- 1Blue Technology Group, Cawthron Institute, Nelson, New Zealand
- 2Innovasea, Bedford, NS, Canada
- 3School of Marine Science and Ocean Engineering, University of New Hampshire, Durham, NH, United States
- 4Ecological Aquaculture International LLC, Biddeford, ME, United States
- 5Faculty of Biosciences & Aquaculture, Nord University, Bodø, Norway
- 6Kelson Marine Co., Portland, ME, United States
- 7Marine Aquaculture, Alfred Wegener Institute Helmholtz Centre for Polar and Marine Research (AWI), Bremerhaven, Germany
- 8Applied Marine Biology, University of Applied Sciences Bremerhaven, Bremerhaven, Germany
Aquaculture in exposed and/or distant ocean sites is an emerging industry and field of study that addresses the need to improve food security along with the challenges posed by expansion of urban and coastal stakeholders into nearshore and sheltered marine waters. This move necessitates innovative solutions for this industry to thrive in high-energy environments. Some innovative research has increased understanding of the physics, hydrodynamics, and structural requirements enabling the development of appropriate systems. The blue mussel (Mytilus edulis), the New Zealand green shell or green lipped mussel (Perna canaliculus), and the Pacific Oyster (Magallana gigas), are the primary targets for commercial exposed bivalve aquaculture. Researchers and industry members are actively advancing existing structures and developing new structures and methodologies for these and alternative high-value species suitable for such conditions. For macroalgae (seaweed) cultivation, such as sugar kelp (Saccharina latissimi), oar weed (Laminaria digitata), or kelp sp. (Ecklonia sp.), longline systems are commonly used, but further development is needed to withstand fully exposed environments and improve productivity and efficiency. In marine finfish aquaculture, three primary design categories for open ocean net pens are identified: flexible gravity pens, rigid megastructures, closed pens, and submersible pens. As aquaculture ventures into more demanding environments, a concerted focus on operational efficiency is imperative. This publication considers the commercial and research progress relating to the requirements of aquaculture’s expansion into exposed seas, with a particular focus on the cultivation of bivalves, macroalgae, and marine finfish cultivation technologies and structural developments.
1 Introduction
Urban expansion into agricultural land has begun to impact food supply (Güneralp et al., 2020) and portends a similar trend in aquaculture due to increased anthropogenic activity in coastal areas. Currently, aquaculture sites are concentrated in sheltered bays and regions with low exposure to wind, currents and waves (Milewski, 2001; Buck et al., 2024a). However, ocean space near population centres is increasingly occupied by other industries and stakeholders resulting in reduced potential aquaculture carrying capacity through physical, ecological, and social limitations (Inglis et al., 2000; Buck et al., 2004; Gibbs, 2009; Smaal and van Duren, 2019; Wijsman et al., 2019; Galparsoro et al., 2020; Mascorda Cabre et al., 2021). In addition, extractive aquaculture, such as production of bivalves and macroalgae, require larger production scales than fed aquaculture to be viable (Harvey et al., 2024) necessitating more water space in a diminishing area. Extending aquaculture from sheltered sites into more exposed and/or distant sites increases the energy, through larger waves and stronger water currents, impacting structural design, material choices, species selection, and commercial viability (Heasman et al., 2024; Lojek et al., 2024; Dewhurst et al., in review; Lien and Fredheim, 2001; Stevens et al., 2008; Morro et al., 2022). Advancement into exposed and/or offshore areas will require increasing robustness of equipment, improved installation protocols, reviewed health and safety protocols, and robust infrastructure maintenance protocols (Chambers et al., 2003, 2007).
Terms, such as “offshore aquaculture”, “open ocean aquaculture” or “exposed aquaculture”, are frequently used, although there is no differentiation between these terms or descriptive definitions currently. As a result, they are used entirely interchangeably and there is no clear categorisation. The definition of “exposed”, “offshore”, and “open ocean” aquaculture has been discussed in Buck et al., (2024a) as well as in Lojek et al., 2024, who discusses the various parameters, which impact farming at these sites (Figure 1). In the following, we will use the term “exposed”, as this article is focused on the extension into exposed conditions and about the adaptation of aquaculture farms to harsh weather conditions, irrespective of distance from the shore.
