin E. V. (2007). The universal algorithm of maturation for secretory and excretory protein precursors. Toxicon 49, 721–726. doi: 10.1016/j.toxicon.2006.11.007
Kuhn-Nentwig L., Langenegger N., Heller M., Koua D., Nentwig W. (2019). The dual prey-inactivation strategy of spiders—in-depth venomic analysis of Cupiennius salei. Toxins 11, 167. doi: 10.3390/toxins11030167
Kuhn-Nentwig L., Stöcklin R., Nentwig W. (2011). “Venom composition and strategies in spiders: is everything possible?,” in Advances in Insect Physiology. Ed. Casas J. (Amsterdam, Netherlands), 1–86. doi: 10.1016/B978-0-12-387668-3.00001-5
Kulathila R., Consalvo A. P., Fitzpatrick P. F., Freeman J. C., Snyder L. M., Villafranca J. J., et al. (1994). Bifunctional peptidylglcine α-amidating enzyme requires two copper atoms for maximum activity. Arch. Biochem. Biophys. 311, 191–195. doi: 10.1006/abbi.1994.1225
Kumar S., Chakravarty S. (2018). “Amylases,” in Enzymes in Human and Animal Nutrition. Eds. Nunes C. S., Kumar V. (Amsterdam, Netherlands: Elsevier), 163–180. doi: 10.1016/B978-0-12-805419-2.00008-3
Lajoie D. M., Roberts S. A., Zobel-Thropp P. A., Delahaye J. L., Bandarian V., Binford G. J., et al. (2015). Variable substrate preference among phospholipase D toxins from sicariid spiders. J. Biol. Chem. 290, 10994–11007. doi: 10.1074/jbc.M115.636951
Lajoie D. M., Zobel-Thropp P. A., Kumirov V. K., Bandarian V., Binford G. J., Cordes M. H. J. (2013). Phospholipase D toxins of brown spider venom convert lysophosphatidylcholine and sphingomyelin to cyclic phosphates. PloS One 8, e72372. doi: 10.1371/journal.pone.0072372
Landucci E. C. T., Condino-Neto A., Perez A. C., Hyslop S., Corrado A. P., Novello J. C., et al. (1994). Crotoxin induces aggregation of human washed platelets. Toxicon 32, 217–226. doi: 10.1016/0041-0101(94)90111-2
Langenegger N., Koua D., Schürch S., Heller M., Nentwig W., Kuhn-Nentwig L. (2018). Identification of a precursor processing protease from the spider Cupiennius salei essential for venom neurotoxin maturation. J. Biol. Chem. 293, 2079–2090. doi: 10.1074/jbc.M117.810911
Langenegger N., Nentwig W., Kuhn-Nentwig L. (2019). Spider venom: components, modes of action, and novel strategies in transcriptomic and proteomic analyses. Toxins 11, 611. doi: 10.3390/toxins11100611
Langford K. G., Zhou Y., Russell L. D., Wilcox J. N., Bernstein K. E. (1993). Regulated expression of testis angiotensin-converting enzyme during spermatogenesis in mice. Biol. Reprod. 48, 1210–1218. doi: 10.1095/biolreprod48.6.1210
Leśnierowski G., Yang T. (2021). Lysozyme and its modified forms: a critical appraisal of selected properties and potential. Trends Food Sci. Technol. 107, 333–342. doi: 10.1016/j.tifs.2020.11.004
Lee S., Lynch K. R. (2005). Brown recluse spider (Loxosceles reclusa) venom phospholipase D (PLD) generates lysophosphatidic acid (LPA). Biochem. J. 391, 317–323. doi: 10.1042/BJ20050043
Leeb-Lundberg L. M. F., Marceau F., Müller-Esterl W., Pettibone D. J., Zuraw B. L. (2005). Classification of the kinin receptor family: from molecular mechanisms to pathophysiological consequences. Pharmacol. Rev. 57, 27–77. doi: 10.1124/pr.57.1.2
Lehfeldt C., Shirley A. M., Meyer K., Ruegger M. O., Cusumano J. C., Viitanen P. V., et al. (2000). Cloning of the SNG1 gene of arabidopsis reveals a role for a serine carboxypeptidase-like protein as an acyltransferase in secondary metabolism. Plant Cell 12, 1295–1306. doi: 10.1105/tpc.12.8.1295
Lewis E. J., Allison S., Fader D., Claflin V., Baizer L. (1990). Bovine dopamine β-hydroxylase cDNA. Complete coding sequence and expression in mammalian cells with vaccinia virus vector. J. Biol. Chem. 265, 1021–1028. doi: 10.1016/S0021-9258(19)40153-1
