- 1Department of Parasitology, Institute of Biomedical Sciences, University of São Paulo, São Paulo, Brazil
- 2Direction of Animal Health, National Center for Animal and Plant Health, Carretera de Tapaste y Autopista Nacional, San José de las Lajas, Mayabeque, Cuba
- 3Anses, INRAE, Ecole Nationale Vétérinaire d’Alfort, UMR BIPAR, Laboratoire de Santé Animale, Maisons‐Alfort, France
- 4Department of Animal Biotechnology, Center for Genetic Engineering and Biotechnology, Havana, Cuba
- 5Institute of Parasitology, Biology Centre, Czech Academy of Sciences, České Budeovice, Czechia
Vector-borne diseases (VBDs) pose significant global health threats. The microbiota of arthropod vectors influences their fitness and pathogen acquisition and/or transmission. Here, we review the intricate interplay among the arthropod immune system, the microbiota, and pathogens that limits or favors infection. We focused on the most important arthropod vectors, namely mosquitos, phlebotomines, tsetse flies, triatomines, and ticks, and expanded our analysis to include the nonvector model Drosophila melanogaster for comparison. The microbiota and immune system of arthropod vectors are targets for the development of promising control strategies, such as paratransgenesis and anti‐microbiota vaccines. Further research should focus on elucidating the underlying mechanisms of vector–pathogen–microbiota interactions and optimizing anti-microbiota strategies. These approaches have the potential to combat VBDs and reduce their global impact.
1 Introduction
Arthropoda is the largest phylum of the animal kingdom, comprising more than 80% of the species described thus far (Thorp, 2009). This enormous diversity and the wide distribution of arthropods in virtually all ecological niches on Earth attest to the efficiency of their adaptations. Arthropods come in contact with microorganisms throughout their life cycle. Some of these microorganisms are commensals or mutualistic and confer biological advantages (for instance, in nutrition, development or sexual reproduction), constituting the arthropod resident microbiota (Douglas, 2014), while others are harmful. Therefore, arthropods must be able to control dangerous microorganisms while maintaining a balanced microbiota community to maintain homeostasis. The members of the resident microbiota can also exert a positive or a negative effect on the establishment of pathogens in the arthropod gut using different mechanisms, including (i) direct interaction; (ii) competition for nutrients; (iii) and modulation (up- or downregulation) of components of the arthropod immune system (Weiss and Aksoy, 2011).
In this review, we summarize the main aspects of the tuned symphony played by the resident microbiota and the molecular factors in the arthropod gut that consequently allow or restrict pathogen establishment in disease vectors and, for comparative purposes, in the nonvector model Drosophila melanogaster (hereafter referred to as Drosophila).
2 Drosophila: the nonvector model
The fruit fly Drosophila (Diptera: Drosophilidae) is widely used as a model for studying the interactions among the arthropod immune system, the microbiota, and pathogens (Lee and Lee, 2014). The fly gut microbiota is mainly located in the anterior gut (Watnick and Jugder, 2020), and its composition varies between wild and laboratory-reared flies. Wild flies are colonized by Actinobacteria, Firmicutes, Bacteroidetes, and alpha-, beta- and gamma-Proteobacteria (Chandler et al., 2011; Adair et al., 2018), while laboratory-reared flies are mainly colonized by Acetobacter and Lactobacillus spp. (Blum et al., 2013; Pais et al., 2018).
In the Drosophila gut, the immune deficiency (IMD) pathway is triggered by bacterial diaminopimelic acid-type peptidoglycan (DAP-PGN), leading to the activation of the nuclear factor-kappa B (NF-κB) Relish and the transcription of antimicrobial peptides (AMPs) (Lemaitre and Hoffmann, 2007; Kleino and Silverman, 2014) (Figure 1A). Relish-dependent AMP expression induced by commensal bacteria is specifically regulated by Caudal, a transcriptional repressor (Ryu et al., 2008) (Figure 1A). The IMD pathway also activates the transcription of two enteroendocrine peptides: diuretic hormone 31 (DH31) and tachykinin (Tk) (Veenstra et al., 2008). DH31 increases gut contractions, mechanically limiting the number of extracellular bacteria (Vanderveken and O'donnell, 2014), while Tk inhibits lipid synthesis in enterocytes, reducing the number of intracellular bacteria (Song et al., 2014) (Figure 1A).
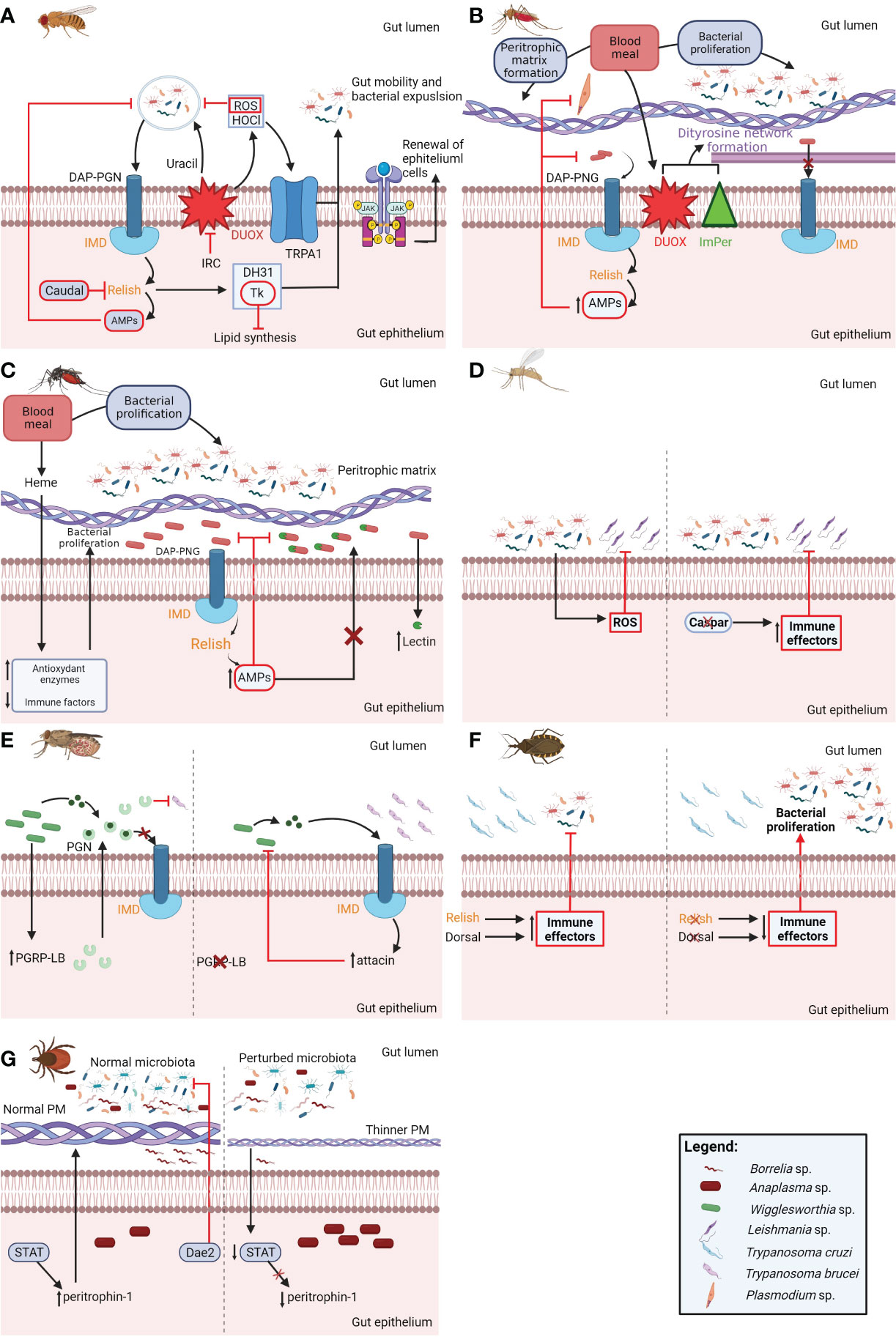
Figure 1 Schematic representation of the principal interactions among pathogens, the microbiota, and the arthropod gut components. In the Drosophila gut, DAP-PGN (diaminopimelic acid type-peptidoglycan) of both pathogenic and nonpathogenic bacteria activates the IMD (immune deficiency) pathway, leading to the activation of Relish and the expression of AMPs (antimicrobial peptides). The production of Relish-dependent AMPs is inhibited by Caudal. The IMD pathway also controls the expression of DH31 (diuretic hormone 31) and Tk (tachykinin). The uracil from pathogenic bacteria activates the production of ROS (reactive oxygen species) and HOCl (hypochlorous acid) by DUOX (dual-oxidase), which is inhibited by IRC (immune-regulated catalase). The activation of the HOCl-sensitive receptor TRPA1 (A) 10b (isoform of the HOCl-sensitive receptor TRPA1) and DH3 causes an increase in gut motility and the expulsion of bacteria from the lumen. Tk reduces intracellular bacterial proliferation by inhibiting lipid synthesis. The JAK/STAT (Janus kinase/signal transducer and activator of transcription) pathway regulates epithelial cell renewal and intestinal stem cell proliferation and controls the bacterial load in the fly gut (A). The acquisition of a blood meal causes an increase in bacterial population size in the Anopheles gut, activating the IMD pathway by the recognition of DAP-PGN. Effectors of the IMD pathway exert a negative effect on Plasmodium. The blood meal also stimulates the production of the PM (peritrophic matrix), trapping both bacteria and Plasmodium; in turn, the parasite produces a chitinase to escape from it. An additional barrier, named the dityrosine network, is produced by IMPer (immunomodulatory peroxidase) in cooperation with DUOX, impairing immune system activation. Plasmodium infection reduces the activity of catalase, perturbing the microbiota and favoring its development (B). In the Aedes gut, the heme from the host blood upregulates the expression of antioxidant genes and downregulates that of immune-related genes, favoring bacterial proliferation. The increase in bacterial load activates the IMD pathway, limiting SINV (Sindbis virus) replication. Microbiota bacteria upregulate the expression of mosquito CTLs (C-type lectins), using them for protection against AMP effects (C). In the sand fly L. longipalpis, Serratia causes an increase in ROS concentration, which negatively impacts Leishmania. Infection by L. mexicana and L. infantum is also reduced in Caspar-silenced sand flies, possibly due to augmented expression of IMD pathway effectors (D). In tsetse flies, RNAi-mediated silencing of PGRB-LB (peptidoglycan recognition protein LB) permits the peptidoglycan (PGN) released by Wigglesworthia to activate the IMD pathway, increasing the levels of the AMP attacin and reducing Wigglesworthia loads. PGRB-LB may also exert anti-T. brucei activity, as parasite loads are higher in silenced flies (E). In the gut of R. prolixus, the silencing of either Relish or Dorsal [NF-κB (nuclear factor-kappa B) of the IMD or Toll pathway, respectively] causes an increase in the load of the symbiotic bacterium R. rhodinii, but does not alter T. cruzi infection (F). In the tick I. scapularis, microbiota alterations downregulate STAT, with a consequent reduction in peritrophin-1 gene expression. A thinner PM reduces infection by B. burgdorferi but increases infection by A. phagocytophilum. The tick epithelium secretes Dae2 (domesticated amidase effector 2), which acts against host skin bacteria, preventing their proliferation (G).