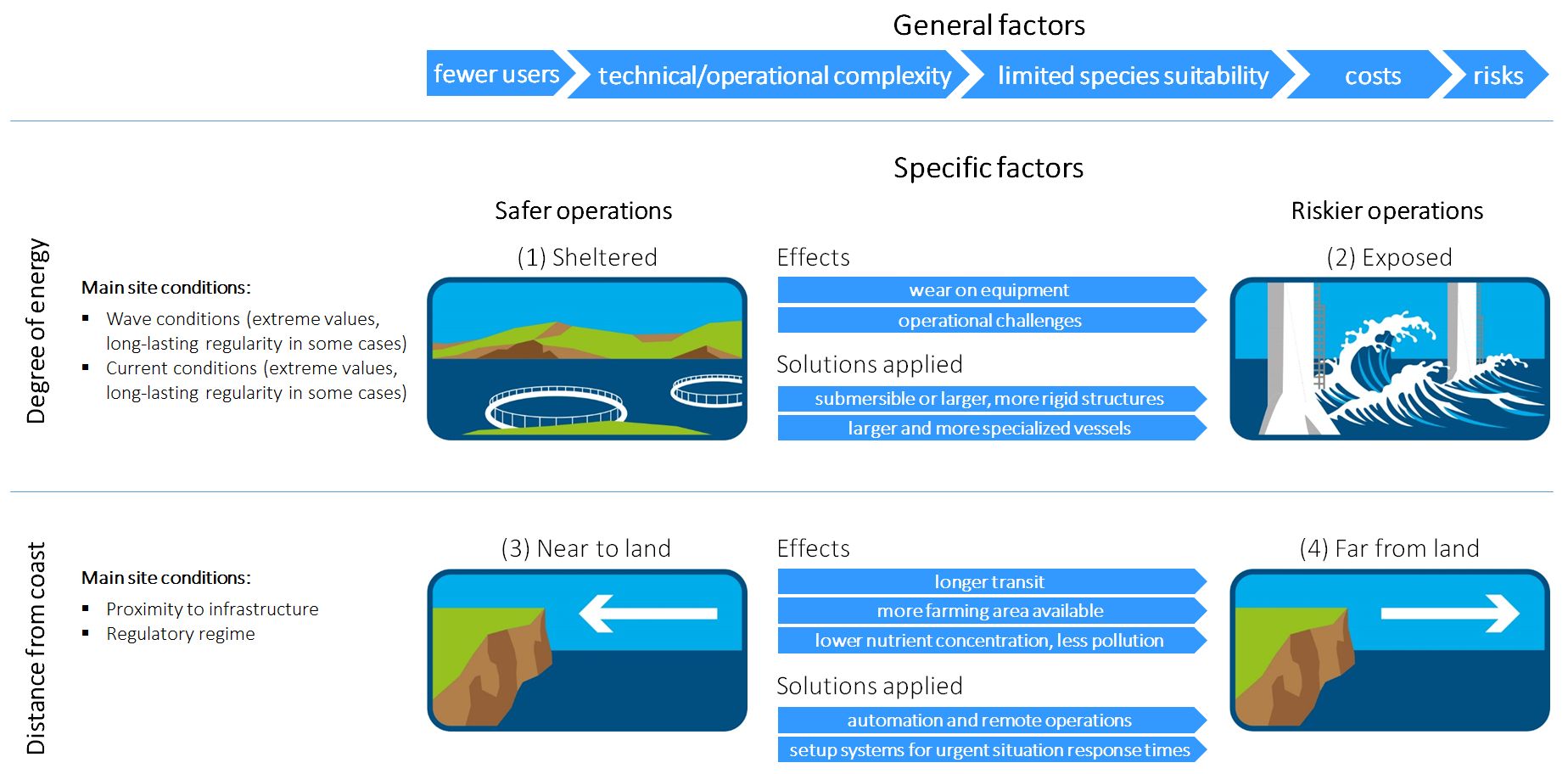
Figure 1. Comparison of “Safer operations” vs. “Riskier operations” (top) as well as “Degree of energy” vs. “Distance from coast” (left) environments along with a selected collection of general and specific descriptions of each. Modified after Buck et al. (2024b).
This publication considers the current trends of bivalve, macroalgae and marine finfish commercial systems found in sheltered waters and investigates the requirements enabling the advancement of these aquaculture species group into the exposed waters. Marine shrimp cultivation is not discussed in this article despite being an important aquaculture species, as there has been limited successful activity in exposed environments.
2 Information sources
Peer-reviewed articles from relevant journals and publications from grey literature (reports, expert opinions, other articles, etc.) were used to research data. The authors also had access to a very broad, global network of scientists working in the field of exposed aquaculture. In addition, data collection was supported by collaboration between international research projects/working groups, (ICES1 WGOOA2, ICES WGSEDA3, ARPA e4, etc.).