Li Z., Niu D., Peng M., Xiong Y., Ji J., Dong Z., et al. (2020b). Dopamine β-hydroxylase and its role in regulating the growth and larval metamorphosis in Sinonovacula constricta. Gene 737, 144418. doi: 10.1016/j.gene.2020.144418
Li P., Zhang Z., Liao Q., Meng E., Mwangi J., Lai R., et al. (2020a). LCTX-F2, a novel potentiator of coagulation factors from the spider venom of Lycosa singoriensis. Front. Pharmacol. 11. doi: 10.3389/fphar.2020.00896
Liao Z., Cao J., Li S., Yan X., Hu W., He Q., et al. (2007). Proteomic and peptidomic analysis of the venom from chinese tarantula Chilobrachys jingzhao. Proteomics 7, 1892–1907. doi: 10.1002/pmic.200600785
Liberato T., Troncone L. R. P., Yamashiro E. T., Serrano S. M. T., Zelanis A. (2016). High-resolution proteomic profiling of spider venom: expanding the toxin diversity of Phoneutria nigriventer venom. Amino Acids 48, 901–906. doi: 10.1007/s00726-015-2151-6
Liu G., Zhou Y., Liu D., Wang Q., Ruan Z., He Q., et al. (2015). Global transcriptome analysis of the tentacle of the jellyfish Cyanea capillata using deep sequencing and expressed sequence tags: insight into the toxin- and degenerative disease-related transcripts. PloS One 10, e0142680. doi: 10.1371/journal.pone.0142680
Lorenz P., Eck J. (2005). Metagenomics and industrial applications. Nat. Rev. Microbiol. 3, 510–516. doi: 10.1038/nrmicro1161
Lüddecke T., Herzig V., von Reumont B. M., Vilcinskas A. (2022). The biology and evolution of spider venoms. Biol. Rev. 97, 163–178. doi: 10.1111/brv.12793
Lüddecke T., Vilcinskas A., Lemke S. (2019). Phylogeny-guided selection of priority groups for venom bioprospecting: harvesting toxin sequences in tarantulas as a case study. Toxins 11, 488. doi: 10.3390/toxins11090488
Lüddecke T., von Reumont B. M., Förster F., Billion A., Timm T., Lochnit G., et al. (2020). An economic dilemma between molecular weapon systems may explain an arachno-atypical venom in wasp spiders (Argiope bruennichi). Biomolecules 10, 978. doi: 10.3390/biom10070978
Macrander J., Broe M., Daly M. (2016). Tissue-specific venom composition and differential gene expression in sea anemones. Genome Biol. Evol. 8, 2358–2375. doi: 10.1093/gbe/evw155
Mann K., Nesheim M., Church W., Haley P., Krishnaswamy S. (1990). Surface-dependent reactions of the vitamin K-dependent enzyme complexes. Blood 76, 1–16. doi: 10.1182/blood.V76.1.1.1
Manning J. C., Romero A., Habermann F. A., García Caballero G., Kaltner H., Gabius H.-J. (2017). Lectins: a primer for histochemists and cell biologists. Histochem Cell Biol. 147, 199–222. doi: 10.1007/s00418-016-1524-6
Marino M. D., Bourdélat-Parks B. N., Cameron Liles L., Weinshenker D. (2005). Genetic reduction of noradrenergic function alters social memory and reduces aggression in mice. Behav. Brain Res. 161, 197–203. doi: 10.1016/j.bbr.2005.02.005
Masood R., Ullah K., Ali H., Ali I., Betzel C., Ullah A. (2018). Spider’s venom phospholipases D: a structural review. Int. J. Biol. Macromol 107, 1054–1065. doi: 10.1016/j.ijbiomac.2017.09.081
Matoba Y., Katsube Y., Sugiyama M. (2002). The crystal structure of prokaryotic phospholipase A2. J. Biol. Chem. 277, 20059–20069. doi: 10.1074/jbc.M200263200
McIntyre N. R., Lowe E. W., Belof J. L., Ivkovic M., Shafer J., Space B., et al. (2010). Evidence for substrate pre-organization in the peptidylglycine α-amidating monooxygenase (PAM) reaction describing the contribution of ground state structure to hydrogen tunneling. J. Am. Chem. Soc. 132, 16393–16402. doi: 10.1021/ja1019194
Mel’nikova U. Y., Karpunina L. V., Ostakhina N. V., Ignatov V. V. (2000). Enzyme activity of lectins from the nitrogen-fixing soil bacterium Bacillus polymyxa. Curr. Microbiol. 41, 246–249. doi: 10.1007/s002840010128