The Drosophila gut also produces reactive oxygen species (ROS) and hypochlorous acid (HOCl) through dual-oxidase (DUOX) activity (Ha et al., 2005a). Unlike PGN-sensing IMD pathway activation, DUOX is triggered by uracil secreted by pathogenic bacteria (Lee et al., 2013). To neutralize DUOX-dependent ROS production, Drosophila encodes the immune-regulated catalase (IRC) (Ha et al., 2005b) (Figure 1A). DUOX-produced HOCl activates the isoform TRPA1 (A) 10b of the HOCl-sensitive receptor TRPA1, leading to increased gut motility and the expulsion of uracil-releasing bacteria (Du et al., 2016). The Janus kinase/signal transducer and activator of transcription (JAK/STAT) immune signaling pathway also plays a role in bacterial control in the Drosophila gut, regulating epithelial cell renewal and intestinal stem cell proliferation (Ohlstein and Spradling, 2006).
In summary, the fruit-fly Drosophila employs a complex network of immune factors, gut motility, and intracellular control of lipid metabolism to maintain gut homeostasis. These findings provide valuable insights into the intricate mechanisms governing insect–microbe interactions and offer potential avenues for understanding and manipulating immune responses in other organisms.
3 Disease-transmitting vectors
The blood-feeding behavior of some ectoparasitic arthropods allows them to acquire and transmit a wide array of pathogens, including viruses, bacteria, protozoans, and nematodes, which can cause life-threatening diseases in humans and other animals (Müller R. et al., 2019). Notably, mosquitoes, phlebotomine sandflies, tsetse flies, triatomine bugs, and ticks stand out among pathogen-carrying arthropods.
According to the World Health Organization (WHO), vector-borne diseases (VBDs) cause more than 700,000 human deaths every year1. These diseases disproportionately impact impoverished regions where favorable conditions for transmission exist (Müller R. et al., 2019). In this alarming scenario, studies on the molecular factors governing the relationships between arthropod vectors, the microbiota, and pathogens are highly relevant for revealing new targets for the development of innovative strategies to control VBDs.
3.1 Mosquitoes: the multidisease vectors
Mosquitoes (Diptera: Culicidae) are prolific vectors of different human pathogens, including arboviruses, protozoans, and nematodes. They acquire their microbiota mainly from the environment; however, bacteria in the female reproductive tract can also be transmitted to progeny. In this case, microorganisms on the egg shell are eaten by larvae during hatching or spread in the aquatic habitat where larvae feed. It has also been shown that the microorganisms present at an egg laying site can exert either an attractant or a repellent effect on gravid female mosquitoes, and they can act as a stimulant or deterrent of oviposition (reviewed by Girard et al., 2021). For example, Pantoea stewartii shows a positive effect on the oviposition of gravid Anopheles gambiae females, possibly mediated by the release of indole and 3-methyl-1-butanol (Lindh et al., 2008). A mixture of bacteria isolated from either bamboo or white-oak leaf infusions also exerted a positive effect on the oviposition of Aedes aegypti. In addition, a blend of synthetic carboxylic acids at the natural ratio found in the bacterial mixture (consisting of 16% nonanoic acid, 83% tetradecanoic acid, and 1% methyl tetradecanoate) was highly stimulatory to oviposition of Ae. aegypti females (Ponnusamy et al., 2008).
The microbiota of mosquitoes consists mostly of gram-negative bacteria in the phyla Proteobacteria, Firmicutes, Bacteroidetes and Actinobacteria (Strand, 2018). After a blood-meal, the microbiota bacterial density substantially increases in the gut of adult females (Romoli and Gendrin, 2018), activating the immune system and impacting pathogen establishment. However, bacteria are subsequently eliminated with the peritrophic matrix (PM), returning to pre-blood feeding levels (Rodgers et al., 2017).
Anopheles mosquitoes are the main vectors of Plasmodium, the causative agent of malaria, one of the most severe VBDs in the world (Phillips et al., 2017). Proteobacteria, Bacteroidetes, Actinobacteria, Firmicutes, and Fusobacteria are the most abundant phyla in the gut microbiota of adult Anopheles gambiae originating from field-collected larvae (Boissiere et al., 2012). Laboratory-reared mosquitoes harbor a different microbiota, dominated by Flavobacteria, which in field mosquitoes corresponds to only 0.38% of the total bacteria (Dong et al., 2009; Boissiere et al., 2012). In addition, while 5% of the total bacteria in field mosquitos are gram-positive bacteria (in the classes Bacilli and Actinobacteria), no gram-positive bacterium has been identified in laboratory-reared mosquitoes. Interestingly, Plasmodium falciparum-positive mosquitoes harbor more Enterobacteriaceae bacteria, suggesting a potential positive effect on parasite establishment (Boissiere et al., 2012).
The increase in bacterial population size after a blood-meal activates the mosquito IMD pathway through the recognition of DAP-PGN, reducing the load of Plasmodium (Meister et al., 2009) (Figure 1B). The elimination of the microbiota by antibiotic treatment increases the numbers of ookinetes and oocysts in the mosquito gut (Dong et al., 2009). Among the IMD effectors identified thus far, thioester-containing protein 1 (TEP-1) has been implicated in Plasmodium control. TEP-1 binds to the ookinete in the mosquito hemocoel, leading to its melanization or lysis (Blandin et al., 2004; Garver et al., 2012). The microbiota contributes to the formation of the PM, protecting mosquitoes from bacterial dissemination into the hemocoel (Rodgers et al., 2017). The PM also traps Plasmodium, which, in turn, produces a chitinase to escape it (Huber et al., 1991) (Figure 1B). Disruption of the P. falciparum chitinase encoding gene impairs its ability to form oocysts in the Anopheles freeborni gut (Tsai et al., 2001). In a microbiota independent process, the acquisition of a blood-meal upregulates the expression of immunomodulatory peroxidase (IMPer) (Kumar et al., 2010). In cooperation with DUOX, IMPer leads to the formation of a dityrosine network between the gut epithelium and the PM (Figure 1B). This network reduces the activation of the mosquito immune system, which is essential for the survival of the microbiota bacteria and Plasmodium (Kumar et al., 2010). Plasmodium perturbs the mosquito microbiota, reducing, for instance, catalase activity and affecting redox metabolism, which favors parasite development (Bahia et al., 2013) (Figure 1B). Specifically, the knockdown of catalase, mediated by RNA interference (RNAi), increases the parasite load and decreases the bacterial load. Together, these data suggest that Plasmodium manipulates vector redox metabolism, diminishing the activity of antioxidant enzymes, which affect the microbiota and favor its own development (Bahia et al., 2013).
Ae. aegypti mosquitoes transmit a range of arboviruses, including dengue virus (DENV), zika virus (ZIKV), chikungunya virus (CHIKV), and yellow fever virus (YFV). Several studies have shown that the larval microbiota influences the vector competence of adult mosquitoes (reviewed by Caragata et al., 2019). For example, colonization of larvae exclusively with Salmonella sp. increases DENV infection of adult mosquitoes in comparison to those colonized with a mixture of different bacteria in the family Enterobacteriaceae (Dickson et al., 2017). The exposure of larvae to pathogenic Bacillus thuringiensis subsp. israelensis also alters the mosquito microbial community (Tetreau et al., 2018), increasing the susceptibility of adult mosquitoes to DENV but not to CHIKV (Moltini-Conclois et al., 2018).
In the gut of adult Ae. aegypti, the heme released upon blood digestion upregulates the expression of antioxidant genes and downregulates that of immune-related genes, resulting in increased bacterial proliferation (Oliveira et al., 2011). The higher bacterial load triggers the activation of the IMD pathway and production of AMPs (Figure 1C). In turn, microbiota bacteria upregulate the expression of C-type lectins (CTLs) in the mosquito gut, which bind to the bacterial cell wall, protecting them from Relish-regulated AMPs (Pang et al., 2016) (Figure 1C). The activation of the IMD pathway by microbiota bacteria limits Sindbis virus (SINV) replication in infected mosquitoes (Barletta et al., 2017) (Figure 1C).
In conclusion, the acquisition of a blood meal results in an increase in the microbiota load, modulating the production of immune factors and structural components in the mosquito gut and shaping vector competence to Plasmodium and certain arboviruses.
3.2 Sand flies: complex relationships among numerous species of vectors and parasites
Sand flies (Diptera: Psychodidae) are vectors of protozoans in the genus Leishmania, causative agents of leishmaniasis (Telleria et al., 2018). Sand flies can also transmit viruses (Depaquit et al., 2010) and bacteria (Herrer and Christensen, 1975). The most relevant sand fly genera for human leishmaniasis are Phlebotomus (found in the Old World) and Lutzomyia (found in the New World). There are approximately 42 species of Phlebotomus and 56 species of Lutzomyia known to transmit human leishmaniasis (Telleria et al., 2018) and approximately 20 species of Leishmania described to cause disease in humans (Maroli et al., 2013).
Due to the vast number of sand fly species and the diverse habitats they may occupy, the composition of the microbiota varies significantly. However, a network analysis of sixteen studies on the Phlebotomus and Lutzomyia microbiotas revealed common bacteria shared among some species (Fraihi et al., 2017). When analyzing bacterial phyla, both Phlebotomus and Lutzomyia were found to be mainly composed of Proteobacteria, followed by Firmicutes and Actinobacteria. In terms of order, Gammaproteobacteria and Bacilli were the most abundant in both sand fly genera. The main family in the Gammaproteobacteria order is Enterobacteriaceae, which is present in both Phlebotomus and Lutzomyia (Fraihi et al., 2017). The microbiota composition of Lutzomyia longipalpis larvae and adults widely varies according to the substrate where eggs are laid (Martins et al., 2021). However, reduced microbiota diversity is observed in L. longipalpis infected by Leishmania infantum, suggesting that the parasite acts as an equalizer agent of the vector microbiota (Kelly et al., 2017). Moreover, treatment with antibiotics results in reduced bacterial and Leishmania spp. loads in both L. longipalpis (Kelly et al., 2017) and Phlebotomus duboscqi (Louradour et al., 2017). On the other hand, prefeeding L. longipalpis with specific commensal bacteria or a mixture of bacteria and yeast make the sand fly less susceptible to Leishmania mexicana infection (Sant'anna et al., 2014). Serratia, but not L. mexicana, increases ROS concentrations (including H2O2) in the L. longipalpis gut (Diaz-Albiter et al., 2012). The oral administration of ROS to sand flies and by RNAi-mediated silencing of catalase reduced the L. mexicana load (Diaz-Albiter et al., 2012) (Figure 1D). The knockdown of Caspar, an inhibitor of the IMD pathway, also reduces infection by L. mexicana and L. infantum (Telleria et al., 2012). However, microbiota bacteria remained unaltered in Caspar-silenced L. longipalpis (Figure 1D). Leishmania-infected sand flies are resistant to infection with Serratia, suggesting that the parasite may also exert a negative effect on bacteria (Sant'anna et al., 2014).
Ten cultivable bacterial genera present in the L. longipalpis midgut were identified, and the effect of some of these genera on the growth of L. infantum chagasi, L. major, L. amazonensis and L. braziliensis was assessed by an in vitro assay (Campolina et al., 2020). The results showed an antiparasitic effect for nine bacterial genera. The most harmful genera were Lysinibacillus and Serratia, which killed all Leishmania species within 48 and 72 hours, respectively, highlighting these genera as potential candidates for infection control (Campolina et al., 2020).
In summary, microbiota bacteria can affect sand fly susceptibility to Leishmania infection. Some microbiota bacteria have a negative effect on the parasite, possibly due to the activation of ROS production and/or by a direct antiparasitic effect. Understanding these relationships can potentially lead to the development of novel strategies for the control and prevention of leishmaniasis.