3 Species and technology: a comparison of the requirements between sheltered and exposed sites
Certain traditional aquaculture production methods and technologies that have been used in sheltered water for decades may be mentioned here but not discussed in detail if they are not pertinent to the advancement into an exposed farming environment. Structures that have developed more recently and that have been considered and tested in exposed areas are the focus of this manuscript.
Many technologies that withstand the extreme conditions on the high seas, i.e. exposed and/or offshore, are at an early stage of development (Kimmel et al., 2020), and often have a research project background or are still in a conceptual and drafting stage. However, there are some systems that are semi-commercial, which provide insights into requirements for expanding into more exposed regions.
Advancing from sheltered into exposed ocean environments demands a number of adaptations due to the increased energy that the structures, vessels, and species will endure. The change required is to avoid the energy forces or increase the ability of the structures, and relevant supporting infrastructure, to withstand damaging energy. To avoid energy, the structures can be partially or fully submerged (Bourque and Myrand, 2014; Idhalla et al., 2017). However, infrastructure being submerged often results in complex or more demanding methods of operation, such as methods to bring the structure close enough to the surface to be operated. Submerging aquaculture farming systems usually involves changing the buoyancy, with robust mooring also being required. It is technically complex to keep the system in the water column such that (1) it is permanently held in the desired position below the water surface, (2) it does not descend too deep and, in the worst-case scenario, sinks to the seabed, where it could collapse and result in the loss of the crop and 3) the submergence process can be reversed, usually by controlling buoyancy again which is challenging and can require extensive maintenance. Measured control is required, for example, if the system is raised too quickly, swim bladders of physoclistous fish (i.e. Cod – Gadus sp.) will inflate with decreased depth pressure, resulting in mortality of fish. In addition, the increased biomass of the species (particularly bivalves and macroalgae) and fouling will change the hydrodynamic and physical characteristics of the structure, making design challenging.
Taking a structure that has been successful in the inshore and sheltered regions, enlarging ropes and floats, and placing it into an exposed site (i.e. evolving the gear) can be a successful strategy in some instances but has its limitations. We refer to this type of modification of existing system design, which only undergoes a slight change in size and weight, as an evolutionary adaptation. The structure may survive but the organisms being cultured will not be able to endure the response of the structure to the extra energy found at the exposed site resulting in stressed, damaged, detached crop, or mortality. A revolutionary approach requires unique solutions and new strategies (such as efficient submerging equipment) or innovative, novel equipment, probably with materials not commonly used before under marine environmental conditions. Linear systems and marine finfish pens lend themselves well to modification or revolutionary (as opposed to evolutionary) adaptation to higher energy while maintaining their production efficiency.
3.1 Bivalves
Globally, there are a number of molluscan bivalve species including scallops, clams and oyster species cultivated in sheltered environments (Wijsman et al., 2019) and nine prominent cultivated species of mussels (Kamermans and Capelle, 2019). Although Asia produces the majority of the world’s bivalves through aquaculture (Wijsman et al., 2019), there do not appear to be sites with published information in truly exposed areas in Asia. Currently, commercial exposed ocean bivalve aquaculture operations worldwide appear to primarily rely on three species. In the Northern Hemisphere, Mytilus edulis, commonly known as the blue mussel, as well as Mytilus galloprovincialis, known as Mediterranean mussel, are cultivated, with sites situated in the USA, England (Gagnon, 2024), and Germany (Heasman et al., 2024; Buck et al., 2024b). Conversely, in the Southern Hemisphere, culture of Perna canaliculus, or the New Zealand green shell mussel, is the primary focus (Newell et al., 2021) although Chile is an important producer of Chilian mussels (Mytilus chilensis) in semi exposed sites (Gonzalez-Poblete et al., 2018). Mussels are well suited to cultivation in exposed situations as their primary habitat is generally high energy, and they are capable of reattaching or reinforcing their attachment to artificial substrates. Mussels, however, yield a low profit margin and are therefore required to be produced in high volumes with high efficiency.
Mussels (e.g., Perna sp. Mytilus sp. etc), oysters (e.g., Crassostrea sp. Magallana sp.), clams (e.g., Quahog, Mercenaria mercenaria, Manila clam, Venerupis sp., little neck clam, Protothaca sp. etc.), and scallops (e.g. Aequipecten sp., Argopecten sp., Chlamys sp., Pecten sp., etc.) are good candidates for exposed ocean production, however, there is limited exposed ocean cultivation activity with all four of these shellfish. Therefore, discussion will be limited to inshore and experimental exposed ocean systems.