Menniti F. S., Knoth J., Diliberto E. J. (1986). Role of ascorbic acid in dopamine β-hydroxylation. The endogenous enzyme cofactor and putative electron donor for cofactor regeneration. J. Biol. Chem. 261, 16901–16908. doi: 10.1016/S0021-9258(19)75974-2
Mesbah N. M. (2022). Industrial biotechnology based on enzymes from extreme environments. Front. bioeng Biotechnol. 10. doi: 10.3389/fbioe.2022.870083
Michálek O., Walker A. A., Šedo O., Zdráhal Z., King G. F., Pekár S. (2022). Composition and toxicity of venom produced by araneophagous white-tailed spiders (Lamponidae: Lampona sp.). Sci. Rep. 12, 21597. doi: 10.1038/s41598-022-24694-5
Mikola L. (1986). Acid carboxypeptidases in grains and leaves of wheat, Triticum aestivum L. Plant Physiol. 81, 823–829. doi: 10.1104/pp.81.3.823
Mommsen T. P. (1980). Chitinase and β-N-acetylglucosaminidase from the digestive fluid of the spider, Cupiennius salei. Biochim. Biophys. Acta 612, 361–372. doi: 10.1016/0005-2744(80)90119-9
Murakami M. T., Fernandes-Pedrosa M. F., Tambourgi D. V., Arni R. K. (2005). Structural basis for metal ion coordination and the catalytic mechanism of sphingomyelinases D. J. Biol. Chem. 280, 13658–13664. doi: 10.1074/jbc.M412437200
Murakami M. T., Freitas Fernandes-Pedrosa M., de Andrade S. A., Gabdoulkhakov A., Betzel C., Tambourgi D. V., et al. (2006). Structural insights into the catalytic mechanism of sphingomyelinases D and evolutionary relationship to glycerophosphodiester phosphodiesterases. Biochemand Biophys. Res. Commun. 342, 323–329. doi: 10.1016/j.bbrc.2006.01.123
Murakami M., Taketomi Y., Sato H., Yamamoto K. (2011). Secreted phospholipase A2 revisited. J. Biochem. 150, 233–255. doi: 10.1093/jb/mvr088
Murthy K. R., Vakil A. E. (1988). Elevation of plasma angiotensin levels in dogs by Indian red scorpion (Buthus tamulus) venom & its reversal by administration of insulin+tolazoline. Indian J. Med. Res. 88, 376–379.
Muzzarelli R. A. A. (1999). “Native, industrial and fossil chitins,” in Chitin and Chitinases. Eds. Jollès P., Muzzarelli R. A. A. (Birkhäuser Basel, Basel), 1–6. doi: 10.1007/978-3-0348-8757-1_1
Nagaraju S. (2016). “Hippasa spider: biology, envenomation, toxin profiles, and biological functions – a review,” in Spider Venoms. Eds. Gopalakrishnakone P., Corzo G. A., Lima M.E.D., Diego-García E. (Springer Netherlands, Dordrecht), 313–331. doi: 10.1007/978-94-007-6389-0_5
Nagaraju S., Girish K. S., Fox J. W., Kemparaju K. (2007). ‘Partitagin’ a hemorrhagic metalloprotease from Hippasa partita spider venom: role in tissue necrosis. Biochimie 89, 1322–1331. doi: 10.1016/j.biochi.2007.04.005
Nalivaeva N., Zhuravin I., Turner A. (2020). Neprilysin expression and functions in development, ageing and disease. Mech. Ageing Dev. 192, 111363. doi: 10.1016/j.mad.2020.111363
Nishiduka E. S., Abreu T. F., Abukawa F. M., Oliveira U. C., Tardivo C. E. O., Nascimento S. M., et al. (2022). Multiomics Profiling of toxins in the venom of the amazonian spider Acanthoscurria juruenicola. J. Proteome Res. 21, 2783–2797. doi: 10.1021/acs.jproteome.2c00593
Nishihira J., Hibiya Y., Sakai M., Nishi S., Kumazaki T., Ohki S., et al. (1995). The C-terminal region, Arg201 Gln209, of glutathione S-transferase P contributes to stability of the active-site conformation. Biochim. Biophys. Acta Protein Struct. Mol. Enzymol. 1252, 233–238. doi: 10.1016/0167-4838(95)00139-L
Nizet V., Varki A., Aebi M. (2015).Microbial Lectins: hemagglutinins, adhesins, and toxins. In: Essentials of Glycobiology (Cold Spring Harbor (NY: Cold Spring Harbor Laboratory Press). Available online at: http://www.ncbi.nlm.nih.gov/books/NBK453032/ (Accessed April 11, 2024).