3.3 Tsetse flies: the multiple endosymbiont hosts
Tsetse flies (Diptera: Glossinidae) are strict hematophagous insects that transmit the protozoan Trypanosoma brucei, the causative agent of human African trypanosomiasis (HAT, also known as sleeping sickness) and animal African trypanosomiasis (AAT, also known as nagana). Tsetse flies belong to the genus Glossina and present a viviparous reproduction, in which larvae hatch in the female’s reproductive tract and are nourished through a secretion produced by reproductive accessory glands (the milk glands). After completing their development, larvae are deposited and immediately pupate (reviewed by Geiger et al., 2015).
The microbiota of tsetse flies is composed of both maternally-transmitted endosymbionts and commensals that they acquire from the environment. Each tsetse species harbors one to three endogenous symbionts, namely, Wigglesworthia, Sodalis and Wolbachia (reviewed by Wang et al., 2013b). Wigglesworthia is an obligate mutualist bacterium located in a specialized organ in the anterior midgut of the insect, the bacteriome. The Wigglesworthia genome encodes a range of cofactors, prosthetic groups, and carriers, suggesting that this bacterium provides the components absent in the blood meal to its arthropod hosts (Rio et al., 2012). In addition, Wigglesworthia plays a role in immune system maturation, as in its absence, larvae develop into immune compromised adults, which are also more susceptible to T. brucei infection (Wang et al., 2009; Weiss et al., 2011; Weiss et al., 2012; Weiss et al., 2013). Indeed, phagocytic hemocytes are absent in Wigglesworthia-free flies (Weiss et al., 2012). Moreover, aposymbiotic flies do not exhibit the upregulation of immune genes when challenged with nonpathogenic Escherichia coli, succumbing to infection (Weiss et al., 2012). Wigglesworthia-free flies, as well as flies infected with T. brucei, express lower levels of peptidoglycan recognition protein LB (PGRP-LB). RNAi-mediated silencing of PGRB-LB triggers the activation of the IMD pathway with a consequent production of the AMP attacin, diminishing Wigglesworthia loads (Wang et al., 2009) (Figure 1E). Conversely, the trypanosome density was higher in PGRB-LB-silenced flies. Together, these results suggest that PGRP-LB binds to Wigglesworthia peptidoglycan (PGN), preventing exacerbated activation of the insect immune system (Figure 1E). PGRP-LB may also exert a direct anti-T. brucei effect, reducing parasite loads (Wang et al., 2009) (Figure 1E). Aposymbiotic adults also present a structurally compromised PM, which is associated with earlier but ineffective activation of the fly immune system (Weiss et al., 2013).
Wigglesworthia is vertically transmitted to intrauterine larvae via milk gland secretions, as well as Sodalia. However, in contrast to Wigglesworthia, Sodalia colonizes several tsetse organs, in addition to the midgut, and its presence correlates with higher susceptibility to T. brucei (Farikou et al., 2010; Soumana et al., 2013; Kallu et al., 2023). Interestingly, the density of Sodalis declines in the absence of Wigglesworthia (Wang et al., 2013a), possibly because Sodalis requires thiamine produced by Wigglesworthia (Snyder et al., 2010). The third endosymbiont, Wolbachia, is a pathogen that colonizes tsetse gonads (reviewed by Geiger et al., 2015) and causes cytoplasmic incompatibility (detailed in Section 4.1).
In addition to endosymbionts, the most common bacterial genera in tsetse flies are Enterobacter, Enterococcus, and Acinetobacter (Soumana et al., 2013). The analyses of the microbiota composition of tsetse flies from two HAT/AAT endemic areas (Kafue in Zambia and Hurungwe in Zimbabwe) in sub-Saharan Africa revealed that the bacterial diversity is relatively similar in flies of both sexes, fed on different mammal species, and infected or not infected with T. brucei (Gaithuma et al., 2020). Conversely, significant differences were observed in microbiota diversity and composition among tsetse species, between teneral and nonteneral flies, and between infected and noninfected flies collected in Campo (Camaroon) (Tsakeng et al., 2022). The primary symbiont Wigglesworthia was present in almost all samples, with a relative abundance of approximately 47%. Interestingly, Serratia or Burkholderia seems to be a substitute for Wigglesworthia in some Glossina tachinoides flies (Tsakeng et al., 2022).
In summary, tsetse flies can harbor multiple endosymbionts, which seem to exert opposite effects on the establishment of T. brucei. Indeed, while Wigglesworthia is essential to the maturation and activation of the insect immune system, reducing the parasite loads, Sodalia is associated with higher susceptibility to infection.
3.4 Kissing bugs and Trypanosoma cruzi: a pathogen adapted to different vector species
The subfamily Triatominae (Hemiptera: Reduviidae) is a diverse group of hematophagous insects comprising 157 species (De Paiva et al., 2022). Commonly known as kissing bugs, these insects are vectors of the protozoan Trypanosoma cruzi, the causative agent of Chagas disease. The genera Rhodnius, Triatoma and Panstrongylus are particularly important from an epidemiological point of view (Coura and Vinas, 2010).
Several studies have reported that the microbiota of triatomines typically exhibits low taxonomic complexity and high specificity to the vector species (Da Mota et al., 2012; Gumiel et al., 2015; Rodriguez-Ruano et al., 2018; Arias-Giraldo et al., 2020; Brown et al., 2020). A metagenomic analysis of the microbiota of adult specimens of Panstrongylus, Rhodnius, Triatoma, and Psammolestes collected in the field revealed that Proteobacteria, Actinobacteria, Firmicutes, and Bacteroidetes accounted for more than 90% of the identified phyla (Arias-Giraldo et al., 2020). The bacterial composition correlated with the insect species but not with the presence or absence of T. cruzi (Arias-Giraldo et al., 2020). Indeed, the correlation between the microbiota composition and T. cruzi infection is controversial and seems to depend on the triatomine species and habitat (Gumiel et al., 2015; Rodriguez-Ruano et al., 2018; Waltmann et al., 2019; Eberhard et al., 2022).
Compositional changes in the microbiota are also observed along the ontogenetic development of field-collected Triatoma spp., from high diversity in the early instars to low diversity usually dominated by a single species of symbiotic bacteria in adults (Brown et al., 2020). This reduction in diversity may be related to the molting process, during which insects shed the foregut and hindgut cuticles, resulting in the loss of symbiotic bacteria (Brown et al., 2020). The abundance of Enterococcus, Acinetobacter, and Arsenophonus also differs among the development stages of laboratory-reared Rhodnius prolixus (Rodriguez-Ruano et al., 2018).
The symbiotic gram-positive bacterium Rhodococcus rhodnii, identified in R. prolixus and Triatoma spp., has been shown to play a role in insect development (reviewed by Salcedo-Porras et al., 2020). For instance, aposymbiotic nymphs of R. prolixus (hatched from surface-sterilized eggs and fed on sterile blood) as well as nymphs fed on R. rhodnii-immunized rabbits exhibited slower developmental rates and compromised ecdysis to the adult stage. The feeding of aposymbiotic nymphs with blood supplemented with B-complex vitamins can restore their ability to develop into adults (Lake and Friend, 1968). Serratia marcescens is also frequently found in triatomines. Interestingly, S. marcescens strains isolated from R. prolixus were shown to exert in vitro lytic activity against T. cruzi epimastigotes (Castro et al., 2007). On the other hand, the load of S. marcenses in the midgut of R. prolixus decreases upon T. cruzi infection (Vieira et al., 2016). Treatment with antibiotics, leading to the elimination of both R. rhodnii and S. marcenses, upregulates the expression of defensins in the midgut and fatty body of R. prolixus (Batista et al., 2021). The same pattern of AMP gene expression is observed in the fat body. In addition, phenoloxidase activity is higher in the hemolymph of insects treated with antibiotics than in the control group, suggesting that modifications in the microbiota may also cause systemic alterations (Batista et al., 2021).
The genome of R. prolixus lacks canonical components of the IMD pathway (Mesquita et al., 2015). Nevertheless, this pathway is functional and regulates the expression of defensin A. In addition, silencing the Relish gene (rpRelish) increases the load of R. rhodinii but does not affect T. cruzi infection (Figure 1F). Similarly, silencing of the Dorsal gene, the transcription factor of the Toll pathway, also does not alter T. cruzi infection (Figure 1F) (Mesquita et al., 2015). Years later, Salcedo-Porras et al. (2019) identified additional components of the R. prolixus IMD pathway using reciprocal BLAST and HMM profile searches (Salcedo-Porras et al., 2019).
In T. infestans, an antimicrobial protein named TiAP is upregulated by infection with T. cruzi (Buarque et al., 2016). Knockdown of TiAP by RNAi increased the load of microbiota bacteria but did not interfere with the parasite burden (Buarque et al., 2016). The recombinant molecule also exhibited bacteriostatic activity in vitro but was inactive against the parasite. These results suggest that T. cruzi upregulates TiAP expression to reduce bacterial load in the triatomine gut, favoring its own colonization (Buarque et al., 2016). Similarly, silencing the lectin RpLec of R. prolixus led to an increased number of bacteria but had no effect on the parasite (Araujo et al., 2021).
Briefly, triatomine immune system components seem to exert an effect on microbiota bacteria without affecting T. cruzi, suggesting that the parasite has adapted to resist the vector immune system. Moreover, infection diminishes the load of certain microbiota components, such as S. marcescens, which, in turn, may exert a direct antiparasitic effect.
3.5 Obligatory hematophagous ticks
Ticks (Acari: Ixodida) are ectoparasitic arthropods that feed on the blood of vertebrate hosts and transmit various pathogens that cause life-threatening diseases to humans and other animals (Dantas-Torres et al., 2012). The tick microbiota is composed of vertically transmitted endosymbionts as well as commensal microorganisms that are acquired from the tick habitat. In addition, tick microbiota composition varies, for instance, according to species, life cycle stage, feeding status, and geographical location (reviewed by Bonnet and Pollet, 2020). As described for insect vectors, the tick microbiota also plays a role in arthropod physiology. For example, treatment with antibiotics with a consequent reduction and/or alteration of the microbiota exerted a negative impact on the development of Rhipicephalus microplus (Guizzo et al., 2017), on the reproductive fitness of Dermacentor andersoni (Clayton et al., 2015), Rhipicephalus haemaphysaloides (Li et al., 2018), and Amblyomma americanum (Zhong et al., 2007), and on the feeding fitness of Ixodes ricinus (Guizzo et al., 2022b).
The tick microbiota also impacts vector competence. The tick Ixodes scapularis, which transmits Borrelia burgdorferi (causative agent of Lyme disease) and Anaplasma phagocytophilum (causative agent of human granulocytic anaplasmosis) (Dantas-Torres et al., 2012), shows differences in the microbiota of larvae hatched in a sterile environment compared to normal conditions (Narasimhan et al., 2014). This microbiota perturbation causes the downregulation of STAT, resulting in reduced expression of peritrophin-1, an effector of the JAK/STAT immune signaling pathway that composes the PM (Figure 1G). The consequent reduction in the thickness of the PM results in diminished B. burgdorferi infection (Narasimhan et al., 2014). B. burgdorferi upregulates the expression of an I. scapularis gene encoding a secreted reeler-domain containing protein (PIXR), which inhibits biofilm formation by gram-positive bacteria, favoring borrelial colonization (Narasimhan et al., 2017). Infection with A. phagocytophilum upregulates the expression of the I. scapularis anti-freezing protein (IAFGP), affecting bacterial biofilm formation and altering the microbiota composition (Abraham et al., 2017). The alteration of the natural microbiota composition leads to the formation of a thinner PM (Narasimhan et al., 2014), favoring A. phagocytophilum colonization (Abraham et al., 2017) (Figure 1G). The microbiota composition of Haemaphysalis longicornis nymphs is also altered by antibiotic treatment, reducing the thickness of the PM and increasing the transstadial transmission of Babesia microti to the adult stage (Wei et al., 2021). Therefore, microbiota alterations with a consequent reduction in the thickness of the PM seem to impair infection by the extracellular pathogen B. burgdorferi (Narasimhan et al., 2014) but favor the establishment of the intracellular pathogens A. phagocytothilum (Abraham et al., 2017) and B. microti (Wei et al., 2021).