3.1.1 Trends of current commercial bivalve aquaculture in sheltered systems
The most productive systems for the cultivation of bivalves in sheltered areas range from bouchots, rafts, seabed cultivation and linear systems, such as the New Zealand long line (Kamermans and Capelle, 2019; Strand et al., 2022). Some of these systems (e.g. bouchots) are traditional, going back to the 13th century and continue today (primarily in France), with improving seeding and harvesting technology. On-bottom culture is also traditional but with variations, such as warehousing (grow-out and storing blue mussels on the seabed) until market size is reached, as is conducted in the Netherlands and Germany. Spat collection can be done in the water column via a single-backbone-longline or -longtube systems and the following conditioning (fattening) of adults through on-bottom cultivation, as is done in Germany. Bottom culture represents approximately 15% of overall production, with the remainder being produced on suspended structures (Mckindsey et al., 2011). Mussel rafts are primarily used for production in Spain (Wijsman et al., 2019). Bouchots, on-bottom cultivation (except for the German variant), and rafts have not varied much in recent years, with most of the modifications relating to carrying capacity, seed production/collection, and efficient use.
The use of linear systems, particularly in bivalves, appears to be directly related to the efficiencies these systems offer associated with space utilisation, and ease of use for seeding and harvesting (Goseberg et al., 2017; Newell et al., 2021) as can be seen with linear systems such as the longline, Smart Farm5 (Lien and Fredheim, 2001), and Flipfarm6.
3.1.1.1 The New Zealand longline system – green-lipped mussels
In comparison to the European longline version, the sheltered New Zealand mussel longline systems have a double backbone (header rope) with floats (approximately 300 litres in size) spaced evenly along the length of the backbone (Figure 2A) (Newell et al., 2021). The typical backbone may be synthetic rope (polyethylene/polypropylene), 29mm to 32mm in diameter and 100m in length (range 70 to 180m). Currently there are 3,000 to 4,000m of continuous dropper lines (cultivation rope) and produce up to 32,000kg of mussels per cycle (between 4 and 9 kg/m). Water depth ranges from 15 to 35m and