Nozik-Grayck E., Suliman H. B., Piantadosi C. A. (2005). Extracellular superoxide dismutase. Int. J. Biochem. Cell Biol. 37, 2466–2471. doi: 10.1016/j.biocel.2005.06.012
Nyffeler M., Gibbons J. W. (2022). Spiders feeding on vertebrates is more common and widespread than previously thought, geographically and taxonomically. J. Arachnol 50, 121–134. doi: 10.1636/JoA-S-21-054
Nyffeler M., Knörnschild M. (2013). Bat predation by spiders. PloS One 8, e58120. doi: 10.1371/journal.pone.0058120
O’Brien P. J. (2000). Peroxidases. Chem. Biol. Interact. 129, 113–139. doi: 10.1016/S0009-2797(00)00201-5
Olaoba O. T., Karina Dos Santos P., Selistre-de-Araujo H. S., Ferreira De Souza D. H. (2020). Snake Venom Metalloproteinases (SVMPs): a structure-function update. Toxicon: X 7, 100052. doi: 10.1016/j.toxcx.2020.100052
Olsen J. V., Ong S.-E., Mann M. (2004). Trypsin cleaves exclusively C-terminal to arginine and lysine residues. Mol. Cell Proteom 3, 608–614. doi: 10.1074/mcp.T400003-MCP200
Ossovskaya V. S., Bunnett N. W. (2004). Protease-activated receptors: contribution to physiology and disease. Physiol. Rev. 84, 579–621. doi: 10.1152/physrev.00028.2003
Ovaere P., Lippens S., Vandenabeele P., Declercq W. (2009). The emerging roles of serine protease cascades in the epidermis. Trends Biochem. Sci. 34, 453–463. doi: 10.1016/j.tibs.2009.08.001
Padmanabhan K., Padmanabhan K. P., Tulinsky A., Park C. H., Bode W., Huber R., et al. (1993). Structure of human des(1-45) factor Xa at 2·2 Å resolution. J. Mol. Biol. 232, 947–966. doi: 10.1006/jmbi.1993.1441
Patil R. S., Ghormade V., Deshpande M. V. (2000). Chitinolytic enzymes: an exploration. Enzyme Microb. Technol. 26, 473–483. doi: 10.1016/S0141-0229(00)00134-4
Peiren N., de Graaf D. C., Vanrobaeys F., Danneels E. L., Devreese B., Van Beeumen J., et al. (2008). Proteomic analysis of the honey bee worker venom gland focusing on the mechanisms of protection against tissue damage. Toxicon 52, 72–83. doi: 10.1016/j.toxicon.2008.05.003
Perry J. J. P., Shin D. S., Getzoff E. D., Tainer J. A. (2010). The structural biochemistry of the superoxide dismutases. Biochim. Biophys. Acta Proteins Proteomics 1804, 245–262. doi: 10.1016/j.bbapap.2009.11.004
Pineda S. S., Chin Y. K.-Y., Undheim E. A. B., Senff S., Mobli M., Dauly C., et al. (2020). Structural venomics reveals evolution of a complex venom by duplication and diversification of an ancient peptide-encoding gene. Proc. Natl. Acad. Sci. U.S.A. 117, 11399–11408. doi: 10.1073/pnas.1914536117
Pleiss J., Fischer M., Schmid R. D. (1998). Anatomy of lipase binding sites: the scissile fatty acid binding site. Chem. Phys. Lipids 93, 67–80. doi: 10.1016/S0009-3084(98)00030-9
Ponting C. P. (2001). Domain homologues of dopamine β-hydroxylase and ferric reductase: roles for iron metabolism in neurodegenerative disorders? Hum. Mol. Genet. 10, 1853–1858. doi: 10.1093/hmg/10.17.1853
Puente X. S., Gutiérrez-Fernández A., Ordóñez G. R., Hillier L. W., López-Otín C. (2005). Comparative genomic analysis of human and chimpanzee proteases. Genomics 86, 638–647. doi: 10.1016/j.ygeno.2005.07.009
Rabiet M. J., Blashill A., Furie B., Furie B. C. (1986). Prothrombin fragment 1 X 2 X 3, a major product of prothrombin activation in human plasma. J. Biol. Chem. 261, 13210–13215. doi: 10.1016/S0021-9258(18)69292-0
Rathore A., Gupta R. (2015). Chitinases from bacteria to human: properties, applications, and future perspectives. Enzyme Res. 2015, 1–8. doi: 10.1155/2015/791907
Rawlings N. D., Salvesen G. (Eds.) (2013a). “Aspartic peptidases,” in Handbook of proteolytic enzymes (Elsevier/AP, Amsterdam), Intro–I1464.
Rawlings N. D., Salvesen G. (Eds.) (2013b). “Metallopeptidases,” in Handbook of proteolytic enzymes (Elsevier/AP, Amsterdam), 1464a–12488.
Rawlings N. D., Salvesen G. (Eds.) (2013c). “Serine and threonine peptidases,” in Handbook of proteolytic enzymes (Elsevier/AP, Amsterdam), 2488a–23932.