The tick domesticated amidase effector 2 (Dae2), acquired during evolution by bacterial horizontal transfer (Chou et al., 2015), has been reported to rapidly kill ingested commensal host skin bacteria in the I. scapularis gut (Figure 1G). However, Dae2 shows no direct activity against Borrelia spirochetes (Hayes et al., 2020). Rapid clearance of both gram-positive and gram-negative bacteria was also observed in the gut of unfed Ixodes ricinus females upon artificial feeding (Guizzo et al., 2022a). This clearance may be due to the production of ROS, exogenous hemoglobin fragments (hemocidins) and endogenous AMPs (reviewed by Fogaça et al., 2021).
The ticks Amblyomma aureolatum and Amblyomma sculptum are vectors of Rickettsia rickettsii [the causative agent of Brazilian spotted fever (Labruna, 2009)]. A. aureolatum harbors a robust gut microbiota, predominantly composed of the Francisella genus (Pavanelo et al., 2020) and is highly susceptible to infection (Labruna et al., 2008; Martins et al., 2017). On the other hand, A. sculptum has a much lower number of bacteria in the gut (Pavanelo et al., 2020) and is less susceptible to R. rickettsii (Labruna et al., 2008; Martins et al., 2017). The gut transcriptional response to R. rickettsii infection also differs between these two species, with A. aureolatum showing mostly downregulated genes and A. sculptum showing mostly upregulated genes, including immune factors (Martins et al., 2017). In Dermacentor andersoni, the presence of the Francisella endosymbiont positively influenced the establishment of Francisella novicida (Gall et al., 2016). These results suggest that similar endosymbionts may suppress the tick immune system, facilitating the acquisition of pathogenic bacteria.
Overall, these findings highlight the significant role of the gut microbiota in tick physiology as well as in the modulation of tick immune responses and the synthesis of the PM, specifically modulating the establishment of pathogens.
4 The microbiota as a biotech resource for the control of arthropods and arthropod-borne pathogens: some examples
4.1 Wolbachia as a promising tool for the successful microbiological control of vectors
Wolbachia pipientis is a widely distributed member of the phylum Arthropoda and is estimated to be present in approximately 40% of terrestrial species (Zug and Hammerstein, 2012). Wolbachia is an obligate intracellular bacterium that is maternally transmitted and provides an advantage to infected females, facilitating its spread to uninfected populations. This bacterium alters arthropod reproduction, causing male feminization and mortality, parthenogenesis, and cytoplasmic incompatibility (CI) (reviewed by Porter and Sullivan, 2023). CI occurs when Wolbachia-infected males mate with either uninfected females (unidirectional CI) or females infected with a different and incompatible Wolbachia strain (bidirectional CI), resulting in a drastic reduction in egg hatching. Wolbachia also exhibits the ability to reduce pathogen loads, a mechanism known as pathogen interference (PI). Due to the detrimental effects of Wolbachia on arthropod reproduction and vector competence, there are currently several ongoing programs involving the release of Ae. aegypti mosquitoes, previously colonized with Wolbachia strains in the laboratory in dengue endemic areas (reviewed by Caragata et al., 2016).
The strains wAlbB (Liang et al., 2022) and wMel (Ross et al., 2022) of Wolbachia, when introduced in mosquito colonies, established a stable association with Ae. aegypti. Importantly, Wolbachia reduces CHIKV, ZIKV and DENV infection in mosquitoes (Moreira et al., 2009; Van Den Hurk et al., 2012; Liang et al., 2022). The Wolbachia strain wAlbB also established a stable association with Anopheles stephensi, conferring high resistance to Plasmodium infection (Bian et al., 2013). On the other hand, the wPip strain of Wolbachia does not inhibit infection of Ae. aegypti with DENV (Fraser et al., 2020).
Wolbachia upregulates the expression of Toll pathway components and AMPs in Ae. aegypti, which is associated with reduced DENV infection (Bian et al., 2010). Indeed, RNAi-mediated silencing of Cactus, an inhibitor of the Toll pathway, reduces viral load, while silencing of the adaptor myeloid differentiation primary response 88 (MyD88) has the opposite effect (Xi et al., 2008). In Culex pipiens pallens, colonization by new Wolbachia strains leads to higher transcript levels of components of both the Toll and IMD pathways than in mosquitoes naturally colonized with Wolbachia. These results suggest that Wolbachia induces a strong immune response in new hosts, which may play a role in the acquisition of pathogens (Zhang et al., 2020). Additional studies are warranted to fully understand how Wolbachia alters arthropod vector competence.
4.2 Paratransgenesis for the control of vector-borne pathogens
Paratransgenesis is a technique in which a member of the arthropod microbiota is genetically modified to produce antimicrobial molecules that inhibit the replication of or kill a targeted pathogen (Figure 2A). However, successful paratransgenesis requires careful selection of the bacterial species based on several key criteria (reviewed by Ratcliffe et al., 2022), including:
(i) Harmlessness: The selected bacterium should not be harmful to humans or other organisms;
(ii) Mutualistic or commensal relationship: It should be a member of the native microbiota, having a mutually beneficial or commensal symbiotic relationship with the arthropod;
(iii) No impact on vector fitness: The bacterium should not negatively affect the fitness of the arthropod vector;
(iv) Transstadial transmission: The bacterium should be able to be transmitted from one life stage to another within the vector;
(v) Cultivability and genetic manipulability: It should be cultivable in the laboratory and amenable to genetic manipulation for the expression and secretion of the desired antimicrobial molecule;
(vi) Localization: The bacterium should occupy the same organ or tissue as the targeted pathogen in the arthropod vector, facilitating contact between the modified bacterium and the antiparasitic compound.
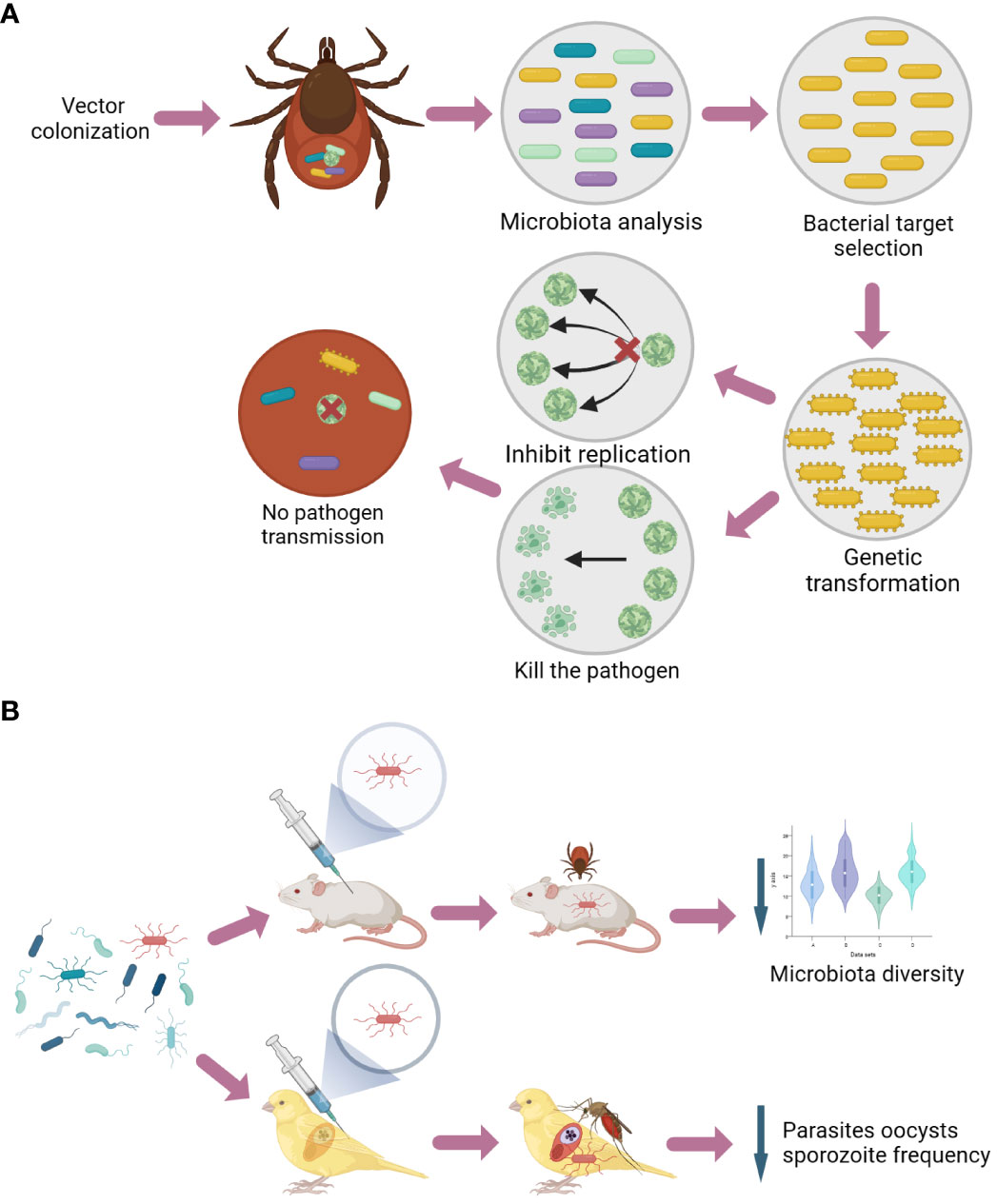
Figure 2 Schematic representation of vector control focused on the microbiota. The general methodology and application of paratransgenesis, a novel method targeting the vector microbiota, are displayed. Initially, microbiota analysis is conducted to identify potential targets within the microbial communities associated with the vector population. Subsequently, specific bacteria are chosen for genetic manipulation to carry traits capable of killing pathogens and inhibiting their replication. These modifications lead to a reduction in the pathogen transmission trait within the vector population (A). General methodology and application of an anti-microbiota vaccine. Here, microbiota analysis is used to identify suitable bacterial candidates (e.g., keystone taxa), and then model hosts such as mice and canaries are vaccinated with the identified bacteria. Once an immune response is triggered toward the bacteria of interest, the anti-microbiota antibodies induced by the vaccine act on the vector microbiota, modulating them and reducing pathogen infection within the vectors (B).
Once the bacterium is selected, it needs to be isolated and genetically transformed to produce either a peptide or a dsRNA molecule that can block the development of a targeted pathogen. Finally, the modified bacterium must be spread within the vector population through horizontal and/or vertical transmission, ultimately disrupting the pathogen’s life cycle.