mooring normally has a 1:3 depth to mooring length ratio. Floatation is added as required during the growth cycle to accommodate increased crop and biofouling biomass. The structures are generally run parallel with the shoreline to maximise space usage (which is not always conducive to the cultivated species) and are normally spaced from 10 to 15 m apart (Goseberg et al., 2017; Newell et al., 2021). Most New Zealand longline systems utilise both wild caught spat and hatchery spat. A single company has a large hatchery which subsidises their wild caught spat. Other companies are following this trend with new hatcheries in construction.
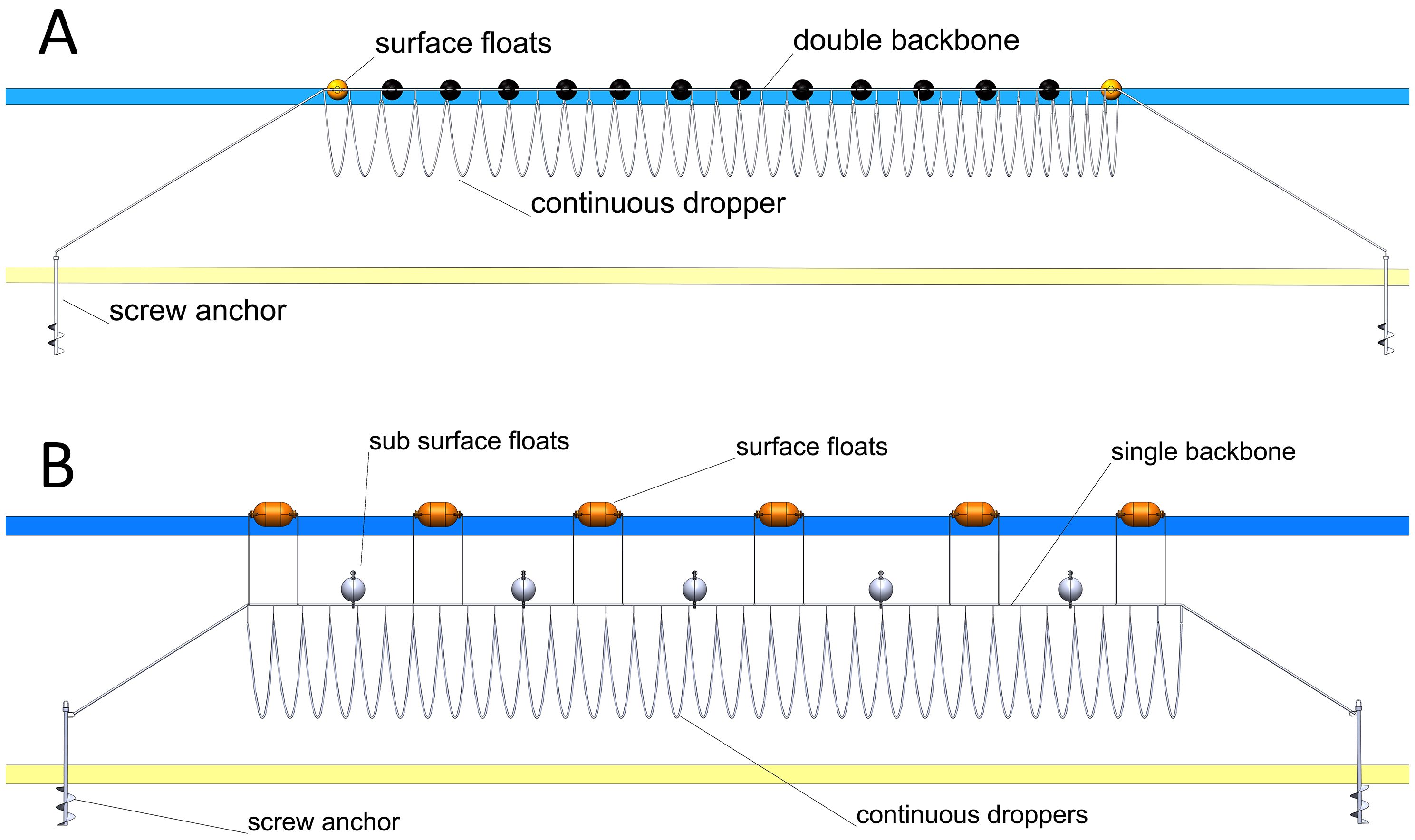
Figure 2. (A) Schematic of a general design of a mussel longline system in sheltered areas. The backbone has dropper lines hanging down perpendicularly being held at the water surface by buoys. This is normally configured as a “double backbone” which equates to two header ropes running parallel with floats in between. (B) Schematic of a general design of a mussel longline system for exposed sites. The backbone with the dropper lines is submerged and flotation at the surface is reduced. This is normally configured in a single backbone which is a single header rope from which floats are attached.
3.1.1.2 The Smart Farm™ - blue mussels
The Smart Farm (Figure 3A) has made two primary advancements from a standard mussel cultivation system with many individual surface floats: the utilisation of a continuous inflatable float in the form of a High Density Polyethylene (HDPE)-tube, about 310mm in diameter and usually 100m long, from which a culture net is suspended with weights at its lowest edge. The structure parameters and floatation vary according to the energy environment. The second is the husbandry and harvesting is carried out in the water with bespoke brushing equipment which is mounted on the vessel. All activity is machine driven improving staff safety. Vessel size can vary according to the volume of the operation and the environment. At this time, Smart Farm utilises only wild caught spat.
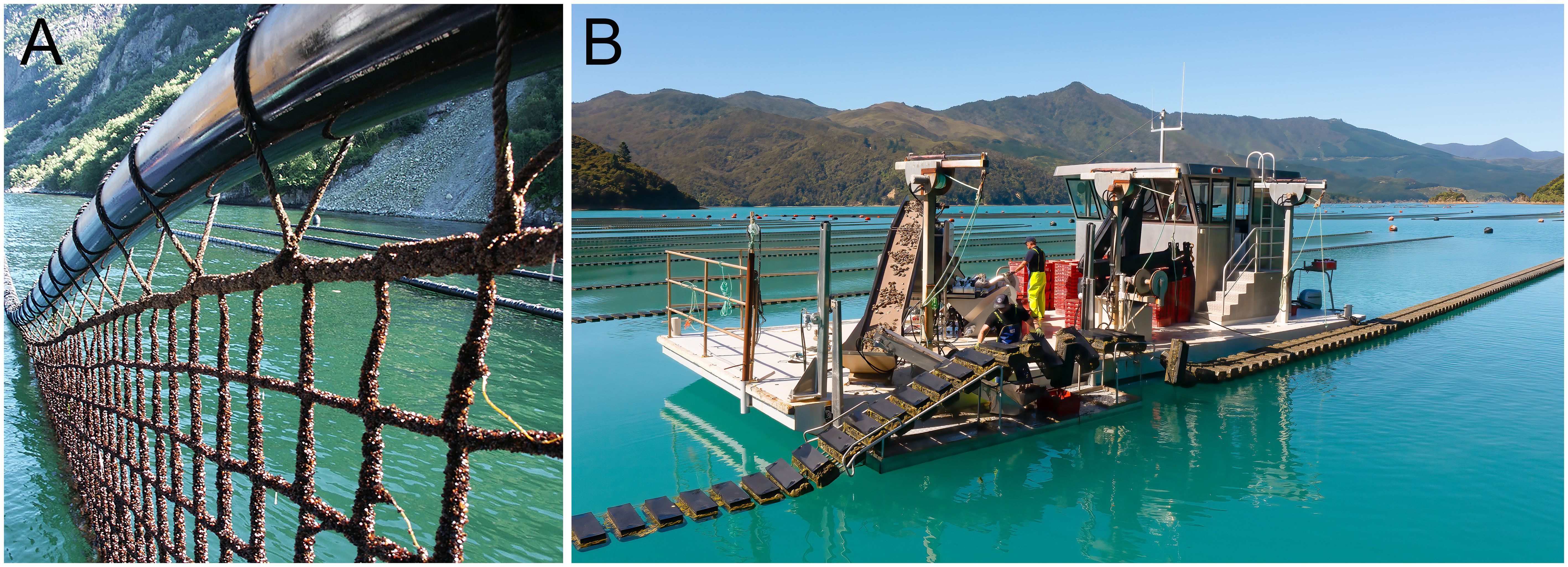
Figure 3. (A) The Smart Farm long tube with suspended culture net (copyright, Smartfarm). (B) The Flipfarm (copyright Flipfarm) oyster system showing the linear configuration of the baskets being rotated (flipped) on the barge.