Reverter D., Maskos K., Tan F., Skidgel R. A., Bode W. (2004). Crystal structure of human carboxypeptidase M, a membrane-bound enzyme that regulates peptide hormone activity. J. Mol. Biol. 338, 257–269. doi: 10.1016/j.jmb.2004.02.058
Reyes A. M., Vazquez D. S., Zeida A., Hugo M., Piñeyro M. D., De Armas M. I., et al. (2016). PrxQ B from Mycobacterium tuberculosis is a monomeric, thioredoxin-dependent and highly efficient fatty acid hydroperoxide reductase. Free Radic. Biol. Med. 101, 249–260. doi: 10.1016/j.freeradbiomed.2016.10.005
Reyes-Olivares C., Guajardo-Santibáñez A., Segura B., Zañartu N., Penna M., Labra A. (2020). Lizard predation by spiders: a review from the neotropical and andean regions. Ecol. Evol. 10, 10953–10964. doi: 10.1002/ece3.6801
Richmond G. S., Smith T. K. (2011). Phospholipases A1. Int. J. Mol. Sci. 12, 588–612. doi: 10.3390/ijms12010588
Ritonja A., Gubenek F. (1985). Ammodytoxin A, a highly lethal phospholipase A2 from Vipera ammodytes ammodytes venom. Biochim. Biophys. Acta Protein Struct. Mol. Enzymol. 828, 306–312. doi: 10.1016/0167-4838(85)90312-7
Saez N. J., Senff S., Jensen J. E., Er S. Y., Herzig V., Rash L. D., et al. (2010). Spider-venom peptides as therapeutics. Toxins 2, 2851–2871. doi: 10.3390/toxins2122851
Safavi-Hemami H., Möller C., Marí F., Purcell A. W. (2013). High molecular weight components of the injected venom of fish-hunting cone snails target the vascular system. J. Proteom 91, 97–105. doi: 10.1016/j.jprot.2013.07.007
Sahai A. S., Manocha M. S. (1993). Chitinases of fungi and plants: their involvement in morphogenesis and host-parasite interaction. FEMS Microbiol. Rev. 11, 317–338. doi: 10.1111/j.1574-6976.1993.tb00004.x
Salzet M., Deloffre L., Breton C., Vieau D., Schoofs L. (2001). The angiotensin system elements in invertebrates. Brain Res. Rev. 36, 35–45. doi: 10.1016/S0165-0173(01)00063-7
Sanggaard K. W., Bechsgaard J. S., Fang X., Duan J., Dyrlund T. F., Gupta V., et al. (2014). Spider genomes provide insight into composition and evolution of venom and silk. Nat. Commun. 5, 3765. doi: 10.1038/ncomms4765
Sannaningaiah D., Subbaiah G. K., Kempaiah K. (2014). Pharmacology of spider venom toxins. Toxin Rev. 33, 206–220. doi: 10.3109/15569543.2014.954134
Santamaría M. E., Hernández-Crespo P., Ortego F., Grbic V., Grbic M., Diaz I., et al. (2012). Cysteine peptidases and their inhibitors in Tetranychus urticae: a comparative genomic approach. BMC Genomics 13, 307. doi: 10.1186/1471-2164-13-307
Santos A. F. S., Silva M.D.C.d., Napoleão T. H., Paiva P. M. G., Correia M. T. S., Coelho L. C. B. B. (2014). Lectins: Function, structure, biological properties andpotential applications. Curr. Top. Pept. Protein 15, 41-62. Available at: https://repositorium.sdum.uminho.pt/handle/1822/43440.
Sarras M. P. (1996). BMP-1 and the astacin family of metalloproteinases: a potential link between the extracellular matrix, growth factors and pattern formation. BioEssays 18, 439–442. doi: 10.1002/bies.950180604
Sartim M. A., Sampaio S. V. (2015). Snake venom galactoside-binding lectins: a structural and functional overview. J. Venom Anim. Toxins Incl Trop. Dis. 21, 35. doi: 10.1186/s40409-015-0038-3
Schetinger M. R. C., Morsch V. M., Bonan C. D., Wyse A. T. S. (2007). NTPDase and 5’ nucleotidase activities in physiological and disease conditions: New perspectives for human health. BioFactors 31, 77–98. doi: 10.1002/biof.5520310205
Selden P. A., Penney D. (2010). Fossil spiders. Biol. Rev. 85, 171–206. doi: 10.1111/j.1469-185X.2009.00099.x
Sen C. K. (2000). “Biological thiols and redox regulation of cellular signal transduction pathways,” in Handbook of Oxidants and Antioxidants in Exercise (Amsterdam, Netherlands: Elsevier), 375–401. doi: 10.1016/B978-044482650-3/50015-8
Senff-Ribeiro A., Henrique da Silva P., Chaim O. M., Gremski L. H., Paludo K. S., Bertoni da Silveira R., et al. (2008). Biotechnological applications of brown spider (Loxosceles genus) venom toxins. Biotechnol. Adv. 26, 210–218. doi: 10.1016/j.bioteChadv.2007.12.003
Serra V., Von Zglinicki T., Lorenz M., Saretzki G. (2003). Extracellular superoxide dismutase is a major antioxidant in human fibroblasts and slows telomere shortening. J. Biol. Chem. 278, 6824–6830. doi: 10.1074/jbc.M207939200