Paratransgenesis has already been used to modify the symbiont R. rhodnii of R. prolixus to express the AMP cecropin A [from the moth Hyalophora cecropia (Boman and Hultmark, 1987)]. Insect colonization with the engineered bacterium limited T. cruzi infection without affecting the vector’s fitness (Durvasula et al., 1997). In mosquitoes, several bacteria, including Wolbachia, have been genetically modified to target Plasmodium (reviewed by Wilke and Marrelli, 2015). In an elegant study, Serratia and Anopheles stephensi mosquitoes were genetically modified (Huang et al., 2022) to produce two effectors: (i) the midgut peptide 2 (MP2) dodecapeptide, which binds to the mosquito midgut epithelium and inhibits P. falciparum invasion (Vega-Rodriguez et al., 2014), and (ii) scorpine, a scorpion AMP with anti-Plasmodium activity (Conde et al., 2000). Although reduced Plasmodium berghei acquisition and transmission have been observed in transgenic mosquitoes as well as in mosquitoes colonized by engineered Serratia, the combination of transgenesis and paratransgenesis shows a more effective result (Huang et al., 2022). In ticks, Sphingomonas was modified to express the major surface protein 4 (MSP4) of A. phagocytophilum, reducing the acquisition of this bacterium by I. scapularis (Mazuecos et al., 2023). The success of paratransgenesis in reducing infection in different arthropods highlights this method as a promising approach to control VBDs.
4.3 Vector microbiota modulation by host antibodies: anti-microbiota vaccines
Antibiotic treatments have provided some insights into the role of the microbiota in both arthropod fitness and vector competence. However, their use raises up some concerns. For instance, it was recently demonstrated that a simple antibiotic microinjection is not sufficient to clear Rickettsia buchnerii from I. scapularis (Oliver et al., 2021) or Midichloria mitochondrii endosymbionts from I. ricinus ovaries (Guizzo et al., 2022b). Caution also needs to be exercised when using high dosages of antibiotics, especially tetracycline, as observed phenotypes may result from their off-target activity of inhibiting arthropod mitochondrial protein synthesis (reviewed by Moullan et al., 2015). In addition, antibiotic treatment affects multiple bacterial species simultaneously, making it challenging to establish causal links between specific taxa and tick fitness/vector competence alterations. To overcome this limitation, and taking the advantage of the fact that host immunoglobulins taken with the blood meal remain functional in tick hemolymph (Vaz Junior et al., 1996; Maitre et al., 2022), recent research has introduced anti-microbiota vaccines as a tool to modify arthropod microbiota composition (Mateos-Hernandez et al., 2020; Mateos-Hernandez et al., 2021; Wu-Chuang et al., 2021; Azelyte et al., 2022; Maitre et al., 2022).
The immunization of mice with live E. coli, a representative of Enterobacteriaceae (a keystone taxon in ticks; Mateos-Hernandez et al., 2020), results in the production of anti-E. coli antibodies. The gut of ticks fed on immunized animals shows reduced Escherichia abundance and microbiota diversity (Mateos-Hernandez et al., 2021) (Figure 2B). Feeding Culex quinquefasciatus mosquitoes on E. coli-immunized canaries infected with Plasmodium relictum also resulted in significant alterations in the abundance of several microbiota taxa (Azelyte et al., 2022). Moreover, mosquitoes fed on immunized birds showed reduced frequency and numbers of parasite oocysts in the midgut and a lower sporozoite frequency in salivary glands, indicating the potential for altering vector competence (Azelyte et al., 2022) (Figure 2B). Importantly, immunization with E. coli in mice (Mateos-Hernandez et al., 2021) and birds (Azelyte et al., 2022) showed no adverse effects or alterations of the fecal microbiota, indicating the safety and specificity of the anti-microbiota vaccines. Therefore, this strategy offers a promising approach for selectively modifying microbiota composition and potentially controlling VBDs.
5 Concluding remarks
In conclusion, the microbiota plays a central role in arthropod physiology as well as in shaping vector competence, mostly by modulating of the arthropod immune system and the production of structural components, such as the PM. In addition, some microbiota members can directly affect pathogens by the secretion of antiparasitic substances and/or competition for nutrients. Similarly, some pathogens can negatively affect the arthropod microbiota to favor its own establishment.
Antibiotic treatment has provided insights into the role of the microbiota; however, the nonspecific nature of these treatments limits the ability to establish the function of specific members. Recent advances in anti-microbiota vaccines offer a promising approach for selectively altering the microbiota composition. Other approaches, such as arthropod colonization with Wolbachia or engineered bacteria, have also shown effective results in infection control. Further studies are needed to pave the way for the development of innovative strategies targeting the arthropod microbiota, allowing the control of VBDs.
Author contributions
DP: Conceptualization, Writing – original draft, Writing – review & editing. EP-S: Writing – original draft, Writing – review & editing. AM: Writing – original draft, Writing – review & editing. LA-D: Writing – original draft, Writing – review & editing. PK: Writing – original draft, Writing – review & editing, Funding acquisition. AC-C: Funding acquisition, Writing – original draft, Writing – review & editing, Supervision. AF: Conceptualization, Funding acquisition, Supervision, Writing – original draft, Writing – review & editing.
Funding
The author(s) declare financial support was received for the research, authorship, and/or publication of this article. This work was supported by a cooperation agreement between the São Paulo Research Foundation (FAPESP; Grants 2021/03649-4) and the Czech Science Foundation (GACR; Grants 22-12648J); the National Council for Scientific and Technological Development [CNPq; Grants CNPq 573959/2008-0; The National Institutes of Science and Technology Program in Molecular Entomology (INCT-EM)]; the French Government’s Investissement d’Avenir program, Laboratoire d’Excellence “Integrative Biology of Emerging Infectious Diseases” (Grant ANR-10-LABX-62-IBEID). DP was supported by a fellowship from FAPESP (2018/00652-1). AF received a CNPq research productivity fellowship (309733/2018-9) and a research fellowship from FAPESP (2022/08257-0).
Acknowledgments
Figures 1 and 2 were created with Biorender.com.
Conflict of interest
The authors declare that the research was conducted in the absence of any commercial or financial relationships that could be construed as a potential conflict of interest.
The author(s) declared that they were an editorial board member of Frontiers, at the time of submission. This had no impact on the peer review process and the final decision.
Publisher’s note
All claims expressed in this article are solely those of the authors and do not necessarily represent those of their affiliated organizations, or those of the publisher, the editors and the reviewers. Any product that may be evaluated in this article, or claim that may be made by its manufacturer, is not guaranteed or endorsed by the publisher.
Footnotes
References
Abraham N. M., Liu L., Jutras B. L., Yadav A. K., Narasimhan S., Gopalakrishnan V., et al. (2017). Pathogen-mediated manipulation of arthropod microbiota to promote infection. Proc. Natl. Acad. Sci. U.S.A. 114, E781–E790. doi: 10.1073/pnas.1613422114
Adair K. L., Wilson M., Bost A., Douglas A. E. (2018). Microbial community assembly in wild populations of the fruit fly Drosophila melanogaster. ISME J. 12, 959–972. doi: 10.1038/s41396-017-0020-x
Araujo C., Pacheco J. P. F., Waniek P. J., Geraldo R. B., Sibajev A., Dos Santos A. L., et al. (2021). A rhamnose-binding lectin from Rhodnius prolixus and the impact of its silencing on gut bacterial microbiota and Trypanosoma cruzi. Dev. Comp. Immunol. 114, 103823. doi: 10.1016/j.dci.2020.103823
Arias-Giraldo L. M., Munoz M., Hernandez C., Herrera G., Velasquez-Ortiz N., Cantillo-Barraza O., et al. (2020). Species-dependent variation of the gut bacterial communities across Trypanosoma cruzi insect vectors. PloS One 15, e0240916. doi: 10.1371/journal.pone.0240916
Azelyte J., Wu-Chuang A., Ziegyte R., Platonova E., Mateos-Hernandez L., Maye J., et al. (2022). Anti-microbiota vaccine reduces avian malaria infection within mosquito vectors. Front. Immunol. 13. doi: 10.3389/fimmu.2022.841835
Bahia A. C., Oliveira J. H., Kubota M. S., Araujo H. R., Lima J. B., Rios-Velasquez C. M., et al. (2013). The role of reactive oxygen species in Anopheles aquasalis response to Plasmodium vivax infection. PloS One 8, e57014. doi: 10.1371/journal.pone.0057014
Barletta A. B., Nascimento-Silva M. C., Talyuli O. A., Oliveira J. H., Pereira L. O., Oliveira P. L., et al. (2017). Microbiota activates IMD pathway and limits Sindbis infection in Aedes aEgypti. Parasit Vectors 10, 103. doi: 10.1186/s13071-017-2040-9
Batista K. K. S., Vieira C. S., Figueiredo M. B., Costa-Latge S. G., Azambuja P., Genta F. A., et al. (2021). Influence of Serratia marcescens and Rhodococcus rhodnii on the humoral immunity of Rhodnius prolixus. Int. J. Mol. Sci. 22, 10901. doi: 10.3390/ijms222010901
Bian G., Joshi D., Dong Y., Lu P., Zhou G., Pan X., et al. (2013). Wolbachia invades Anopheles stephensi populations and induces refractoriness to Plasmodium infection. Science 340, 748–751. doi: 10.1126/science.1236192
Bian G., Xu Y., Lu P., Xie Y., Xi Z. (2010). The endosymbiotic bacterium Wolbachia induces resistance to dengue virus in Aedes aEgypti. PloS Pathog. 6, e1000833. doi: 10.1371/journal.ppat.1000833
Blandin S., Shiao S. H., Moita L. F., Janse C. J., Waters A. P., Kafatos F. C., et al. (2004). Complement-like protein TEP1 is a determinant of vectorial capacity in the malaria vector Anopheles Gambiae. Cell 116, 661–670. doi: 10.1016/s0092-8674(04)00173-4