3.1.1.3 Flipfarm™ (New Zealand - Pacific Oysters - Magallana gigas)
The Flipfarm (Figure 3B) is a semi-autonomous linear system for growing oysters in sheltered waters. It is a floating linear system with oyster baskets at the surface. The baskets are spaced evenly and perpendicular to the central spar around which the basket can rotate. Each basket provides its own flotation. Periodically a small vessel (8 to 15m) with bespoke equipment runs alongside, and parallel, to the baskets flipping them over and exposing fouling to the air and redistributing the oysters within the basket. After a short period of exposure, the baskets can be flipped back to submerge the oysters. Baskets can also be brought into a servicing zone on the vessel in a semi-autonomous, continuous basis at which time each basket can be stocked or harvested. Staff effort is reduced, and safety increased in handling the oysters with this system. The Flipfarm is reliant on hatchery produced oyster spat as it requires unattached individuals.
3.1.1.4 Vessels
Current vessels utilised for inshore green shell and blue mussel longlines are 15 to 45m long, up to 6m in width and generally flat bottomed. The flat bottom reduces the influence of water currents on the hull, but it makes handling more difficult. It is more likely to turn to the wind due to the windage of the surface structure and no or limited keel. The smaller vessels are used for sampling crop for harvestability and minor maintenance. A minimum of two individuals operate the vessel for efficiency and safety reasons. The larger vessels can accommodate 6 staff and undertake any maintenance, seeding or harvesting required by the operation. The deck is configured to optimise processing space and storage space for spat/seed/product. Vessels used for on-bottom culture are between 34 and 46m long and can be up to 10m wide. Smartfarm support vessels, compared to the other vessels, have a very large loading capacity to be able to transport mussels between cultivation areas or from the nets of the Smartfarms. A self-stabilising system with movable steel piles is used in the case of the Smartfarm when installation work is carried out on the tube, or support piles are driven into the ground on both sides to attach the tube. If the mussels are returned to the cultivation areas for further grow-out to market-size, an internal flushing system is used. It flushes the mussels out of the vessel storage holds, allowing them to fall to the seabed and anchor themselves.
3.1.2 Trends enabling advancement of molluscan bivalves into exposed sites of current commercial aquaculture systems
Bivalve linear systems lend themselves well to modification or revolutionary (as opposed to evolutionary) adaptations, and they can be adapted to higher energy environments while maintaining their production efficiency. To successfully extend a linear system into exposed conditions it has to avoid the higher wave energy found at the exposed site by either reducing its surface buoyancy or be fully submerged while still supporting the crop. This is achieved through reducing buoyancy (e.g. float size and shape), transferring energy to the main cultivation structure i.e. reducing the number of floats on the surface, reducing the number of header ropes, or completely submerging the structure (Figure 2B). The most effective transition of a linear system that is in commercial use in exposed regions can be found in New Zealand, Europe, and the USA (Newell et al., 2021). The most successful exposed aquaculture sites are in deeper water reducing the influence of the interaction of large waves with the seabed, where energy increases inversely-proportionately to depth (Heasman et al., 2024).