Sharma R., Chisti Y., Banerjee U. C. (2001). Production, purification, characterization, and applications of lipases. Biotechnol. Adv. 19, 627–662. doi: 10.1016/S0734-9750(01)00086-6
Shi X.-X., Zhu M.-F., Wang N., Huang Y.-J., Zhang M.-J., Zhang C., et al. (2021). Neutral ceramidase is required for the reproduction of brown planthopper, Nilaparvata lugens (Stål). Front. Physiol. 12. doi: 10.3389/fphys.2021.629532
Singh R., Kumar M., Mittal A., Mehta P. K. (2016). Microbial enzymes: industrial progress in 21st century. 3 Biotech. 6, 174. doi: 10.1007/s13205-016-0485-8
Singh C., Oikonomou G., Prober D. A. (2015). Norepinephrine is required to promote wakefulness and for hypocretin-induced arousal in zebrafish. eLife 4, e07000. doi: 10.7554/eLife.07000
Skidgel R. A. (1988). Basic carboxypeptidases: regulators of peptide hormone activity. Trends Pharmacol. Sci. 9, 299–304. doi: 10.1016/0165-6147(88)90015-6
Skidgel R. A., Davis R. M., Tan F. (1989). Human carboxypeptidase M. Purification and characterization of a membrane-bound carboxypeptidase that cleaves peptide hormones. J. Biol. Chem. 264, 2236–2241. doi: 10.1016/S0021-9258(18)94167-0
Skidgel R. A., Erdös E. G. (1998). Cellular carboxypeptidases. Immunol. Rev. 161, 129–141. doi: 10.1111/j.1600-065X.1998.tb01577.x
Stams T., Nair S. K., Okuyama T., Waheed A., Sly W. S., Christianson D. W. (1996). Crystal structure of the secretory form of membrane-associated human carbonic anhydrase IV at 2.8-Å resolution. Proc. Natl. Acad. Sci. U.S.A. 93, 13589–13594. doi: 10.1073/pnas.93.24.13589
Su T., Si M., Zhao Y., Liu Y., Yao S., Che C., et al. (2018). A thioredoxin-dependent peroxiredoxin Q from Corynebacterium glutamicum plays an important role in defense against oxidative stress. PloS One 13, e0192674. doi: 10.1371/journal.pone.0192674
Sussman J. L., Harel M., Frolow F., Oefner C., Goldman A., Toker L., et al. (1991). Atomic structure of acetylcholinesterase from Torpedo californica: a prototypic acetylcholine-binding protein. Science 253, 872–879. doi: 10.1126/science.1678899
Sutti R., Tamascia M., Hyslop S., Rocha-e-Silva T. A. (2014). Purification and characterization of a hyaluronidase from venom of the spider Vitalius dubius (Araneae, Theraphosidae). J. Venom Anim. Toxins Incl Trop. Dis. 20, 2. doi: 10.1186/1678-9199-20-2
Svendsen A. (2000). Lipase protein engineering. Biochim. Biophys. Acta Protein Struct. Mol. Enzymol. 1543, 223–238. doi: 10.1016/S0167-4838(00)00239-9
Tosatto S. C. E., Bosello V., Fogolari F., Mauri P., Roveri A., Toppo S., et al. (2008). The catalytic site of glutathione peroxidases. Antioxid Redox Signal 10, 1515–1526. doi: 10.1089/ars.2008.2055
Trevisan-Silva D., Gremski L. H., Chaim O. M., da Silveira R. B., Meissner G. O., Mangili O. C., et al. (2010). Astacin-like metalloproteases are a gene family of toxins present in the venom of different species of the brown spider (genus Loxosceles). Biochimie 92, 21–32. doi: 10.1016/j.biochi.2009.10.003
Trummal K., Samel M., Aaspõllu A., Tõnismägi K., Titma T., Subbi J., et al. (2015). 5′ Nucleotidase from Vipera lebetina venom. Toxicon 93, 155–163. doi: 10.1016/j.toxicon.2014.11.234
Turk V., Stoka V., Vasiljeva O., Renko M., Sun T., Turk B., et al. (2012). Cysteine cathepsins: from structure, function and regulation to new frontiers. Biochim. Biophys. Acta Proteins Proteomics 1824, 68–88. doi: 10.1016/j.bbapap.2011.10.002
Turner A. J., Hooper N. M. (2002). The angiotensin–converting enzyme gene family: genomics and pharmacology. Trends Pharmacol. Sci. 23, 177–183. doi: 10.1016/S0165-6147(00)01994-5
Turner A. J., Isaac R. E., Coates D. (2001). The neprilysin (NEP) family of zinc metalloendopeptidases: genomics and function. Bioessays 23, 261–269. doi: 10.1002/1521-1878(200103)23:3<261::AID-BIES1036>3.0.CO;2-K
Undheim E. A. B., Sunagar K., Herzig V., Kely L., Low D. H. W., Jackson T. N. W., et al. (2013). A proteomics and transcriptomics investigation of the venom from the Barychelid spider Trittame loki (brush-foot trapdoor). Toxins 5, 2488–2503. doi: 10.3390/toxins5122488