Blum J. E., Fischer C. N., Miles J., Handelsman J. (2013). Frequent replenishment sustains the beneficial microbiome of Drosophila melanogaster. mBio 4, e00860–e00813. doi: 10.1128/mBio.00860-13
Boissiere A., Tchioffo M. T., Bachar D., Abate L., Marie A., Nsango S. E., et al. (2012). Midgut microbiota of the malaria mosquito vector Anopheles Gambiae and interactions with Plasmodium falciparum infection. PloS Pathog. 8, e1002742. doi: 10.1371/journal.ppat.1002742
Boman H. G., Hultmark D. (1987). Cell-free immunity in insects. Annu. Rev. Microbiol. 41, 103–126. doi: 10.1146/annurev.mi.41.100187.000535
Bonnet S. I., Pollet T. (2020). Update on the intricate tango between tick microbiomes and tick-borne pathogens. Parasite Immunol. 43, e12813. doi: 10.1111/pim.12813
Brown J. J., Rodriguez-Ruano S. M., Poosakkannu A., Batani G., Schmidt J. O., Roachell W., et al. (2020). Ontogeny, species identity, and environment dominate microbiome dynamics in wild populations of kissing bugs (Triatominae). Microbiome 8, 146. doi: 10.1186/s40168-020-00921-x
Buarque D. S., Gomes C. M., Araujo R. N., Pereira M. H., Ferreira R. C., Guarneri A. A., et al. (2016). A new antimicrobial protein from the anterior midgut of Triatoma infestans mediates Trypanosoma cruzi establishment by controlling the microbiota. Biochimie 123, 138–143. doi: 10.1016/j.biochi.2016.02.009
Campolina T. B., Villegas L. E. M., Monteiro C. C., Pimenta P. F. P., Secundino N. F. C. (2020). Tripartite interactions: Leishmania, microbiota and Lutzomyia longipalpis. PloS Negl. Trop. Dis. 14, e0008666. doi: 10.1371/journal.pntd.0008666
Caragata E. P., Dutra H. L. C., Moreira L. A. (2016). Exploiting intimate relationships: controlling mosquito-transmitted disease with Wolbachia. Trends Parasitol. 32, 207–218. doi: 10.1016/j.pt.2015.10.011
Caragata E. P., Tikhe C. V., Dimopoulos G. (2019). Curious entanglements: interactions between mosquitoes, their microbiota, and arboviruses. Curr. Opin. Virol. 37, 26–36. doi: 10.1016/j.coviro.2019.05.005
Castro D. P., Seabra S. H., Garcia E. S., De Souza W., Azambuja P. (2007). Trypanosoma cruzi: ultrastructural studies of adhesion, lysis and biofilm formation by Serratia marcescens. Exp. Parasitol. 117, 201–207. doi: 10.1016/j.exppara.2007.04.014
Chandler J. A., Lang J. M., Bhatnagar S., Eisen J. A., Kopp A. (2011). Bacterial communities of diverse Drosophila species: ecological context of a host-microbe model system. PloS Genet. 7, e1002272. doi: 10.1371/journal.pgen.1002272
Chou S., Daugherty M. D., Peterson S. B., Biboy J., Yang Y., Jutras B. L., et al. (2015). Transferred interbacterial antagonism genes augment eukaryotic innate immune function. Nature 518, 98–101. doi: 10.1038/nature13965
Clayton K. A., Gall C. A., Mason K. L., Scoles G. A., Brayton K. A. (2015). The characterization and manipulation of the bacterial microbiome of the Rocky Mountain wood tick, Dermacentor andersoni. Parasit Vectors 8, 632. doi: 10.1186/s13071-015-1245-z
Conde R., Zamudio F. Z., Rodriguez M. H., Possani L. D. (2000). Scorpine, an anti-malaria and anti-bacterial agent purified from scorpion venom. FEBS Lett. 471, 165–168. doi: 10.1016/s0014-5793(00)01384-3
Coura J. R., Vinas P. A. (2010). Chagas disease: a new worldwide challenge. Nature 465, S6–S7. doi: 10.1038/nature09221
Da Mota F. F., Marinho L. P., Moreira C. J., Lima M. M., Mello C. B., Garcia E. S., et al. (2012). Cultivation-independent methods reveal differences among bacterial gut microbiota in triatomine vectors of Chagas disease. PloS Negl. Trop. Dis. 6, e1631. doi: 10.1371/journal.pntd.0001631
Dantas-Torres F., Chomel B. B., Otranto D. (2012). Ticks and tick-borne diseases: a One Health perspective. Trends Parasitol. 28, 437–446. S1471-4922(12)00121-3. doi: 10.1016/j.pt.2012.07.003
De Paiva V. F., Belintani T., De Oliveira J., Galvao C., Da Rosa J. A. (2022). A review of the taxonomy and biology of Triatominae subspecies (Hemiptera: Reduviidae). Parasitol. Res. 121, 499–512. doi: 10.1007/s00436-021-07414-2
Depaquit J., Grandadam M., Fouque F., Andry P. E., Peyrefitte C. (2010). Arthropod-borne viruses transmitted by Phlebotomine sandflies in Europe: a review. Euro Surveill 15, 19507. doi: 10.2807/ese.15.10.19507-en
Diaz-Albiter H., Sant'anna M. R., Genta F. A., Dillon R. J. (2012). Reactive oxygen species-mediated immunity against Leishmania mexicana and Serratia marcescens in the sand phlebotomine fly Lutzomyia longipalpis. J. Biol. Chem. 287, 23995–24003. doi: 10.1074/jbc.M112.376095
Dickson L. B., Jiolle D., Minard G., Moltini-Conclois I., Volant S., Ghozlane A., et al. (2017). Carryover effects of larval exposure to different environmental bacteria drive adult trait variation in a mosquito vector. Sci. Adv. 3, e1700585. doi: 10.1126/sciadv.1700585
Dong Y., Manfredini F., Dimopoulos G. (2009). Implication of the mosquito midgut microbiota in the defense against malaria parasites. PloS Pathog. 5, e1000423. doi: 10.1371/journal.ppat.1000423
Douglas A. E. (2014). The molecular basis of bacterial-insect symbiosis. J. Mol. Biol. 426, 3830–3837. doi: 10.1016/j.jmb.2014.04.005
Du E. J., Ahn T. J., Kwon I., Lee J. H., Park J. H., Park S. H., et al. (2016). TrpA1 regulates defecation of food-borne pathogens under the control of the DUOX Pathway. PloS Genet. 12, e1005773. doi: 10.1371/journal.pgen.1005773
Durvasula R. V., Gumbs A., Panackal A., Kruglov O., Aksoy S., Merrifield R. B., et al. (1997). Prevention of insect-borne disease: an approach using transgenic symbiotic bacteria. Proc. Natl. Acad. Sci. U.S.A. 94, 3274–3278. doi: 10.1073/pnas.94.7.3274
Eberhard F. E., Klimpel S., Guarneri A. A., Tobias N. J. (2022). Exposure to Trypanosoma parasites induces changes in the microbiome of the Chagas disease vector Rhodnius prolixus. Microbiome 10, 45. doi: 10.1186/s40168-022-01240-z
Farikou O., Njiokou F., Mbida Mbida J. A., Njitchouang G. R., Djeunga H. N., Asonganyi T., et al. (2010). Tripartite interactions between tsetse flies, Sodalis glossinidius and trypanosomes - an epidemiological approach in two historical human African trypanosomiasis foci in Cameroon. Infect. Genet. Evol. 10, 115–121. doi: 10.1016/j.meegid.2009.10.008
Fogaça A. C., Sousa G., Pavanelo D. B., Esteves E., Martins L. A., Urbanova V., et al. (2021). Tick immune system: what is known, the interconnections, the gaps, and the challenges. Front. Immunol. 12. doi: 10.3389/fimmu.2021.628054
Fraihi W., Fares W., Perrin P., Dorkeld F., Sereno D., Barhoumi W., et al. (2017). An integrated overview of the midgut bacterial flora composition of Phlebotomus perniciosus, a vector of zoonotic visceral leishmaniasis in the Western Mediterranean Basin. PloS Negl. Trop. Dis. 11, e0005484. doi: 10.1371/journal.pntd.0005484
Fraser J. E., O'donnell T. B., Duyvestyn J. M., O'neill S. L., Simmons C. P., Flores H. A. (2020). Novel phenotype of Wolbachia strain wPip in Aedes aEgypti challenges assumptions on mechanisms of Wolbachia-mediated dengue virus inhibition. PloS Pathog. 16, e1008410. doi: 10.1371/journal.ppat.1008410
Gaithuma A., Yamagishi J., Hayashida K., Kawai N., Namangala B., Sugimoto C. (2020). Blood meal sources and bacterial microbiome diversity in wild-caught tsetse flies. Sci. Rep. 10, 5005. doi: 10.1038/s41598-020-61817-2
Gall C. A., Reif K. E., Scoles G. A., Mason K. L., Mousel M., Noh S. M., et al. (2016). The bacterial microbiome of Dermacentor andersoni ticks influences pathogen susceptibility. ISME J. 10, 1846–1855. doi: 10.1038/ismej.2015.266
Garver L. S., Bahia A. C., Das S., Souza-Neto J. A., Shiao J., Dong Y., et al. (2012). Anopheles Imd pathway factors and effectors in infection intensity-dependent anti-Plasmodium action. PloS Pathog. 8, e1002737. doi: 10.1371/journal.ppat.1002737
Geiger A., Ponton F., Simo G. (2015). Adult blood-feeding tsetse flies, trypanosomes, microbiota and the fluctuating environment in sub-Saharan Africa. ISME J. 9, 1496–1507. doi: 10.1038/ismej.2014.236
Girard M., Martin E., Vallon L., Raquin V., Bellet C., Rozier Y., et al. (2021). Microorganisms associated with mosquito oviposition sites: implications for habitat selection and insect life histories. Microorganisms 9, 1589. doi: 10.3390/microorganisms9081589
Guizzo M. G., Dolezelikova K., Neupane S., Frantova H., Hrbatova A., Pafco B., et al. (2022a). Characterization and manipulation of the bacterial community in the midgut of Ixodes ricinus. Parasit Vectors 15, 248. doi: 10.1186/s13071-022-05362-z
Guizzo M. G., Hatalova T., Frantova H., Zurek L., Kopacek P., Perner J. (2022b). Ixodes ricinus ticks have a functional association with Midichloria mitochondrii. Front. Cell Infect. Microbiol. 12. doi: 10.3389/fcimb.2022.1081666
Guizzo M. G., Parizi L. F., Nunes R. D., Schama R., Albano R. M., Tirloni L., et al. (2017). A Coxiella mutualist symbiont is essential to the development of Rhipicephalus microplus. Sci. Rep. 7, 17554. doi: 10.1038/s41598-017-17309-x
Gumiel M., Da Mota F. F., Rizzo Vde S., Sarquis O., De Castro D. P., Lima M. M., et al. (2015). Characterization of the microbiota in the guts of Triatoma brasiliensis and Triatoma pseudomaculata infected by Trypanosoma cruzi in natural conditions using culture independent methods. Parasit Vectors 8, 245. doi: 10.1186/s13071-015-0836-z
Ha E. M., Oh C. T., Bae Y. S., Lee W. J. (2005a). A direct role for dual oxidase in Drosophila gut immunity. Science 310, 847–850. doi: 10.1126/science.1117311
Ha E. M., Oh C. T., Ryu J. H., Bae Y. S., Kang S. W., Jang I. H., et al. (2005b). An antioxidant system required for host protection against gut infection in Drosophila. Dev. Cell 8, 125–132. doi: 10.1016/j.devcel.2004.11.007
Hayes B. M., Radkov A. D., Yarza F., Flores S., Kim J., Zhao Z., et al. (2020). Ticks resist skin commensals with immune factor of bacterial origin. Cell 183, 1562–1571.e1512. doi: 10.1016/j.cell.2020.10.042
Herrer A., Christensen H. A. (1975). Implication of Phlebotomus sand flies as vectors of bartonellosis and leishmaniasis as early as 1764. Science 190, 154–155. doi: 10.1126/science.1101379