Moorings are varied and generally have to offer greater purchase or mass to maintain their position in higher energy situations. Drag embedment anchors (e.g. Danforth anchors) can be used, however there are generally more than one per mooring line. Concrete blocks (both flat bottomed or shaped bottomed for suction) are used, however they are generally required to be very large and heavy which becomes very cumbersome and expensive to handle and deploy. In sandy or muddy sea beds, heavy but flat shaped anchor stones make it much safer as a mooring as the increased surface area at the seabed “sucks” itself into the sediment. Screw anchors (also known as helix anchors) are becoming more prominent (Newell et al., 2021) as they do not drag, can be positioned accurately, and many can be held on an installation vessel deck at one time and installed in one day. Their use and reliability are dependent on the substrate into which they are being drilled. They can be shaftless or have a shaft with attachment points at the top or on the side of the shaft. Attachment from the screw anchor to the structure may have a large link chain and then a rope or just a rope. Though, in comparison to the use of small anchor blocks, the deployment of screw anchors can be costly and depending on the mooring depth. However, as the anchor block increases in size the cost of the block and deployment may exceed that of screw anchors. Should the sites require decommissioning and complete recovery of moorings, then drag anchors and smaller concrete blocks will be the least problematic. Larger concrete blocks, or shaped blocks (which increase suction to the seabed) will be difficult to dislodge. Some blocks have eyes mounted at the edge of the mooring which can be utilised to break the suction with the seabed, but these can be difficult to access in deep water with limited diver access. Screw anchors, particularly the shaftless variety which are driven several meters deep into the substrate, will be difficult if not impossible to recover as they are metal they will erode over time.
3.1.3 Examples of exposed bivalve aquaculture farms
In New Zealand, the most exposed farms are in 30 to 70 m of water with a 50-year significant wave height anticipated to be 7.6m and a water current speed maxima of 0.6 m/sec. The main species grown is Perna canaliculus. The structures consists of up to 200m of growing header rope and the mooring legs (from header rope to mooring) are 3 times the depth. The moorings consist of screw anchors. In these systems the header rope has been reduced from 2 lines to 1 and most of the floatation is submerged to 9m (Figure 2B). More flotation is added as the crop grows. The header rope has enough slack in it to allow it to be brought to the surface, in an apex, by vessels. The equipment has been upgraded for strength with the header ropes being increased to 44mm with diameter and different floats being tested (shape, volume, attachment methods) to assist with the durability and maintenance of the structure. Each header rope has approximately 4000m of continuous longline on which the mussels are grown.
Service vessels are up to 40m long and can operate in swells of up to 1.5m which in normal years is approximately 72% of the year.
3.1.3.1 Submersible Long Tubes
Longtube systems, such as the Smart Farm, are usually installed at the water surface, but due to the HDPE-tube floats they can also be modified to be submerged (Figure 3A). It has a net on which to culture mussels (Mytilus edulis) as opposed to the continuous longline system. The nets have an advantage over dropper lines in that the mesh transfers wave energy across its surface and the nets act as a single unit. Dropper lines respond independently to energy transfer and will interact with each other resulting in the loss of crop and greater maintenance. A further development of the long tube is that the hollow buoyancy tube can be fitted internally with additional inflatable smaller tubes (Tayler et al., 2022). These smaller tubes can then be filled with air, depending on the position of the overall structure in the water column or on the water surface. In this further development, one end cap of the long tube is open so that water can flow into the spaces between the tubule bundles. The cap on the other tube-end is equipped with valves which supply the inner smaller tubes with inflating air when buoyancy is needed. This means that only the volume of the inflatable smaller tubes needs to be calculated when assessing the sinking or floating, which makes operation much easier. One smaller tube within the main tube is always filled with air (3 bar). This guarantees a minimum buoyancy so that the system will not sink to the seabed if it is lowered without appropriate inflation.
3.1.3.2 Shellfish tower
The shellfish tower (Heasman et al., 2021) (Figure 4) is a unique structure designed for complete submergence and the production of single seed bivalves (such as pacific oysters, Magallana gigas, and scallops, Pecten novaezelandiae). This structure shows all the attributes required for the extension of a structure into exposed waters. The shellfish tower consists of a stainless-steel hexagonal frame equipped with six hexagonal subunits which rotate freely (Figure 4). In the centre of the main frame, a mooring rope runs through a steel tube which is enclosed by the buoyancy device providing the shellfish tower with positive lift. It is fully submerged, avoiding surface wave energy. It has a single mooring which can be referred to as a tension leg, which allows the structure to be drawn off the vertical in strong currents where it can shed energy (Landmann et al., 2019). It is fully floated during initial deployment to allow for crop growth and fouling during the grow-out stage, reducing operational maintenance requirements. Further developments of this structure for the EEZ in the German North Sea can lead to the expansion of cultured species. This refers not only to similar candidates, such as the European oyster (Ostrea edulis), but also macroalgae, such as sea lettuce (Ulva sp.) and sugar kelp (Saccharina latissima). The modified shellfish/seaweed tower, which is deployed in 6-7m depth in the southern North Sea is a bit smaller in width (Figures 5A–E) compared to the New Zealand type to be handled easier by the available operation vessels. Additionally, some technical modifications are included concerning the cultivated species and the release system being fixed at the mooring rope below the shellfish tower (see 4.3.1).