Usmanov P. B., Nuritova F. A. (1994). The anticoagulant action of phospholipase A from Eresus Niger spider venom. Toxicon 32, 625–628. doi: 10.1016/0041-0101(94)90210-0
Valladão R., Neto O. B. S., de Oliveira Gonzaga M., Pimenta D. C., Lopes A. R. (2023). Digestive enzymes and sphingomyelinase D in spiders without venom (Uloboridae). Sci. Rep. 13, 2661. doi: 10.1038/s41598-023-29828-x
Vanthournout B., Greve M., Bruun A., Bechsgaard J., Overgaard J., Bilde T. (2016). Benefits of group living include increased feeding efficiency and lower mass loss during desiccation in the social and inbreeding spider Stegodyphus dumicola. Front. Physiol. 7. doi: 10.3389/fphys.2016.00018
Varlamov O., Fricker L. D. (1996). The C-terminal region of carboxypeptidase E involved in mMembrane binding is distinct from the region involved with intracellular routing. J. Biol. Chem. 271, 6077–6083. doi: 10.1074/jbc.271.11.6077
Vassilevski A. A., Kozlov S. A., Grishin E. V. (2009). Molecular diversity of spider venom. Biochem 74, 1505–1534. doi: 10.1134/S0006297909130069
Vendrell J., Querol E., Avilés F. X. (2000). Metallocarboxypeptidases and their protein inhibitors. Biochim. Biophys. Acta Protein Struct. Mol. Enzymol. 1477, 284–298. doi: 10.1016/S0167-4838(99)00280-0
Venegas A., Goldstein J. C., Beauregard K., Oles A., Abdulhayoglu N., Fuhrman J. A. (1996). Expression of recombinant microfilarial chitinase and analysis of domain function. Mol. Biochem. Parasitol. 78, 149–159. doi: 10.1016/S0166-6851(96)02620-5
Villegas V., Vendrell J., Avilés F. X. (1995). The activation pathway of procarboxypeptidase B from porcine pancreas: Participation of the active enzyme in the proteolytic processing. Protein Sci. 4, 1792–1800. doi: 10.1002/pro.5560040914
Walker A. A., Hernández-Vargas M. J., Corzo G., Fry B. G., King G. F. (2018). Giant fish-killing water bug reveals ancient and dynamic venom evolution in Heteroptera. Cell Mol. Life Sci. 75, 3215–3229. doi: 10.1007/s00018-018-2768-1
Walter A., Bechsgaard J., Scavenius C., Dyrlund T. S., Sanggaard K. W., Enghild J. J., et al. (2017). Characterisation of protein families in spider digestive fluids and their role in extra-oral digestion. BMC Genom 18, 600. doi: 10.1186/s12864-017-3987-9
Wang Y., Luo W., Reiser G. (2008). Trypsin and trypsin-like proteases in the brain: proteolysis and cellular functions. Cell Mol. Life Sci. 65, 237–252. doi: 10.1007/s00018-007-7288-3
Weignerová L., Simerská P., Křen V. (2009). α-Galactosidases and their applications in biotransformations. Biocatal Biotransfor 27, 79–89. doi: 10.1080/10242420802583416
Wernicke J. F., Vanker A. D., Howard B. D. (1975). The mechanism of action of β-bungarotoxin. J. Neurochem. 25, 483–496. doi: 10.1111/j.1471-4159.1975.tb04354.x
Winther J. R., Stevens T. H., Kielland-Brandt M. C. (1991). Yeast carboxypeptidase Y requires glycosylation for efficient intracellular transport, but not for vacuolar sorting, in vivo stability, or activity. Eur. J. Biochem. 197, 681–689. doi: 10.1111/j.1432-1033.1991.tb15959.x
World Spider Catalog (2024). World Spider Catalog. Version 25.0. (Natural History Museum Bern). doi: 10.24436/2
Wu Y., Cui Z., Huang Y.-H., de Veer S. J., Aralov A. V., Guo Z., et al. (2022). Towards a generic prototyping approach for therapeutically-relevant peptides and proteins in a cell-free translation system. Nat. Commun. 13, 260. doi: 10.1038/s41467-021-27854-9
Wu T., Jiang Q., Wu D., Hu Y., Chen S., Ding T., et al. (2019). What is new in lysozyme research and its application in food industry? A review. Food Chem. 274, 698–709. doi: 10.1016/j.foodchem.2018.09.017
Xin X., Varlamov O., Day R., Dong W., Bridgett M. M., Leiter E. H., et al. (1997). Cloning and sequence analysis of cDNA encoding rat carboxypeptidase D. DNA Cell Biol. 16, 897–909. doi: 10.1089/dna.1997.16.897
Yadati T., Houben T., Bitorina A., Shiri-Sverdlov R. (2020). The ins and outs of cathepsins: physiological function and role in disease management. Cells 9, 1679. doi: 10.3390/cells9071679
Yamada S., Sugahara K., Özbek S. (2011). Evolution of glycosaminoglycans: comparative biochemical study. Commun. Integr. Biol. 4, 150–158. doi: 10.4161/cib.4.2.14547