Huang W., Vega-Rodriguez J., Kizito C., Cha S. J., Jacobs-Lorena M. (2022). Combining transgenesis with paratransgenesis to fight malaria. Elife 11, e77584. doi: 10.7554/eLife.77584
Huber M., Cabib E., Miller L. H. (1991). Malaria parasite chitinase and penetration of the mosquito peritrophic membrane. Proc. Natl. Acad. Sci. U.S.A. 88, 2807–2810. doi: 10.1073/pnas.88.7.2807
Kallu S. A., Ndebe J., Qiu Y., Nakao R., Simuunza M. C. (2023). Prevalence and association of trypanosomes and Sodalis glossinidius in tsetse flies from the Kafue National Park in Zambia. Trop. Med. Infect. Dis. 8, 80. doi: 10.3390/tropicalmed8020080
Kelly P. H., Bahr S. M., Serafim T. D., Ajami N. J., Petrosino J. F., Meneses C., et al. (2017). The gut microbiome of the vector Lutzomyia longipalpis is essential for survival of Leishmania infantum. mBio 8, e01121-01116. doi: 10.1128/mBio.01121-16
Kleino A., Silverman N. (2014). The Drosophila IMD pathway in the activation of the humoral immune response. Dev. Comp. Immunol. 42, 25–35. doi: 10.1016/j.dci.2013.05.014
Kumar S., Molina-Cruz A., Gupta L., Rodrigues J., Barillas-Mury C. (2010). A peroxidase/dual oxidase system modulates midgut epithelial immunity in Anopheles Gambiae. Science 327, 1644–1648. doi: 10.1126/science.1184008
Labruna M. B. (2009). Ecology of rickettsia in south america. Ann. N Y Acad. Sci. 1166, 156–166. doi: 10.1111/j.1749-6632.2009.04516.x
Labruna M. B., Ogrzewalska M., Martins T. F., Pinter A., Horta M. C. (2008). Comparative susceptibility of larval stages of Amblyomma aureolatum, Amblyomma cajennense, and Rhipicephalus sanguineus to infection by Rickettsia rickettsii. J. Med. Entomol 45, 1156–1159. doi: 10.1603/0022-2585(2008)45[1156:csolso]2.0.co;2
Lake P., Friend W. G. (1968). The use of artificial diets to determine some of the effects of Nocardia rhodnii on the development of Rhodnius prolixus. J. Insect Physiol. 14, 543–562. doi: 10.1016/0022-1910(68)90070-x
Lee K. A., Kim S. H., Kim E. K., Ha E. M., You H., Kim B., et al. (2013). Bacterial-derived uracil as a modulator of mucosal immunity and gut-microbe homeostasis in Drosophila. Cell 153, 797–811. doi: 10.1016/j.cell.2013.04.009
Lee K. A., Lee W. J. (2014). Drosophila as a model for intestinal dysbiosis and chronic inflammatory diseases. Dev. Comp. Immunol. 42, 102–110. doi: 10.1016/j.dci.2013.05.005
Lemaitre B., Hoffmann J. (2007). The host defense of Drosophila melanogaster. Annu. Rev. Immunol. 25, 697–743. doi: 10.1146/annurev.immunol.25.022106.141615
Liang X., Tan C. H., Sun Q., Zhang M., Wong P. S. J., Li M. I., et al. (2022). Wolbachia wAlbB remains stable in Aedes aEgypti over 15 years but exhibits genetic background-dependent variation in virus blocking. PNAS Nexus 1, pgac203. doi: 10.1093/pnasnexus/pgac203
Li L.-H., Zhang I., Zhu D. (2018). Effects of antibiotic treatment on the fecundity of Rhipicephalus haemaphysaloides ticks. Parasit. Vectors 11, 242. doi: 10.1186/s13071-018-2807-7
Lindh J. M., Borg-Karlson A. K., Faye I. (2008). Transstadial and horizontal transfer of bacteria within a colony of Anopheles Gambiae (Diptera: Culicidae) and oviposition response to bacteria-containing water. Acta Trop. 107, 242–250. doi: 10.1016/j.actatropica.2008.06.008
Louradour I., Monteiro C. C., Inbar E., Ghosh K., Merkhofer R., Lawyer P., et al. (2017). The midgut microbiota plays an essential role in sand fly vector competence for Leishmania major. Cell Microbiol. 19, e12755. doi: 10.1111/cmi.12755
Maitre A., Wu-Chuang A., Azelyte J., Palinauskas V., Mateos-Hernandez L., Obregon D., et al. (2022). Vector microbiota manipulation by host antibodies: the forgotten strategy to develop transmission-blocking vaccines. Parasit Vectors 15, 4. doi: 10.1186/s13071-021-05122-5
Maroli M., Feliciangeli M. D., Bichaud L., Charrel R. N., Gradoni L. (2013). Phlebotomine sandflies and the spreading of leishmaniases and other diseases of public health concern. Med. Vet. Entomol 27, 123–147. doi: 10.1111/j.1365-2915.2012.01034.x
Martins L. A., Galletti M., Ribeiro J. M., Fujita A., Costa F. B., Labruna M. B., et al. (2017). The distinct transcriptional response of the midgut of Amblyomma sculptum and Amblyomma aureolatum ticks to Rickettsia rickettsii correlates to their differences in susceptibility to infection. Front. Cell Infect. Microbiol. 7. doi: 10.3389/fcimb.2017.00129
Martins K. A., Meirelles M. H. A., Mota T. F., Abbasi I., De Queiroz A. T. L., Brodskyn C. I., et al. (2021). Effects of larval rearing substrates on some life-table parameters of Lutzomyia longipalpis sand flies. PloS Negl. Trop. Dis. 15, e0009034. doi: 10.1371/journal.pntd.0009034
Mateos-Hernandez L., Obregon D., Maye J., Borneres J., Versille N., de la Fuente J., et al. (2020). Anti-tick microbiota vaccine impacts Ixodes ricinus performance during feeding. Vaccines (Basel) 8, 702. doi: 10.3390/vaccines8040702
Mateos-Hernandez L., Obregon D., Wu-Chuang A., Maye J., Borneres J., Versille N., et al. (2021). Anti-microbiota vaccines modulate the tick microbiome in a taxon-specific manner. Front. Immunol. 12. doi: 10.3389/fimmu.2021.704621
Mazuecos L., Alberdi P., Hernandez-Jarguin A., Contreras M., Villar M., Cabezas-Cruz A., et al. (2023). Frankenbacteriosis targeting interactions between pathogen and symbiont to control infection in the tick vector. iScience 26, 106697. doi: 10.1016/j.isci.2023.106697
Meister S., Agianian B., Turlure F., Relogio A., Morlais I., Kafatos F. C., et al. (2009). Anopheles Gambiae PGRPLC-mediated defense against bacteria modulates infections with malaria parasites. PloS Pathog. 5, e1000542. doi: 10.1371/journal.ppat.1000542
Mesquita R. D., Vionette-Amaral R. J., Lowenberger C., Rivera-Pomar R., Monteiro F. A., Minx P., et al. (2015). Genome of Rhodnius prolixus, an insect vector of Chagas disease, reveals unique adaptations to hematophagy and parasite infection. Proc. Natl. Acad. Sci. U.S.A. 112, 14936–14941. doi: 10.1073/pnas.1506226112
Moltini-Conclois I., Stalinski R., Tetreau G., Despres L., Lambrechts L. (2018). Larval exposure to the bacterial insecticide Bti enhances dengue virus susceptibility of adult Aedes aEgypti mosquitoes. Insects 9, 193. doi: 10.3390/insects9040193
Moreira L. A., Iturbe-Ormaetxe I., Jeffery J. A., Lu G., Pyke A. T., Hedges L. M., et al. (2009). A Wolbachia symbiont in Aedes aEgypti limits infection with dengue, Chikungunya, and Plasmodium. Cell 139, 1268–1278. doi: 10.1016/j.cell.2009.11.042
Moullan N., Mouchiroud L., Wang X., Ryu D., Williams E. G., Mottis A., et al. (2015). Tetracyclines disturb mitochondrial function across eukaryotic models: a call for caution in biomedical research. Cell Rep. 10, 1681–1691. doi: 10.1016/j.celrep.2015.02.034
Müller R. R. F., Kendrovski V., Montag D., Bonn A. (2019). “Vector-borne diseases,” in Biodiversity and health in the face of climate change. Eds. Marselle M., Stadler J., Korn H., Irvine K. (Cham: Springer), 67–90.
Narasimhan S., Rajeevan N., Liu L., Zhao Y. O., Heisig J., Pan J., et al. (2014). Gut microbiota of the tick vector Ixodes scapularis modulate colonization of the Lyme disease spirochete. Cell Host Microbe 15, 58–71. doi: 10.1016/j.chom.2013.12.001
Narasimhan S., Schuijt T. J., Abraham N. M., Rajeevan N., Coumou J., Graham M., et al. (2017). Modulation of the tick gut milieu by a secreted tick protein favors Borrelia burgdorferi colonization. Nat. Commun. 8, 184. doi: 10.1038/s41467-017-00208-0
Ohlstein B., Spradling A. (2006). The adult Drosophila posterior midgut is maintained by pluripotent stem cells. Nature 439, 470–474. doi: 10.1038/nature04333
Oliveira J. H., Goncalves R. L., Lara F. A., Dias F. A., Gandara A. C., Menna-Barreto R. F., et al. (2011). Blood meal-derived heme decreases ROS levels in the midgut of Aedes aEgypti and allows proliferation of intestinal microbiota. PloS Pathog. 7, e1001320. doi: 10.1371/journal.ppat.1001320
Oliver J. D., Price L. D., Burkhardt N. Y., Heu C. C., Khoo B. S., Thorpe C. J., et al. (2021). Growth dynamics and antibiotic elimination of symbiotic Rickettsia buchneri in the tick Ixodes scapularis (Acari: Ixodidae). Appl. Environ. Microbiol. 87, e01672–e01620. doi: 10.1128/AEM.01672-20
Pais I. S., Valente R. S., Sporniak M., Teixeira L. (2018). Drosophila melanogaster establishes a species-specific mutualistic interaction with stable gut-colonizing bacteria. PloS Biol. 16, e2005710. doi: 10.1371/journal.pbio.2005710
Pang X., Xiao X., Liu Y., Zhang R., Liu J., Liu Q., et al. (2016). Mosquito C-type lectins maintain gut microbiome homeostasis. Nat. Microbiol. 1, 16023. doi: 10.1038/nmicrobiol.2016.23
Pavanelo D. B., Schroder N. C. H., Pin Viso N. D., Martins L. A., Malossi C. D., Galletti M., et al. (2020). Comparative analysis of the midgut microbiota of two natural tick vectors of Rickettsia rickettsii. Dev. Comp. Immunol. 106, 103606. doi: 10.1016/j.dci.2019.103606
Phillips M. A., Burrows J. N., Manyando C., Van Huijsduijnen R. H., Van Voorhis W. C., Wells T. N. C. (2017). Malaria. Nat. Rev. Dis. Primers 3, 17050. doi: 10.1038/nrdp.2017.50
Ponnusamy L., Xu N., Nojima S., Wesson D. M., Schal C., Apperson C. S. (2008). Identification of bacteria and bacteria-associated chemical cues that mediate oviposition site preferences by Aedes aEgypti. Proc. Natl. Acad. Sci. U.S.A. 105, 9262–9267. doi: 10.1073/pnas.0802505105
Porter J., Sullivan W. (2023). The cellular lives of Wolbachia. Nat. Rev. Microbiol. 21, 750–766. doi: 10.1038/s41579-023-00918-x
Ratcliffe N. A., Furtado Pacheco J. P., Dyson P., Castro H. C., Gonzalez M. S., Azambuja P., et al. (2022). Overview of paratransgenesis as a strategy to control pathogen transmission by insect vectors. Parasit Vectors 15, 112. doi: 10.1186/s13071-021-05132-3
Rio R. V., Symula R. E., Wang J., Lohs C., Wu Y. N., Snyder A. K., et al. (2012). Insight into the transmission biology and species-specific functional capabilities of tsetse (Diptera: Glossinidae) obligate symbiont Wigglesworthia. mBio 3, e00240–e00211. doi: 10.1128/mBio.00240-11
Rodgers F. H., Gendrin M., Wyer C. A. S., Christophides G. K. (2017). Microbiota-induced peritrophic matrix regulates midgut homeostasis and prevents systemic infection of malaria vector mosquitoes. PloS Pathog. 13, e1006391. doi: 10.1371/journal.ppat.1006391
Rodriguez-Ruano S. M., Skochova V., Rego R. O. M., Schmidt J. O., Roachell W., Hypsa V., et al. (20181167). Microbiomes of North American Triatominae: the grounds for Chagas disease epidemiology. Front. Microbiol. 9. doi: 10.3389/fmicb.2018.01167
Romoli O., Gendrin M. (2018). The tripartite interactions between the mosquito, its microbiota and Plasmodium. Parasit Vectors 11, 200. doi: 10.1186/s13071-018-2784-x
Ross P. A., Robinson K. L., Yang Q., Callahan A. G., Schmidt T. L., Axford J. K., et al. (2022). A decade of stability for wMel Wolbachia in natural Aedes aEgypti populations. PloS Pathog. 18, e1010256. doi: 10.1371/journal.ppat.1010256
Ryu J. H., Kim S. H., Lee H. Y., Bai J. Y., Nam Y. D., Bae J. W., et al. (2008). Innate immune homeostasis by the homeobox gene caudal and commensal-gut mutualism in Drosophila. Science 319, 777–782. doi: 10.1126/science.1149357
Salcedo-Porras N., Guarneri A., Oliveira P. L., Lowenberger C. (2019). Rhodnius prolixus: identification of missing components of the IMD immune signaling pathway and functional characterization of its role in eliminating bacteria. PloS One 14, e0214794. doi: 10.1371/journal.pone.0214794
Salcedo-Porras N., Umana-Diaz C., Bitencourt R. O. B., Lowenberger C. (2020). The role of bacterial symbionts in triatomines: an evolutionary perspective. Microorganisms 8, 1438. doi: 10.3390/microorganisms8091438
Sant'anna M. R., Diaz-Albiter H., Aguiar-Martins K., Al Salem W. S., Cavalcante R. R., Dillon V. M., et al. (2014). Colonisation resistance in the sand fly gut: Leishmania protects Lutzomyia longipalpis from bacterial infection. Parasit Vectors 7, 329. doi: 10.1186/1756-3305-7-329
Snyder A. K., Deberry J. W., Runyen-Janecky L., Rio R. V. (2010). Nutrient provisioning facilitates homeostasis between tsetse fly (Diptera: Glossinidae) symbionts. Proc. Biol. Sci. 277, 2389–2397. doi: 10.1098/rspb.2010.0364
Song W., Veenstra J. A., Perrimon N. (2014). Control of lipid metabolism by tachykinin in Drosophila. Cell Rep. 9, 40–47. doi: 10.1016/j.celrep.2014.08.060
Soumana I. H., Simo G., Njiokou F., Tchicaya B., Abd-Alla A. M., Cuny G., et al. (2013). The bacterial flora of tsetse fly midgut and its effect on trypanosome transmission. J. Invertebr Pathol. 112 Suppl, S89–S93. doi: 10.1016/j.jip.2012.03.029
Strand M. R. (2018). Composition and functional roles of the gut microbiota in mosquitoes. Curr. Opin. Insect Sci. 28, 59–65. doi: 10.1016/j.cois.2018.05.008
Telleria E. L., Martins-Da-Silva A., Tempone A. J., Traub-Cseko Y. M. (2018). Leishmania, microbiota and sand fly immunity. Parasitology 145, 1336–1353. doi: 10.1017/S0031182018001014
Telleria E. L., Sant'anna M. R., Ortigao-Farias J. R., Pitaluga A. N., Dillon V. M., Bates P. A., et al. (2012). Caspar-like gene depletion reduces Leishmania infection in sand fly host Lutzomyia longipalpis. J. Biol. Chem. 287, 12985–12993. doi: 10.1074/jbc.M111.331561
Tetreau G., Grizard S., Patil C. D., Tran F. H., Tran Van V., Stalinski R., et al. (2018). Bacterial microbiota of Aedes aEgypti mosquito larvae is altered by intoxication with Bacillus thuringiensis Israelensis. Parasit Vectors 11, 121. doi: 10.1186/s13071-018-2741-8
Thorp J. H. (2009). Arthropoda and related groups. In: Encyclopedia of Insects: 2nd Edition (San Diego, California, USA: Academic Press) (Elsevier) 50–56.
Tsai Y. L., Hayward R. E., Langer R. C., Fidock D. A., Vinetz J. M. (2001). Disruption of Plasmodium falciparum chitinase markedly impairs parasite invasion of mosquito midgut. Infect. Immun. 69, 4048–4054. doi: 10.1128/IAI.69.6.4048-4054.2001
Tsakeng C. U. B., Tanekou T. T. M., Soffack S. F., Tirados I., Noutchih C., Njiokou F., et al. (2022). Assessing the tsetse fly microbiome composition and the potential association of some bacteria taxa with trypanosome establishment. Microorganisms 10, 1141. doi: 10.3390/microorganisms10061141
Van Den Hurk A. F., Hall-Mendelin S., Pyke A. T., Frentiu F. D., Mcelroy K., Day A., et al. (2012). Impact of Wolbachia on infection with chikungunya and yellow fever viruses in the mosquito vector Aedes aEgypti. PloS Negl. Trop. Dis. 6, e1892. doi: 10.1371/journal.pntd.0001892
Vanderveken M., O'donnell M. J. (2014). Effects of diuretic hormone 31, drosokinin, and allatostatin A on transepithelial K(+) transport and contraction frequency in the midgut and hindgut of larval Drosophila melanogaster. Arch. Insect Biochem. Physiol. 85, 76–93. doi: 10.1002/arch.21144
Vaz Junior I. D. S., Martinez R. H., Oliveira A., Heck A., Logullo C., Gonzales J. C., et al. (1996). Functional bovine immunoglobulins in Boophilus microplus hemolymph. Vet. Parasitol. 62, 155–160. doi: 10.1016/0304-4017(95)00851-9
Veenstra J. A., Agricola H. J., Sellami A. (2008). Regulatory peptides in fruit fly midgut. Cell Tissue Res. 334, 499–516. doi: 10.1007/s00441-008-0708-3
Vega-Rodriguez J., Ghosh A. K., Kanzok S. M., Dinglasan R. R., Wang S., Bongio N. J., et al. (2014). Multiple pathways for Plasmodium ookinete invasion of the mosquito midgut. Proc. Natl. Acad. Sci. U.S.A. 111, E492–E500. doi: 10.1073/pnas.1315517111
Vieira C. S., Waniek P. J., Castro D. P., Mattos D. P., Moreira O. C., Azambuja P. (2016). Impact of Trypanosoma cruzi on antimicrobial peptide gene expression and activity in the fat body and midgut of Rhodnius prolixus. Parasit Vectors 9, 119. doi: 10.1186/s13071-016-1398-4
Waltmann A., Willcox A. C., Balasubramanian S., Borrini Mayori K., Mendoza Guerrero S., Salazar Sanchez R. S., et al. (2019). Hindgut microbiota in laboratory-reared and wild Triatoma infestans. PloS Negl. Trop. Dis. 13, e0007383. doi: 10.1371/journal.pntd.0007383
Wang J., Brelsfoard C., Wu Y., Aksoy S. (2013a). Intercommunity effects on microbiome and GpSGHV density regulation in tsetse flies. J. Invertebr Pathol. 112 Suppl, S32–S39. doi: 10.1016/j.jip.2012.03.028
Wang J., Weiss B. L., Aksoy S. (2013b). Tsetse fly microbiota: form and function. Front. Cell Infect. Microbiol. 3. doi: 10.3389/fcimb.2013.00069
Wang J., Wu Y., Yang G., Aksoy S. (2009). Interactions between mutualist Wigglesworthia and tsetse peptidoglycan recognition protein (PGRP-LB) influence trypanosome transmission. Proc. Natl. Acad. Sci. U.S.A. 106, 12133–12138. doi: 10.1073/pnas.0901226106
Watnick P. I., Jugder B. E. (2020). Microbial control of intestinal homeostasis via enteroendocrine cell innate immune signaling. Trends Microbiol. 28, 141–149. doi: 10.1016/j.tim.2019.09.005
Wei N., Cao J., Zhang H., Zhou Y., Zhou J. (2021). The tick microbiota dysbiosis promote tick-borne pathogen transstadial transmission in a Babesia microti-infected mouse model. Front. Cell Infect. Microbiol. 11. doi: 10.3389/fcimb.2021.713466
Weiss B., Aksoy S. (2011). Microbiome influences on insect host vector competence. Trends Parasitol. 27, 514–522. doi: 10.1016/j.pt.2011.05.001
Weiss B. L., Maltz M., Aksoy S. (2012). Obligate symbionts activate immune system development in the tsetse fly. J. Immunol. 188, 3395–3403. doi: 10.4049/jimmunol.1103691
Weiss B. L., Wang J., Aksoy S. (2011). Tsetse immune system maturation requires the presence of obligate symbionts in larvae. PloS Biol. 9, e1000619. doi: 10.1371/journal.pbio.1000619
Weiss B. L., Wang J., Maltz M. A., Wu Y., Aksoy S. (2013). Trypanosome infection establishment in the tsetse fly gut is influenced by microbiome-regulated host immune barriers. PloS Pathog. 9, e1003318. doi: 10.1371/journal.ppat.1003318
Wilke A. B., Marrelli M. T. (2015). Paratransgenesis: a promising new strategy for mosquito vector control. Parasit Vectors 8, 342. doi: 10.1186/s13071-015-0959-2
Wu-Chuang A., Hodzic A., Mateos-Hernandez L., Estrada-Pena A., Obregon D., Cabezas-Cruz A. (2021). Current debates and advances in tick microbiome research. Curr. Res. Parasitol. Vector Borne Dis. 1, 100036. doi: 10.1016/j.crpvbd.2021.100036
Xi Z., Ramirez J. L., Dimopoulos G. (2008). The Aedes aEgypti Toll pathway controls dengue virus infection. PloS Pathog. 4, e1000098. doi: 10.1371/journal.ppat.1000098
Zhang D., Wang Y., He K., Yang Q., Gong M., Ji M., et al. (2020). Wolbachia limits pathogen infections through induction of host innate immune responses. PloS One 15, e0226736. doi: 10.1371/journal.pone.0226736
Zhong J., Jasinskas A., Barbour A. G. (2007). Antibiotic treatment of the tick vector Amblyomma americanum reduced reproductive fitness. PloS One 2, e405. doi: 10.1371/journal.pone.0000405
Keywords: disease vectors, immune system, microbiota, pathogen, arthropod control
Citation: Pavanelo DB, Piloto-Sardiñas E, Maitre A, Abuin-Denis L, Kopáček P, Cabezas-Cruz A and Fogaça AC (2023) Arthropod microbiota: shaping pathogen establishment and enabling control. Front. Arachn. Sci. 2:1297733. doi: 10.3389/frchs.2023.1297733
Received: 20 September 2023; Accepted: 20 November 2023;
Published: 04 December 2023.
Edited by:
Nighat Perveen, United Arab Emirates University, United Arab EmiratesReviewed by:
Maria Kazimirova, Slovak Academy of Sciences, SlovakiaLeonardo Diaz Nieto, Universidad Nacional de San Juan, Argentina
Copyright © 2023 Pavanelo, Piloto-Sardiñas, Maitre, Abuin-Denis, Kopáček, Cabezas-Cruz and Fogaça. This is an open-access article distributed under the terms of the Creative Commons Attribution License (CC BY). The use, distribution or reproduction in other forums is permitted, provided the original author(s) and the copyright owner(s) are credited and that the original publication in this journal is cited, in accordance with accepted academic practice. No use, distribution or reproduction is permitted which does not comply with these terms.
*Correspondence: Andrea C. Fogaça, ZGVhZm9nQHVzcC5icg==
†Present address: Daniel B. Pavanelo, Laboratório Central do Rio Grande do Sul, Centro Estadual de Vigilância em Saúde, Secretaria da Saúde, Rio Grande do Sul, Brazil
‡These authors have contributed equally to this work and share senior authorship