Yasothornsrikul S., Greenbaum D., Medzihradszky K. F., Toneff T., Bundey R., Miller R., et al. (2003). Cathepsin L in secretory vesicles functions as a prohormone-processing enzyme for production of the enkephalin peptide neurotransmitter. Proc. Natl. Acad. Sci. U.S.A. 100, 9590–9595. doi: 10.1073/pnas.1531542100
Yin E. T. (1964). Kinetics of human blood coagulation induced by trypsin. Thromb. Haemost. 12, 307–330. doi: 10.1055/s-0038-1655616
Yoon K. A., Kim K., Kim W.-J., Bang W. Y., Ahn N.-H., Bae C.-H., et al. (2020). Characterization of venom components and their phylogenetic properties in some aculeate bumblebees and wasps. Toxins 12, 47. doi: 10.3390/toxins12010047
Yuan C., Jin Q., Tang X., Hu W., Cao R., Yang S., et al. (2007). Proteomic and peptidomic characterization of the venom from the chinese bird spider, Ornithoctonus huwena Wang. J. Proteome Res. 6, 2792–2801. doi: 10.1021/pr0700192
Yuli P. E., Suhartono M. T., Rukayadi Y., Hwang J. K., Pyun Y. R. (2004). Characteristics of thermostable chitinase enzymes from the Indonesian Bacillus sp.13.26. Enzyme Microb. Technol. 35, 147–153. doi: 10.1016/j.enzmictec.2004.03.017
Zhang Y., Chen J., Tang X., Wang F., Jiang L., Xiong X., et al. (2010). Transcriptome analysis of the venom glands of the chinese wolf spider Lycosa singoriensis. Zoology 113, 10–18. doi: 10.1016/j.zool.2009.04.001
Zhang H., Wang Z., Huang J., Cao J., Zhou Y., Zhou J. (2020). A novel thioredoxin-dependent peroxiredoxin (TPx-Q) plays an important role in defense against oxidative stress and is a possible drug target in Babesia microti. Front. Vet. Sci. 7. doi: 10.3389/fvets.2020.00076
Zhang C., Wu S., Xu D. (2013). Catalytic mechanism of angiotensin-converting enzyme and effects of the chloride ion. J. Phys. Chem. B 117, 6635–6645. doi: 10.1021/jp400974n
Zhu B., Jin P., Hou Z., Li J., Wei S., Li S. (2022). Chromosomal-level genome of a sheet-web spider provides insight into the composition and evolution of venom. Mol. Ecol. Resour 22, 2333–2348. doi: 10.1111/1755-0998.13601
Zigrino P., Kamiguti A. S., Eble J., Drescher C., Nischt R., Fox J. W., et al. (2002). The reprolysin jararhagin, a snake venom metalloproteinase, functions as a fibrillar collagen agonist involved in fibroblast cell adhesion and signaling. J. Biol. Chem. 277, 40528–40535. doi: 10.1074/jbc.M202049200
Zimmermann H. (1992). 5′-Nucleotidase: molecular structure and functional aspects. Biochem. J. 285, 345–365. doi: 10.1042/bj2850345
Zobel-Thropp P. A., Bulger E. A., Cordes M. H. J., Binford G. J., Gillespie R. G., Brewer M. S. (2018). Sexually dimorphic venom proteins in long-jawed orb-weaving spiders (Tetragnatha) comprise novel gene families. PeerJ 6, e4691. doi: 10.7717/peerj.4691
Zobel-Thropp P. A., Mullins J., Kristensen C., Kronmiller B. A., David C. L., Breci L. A., et al. (2019). Not so dangerous after all? Venom composition and potency of the pholcid (Daddy Long-Leg) spider Physocyclus mexicanus. Front. Ecol. Evol. 7. doi: 10.3389/fevo.2019.00256
Keywords: Araneae, biocatalysis, biodiscovery, venomics, industrial application, toxinology
Citation: Dresler J, Avella I, Damm M, Dersch L, Krämer J, Vilcinskas A and Lüddecke T (2024) A roadmap to the enzymes from spider venom: biochemical ecology, molecular diversity, and value for the bioeconomy. Front. Arachn. Sci. 3:1445500. doi: 10.3389/frchs.2024.1445500
Received: 07 June 2024; Accepted: 30 July 2024;
Published: 20 August 2024.
Edited by:
Greta J. Binford, Lewis & Clark College, United StatesReviewed by:
Karla De Castro Figueiredo Bordon, University of São Paulo, BrazilAdriana Rios Lopes, Butantan Institute, Brazil
Copyright © 2024 Dresler, Avella, Damm, Dersch, Krämer, Vilcinskas and Lüddecke. This is an open-access article distributed under the terms of the Creative Commons Attribution License (CC BY). The use, distribution or reproduction in other forums is permitted, provided the original author(s) and the copyright owner(s) are credited and that the original publication in this journal is cited, in accordance with accepted academic practice. No use, distribution or reproduction is permitted which does not comply with these terms.
*Correspondence: Josephine Dresler, am9zZXBoaW5lLmRyZXNsZXJAaW1lLmZyYXVuaG9mZXIuZGU=; Tim Lüddecke, dGltLmx1ZWRkZWNrZUBpbWUuZnJhdW5ob2Zlci5kZQ==