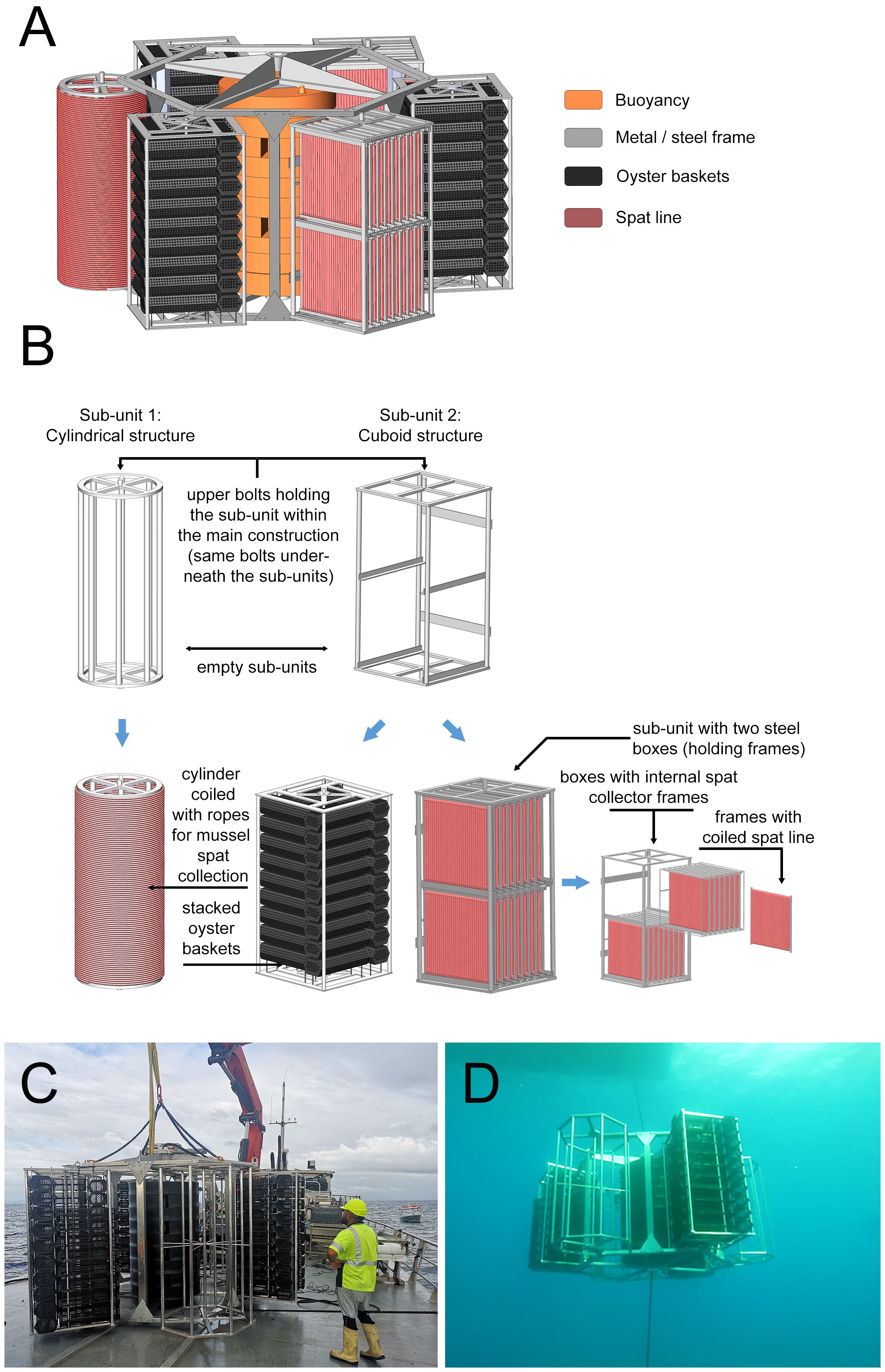
Figure 4. Illustrations of the shellfish tower design for the southern North Sea (modified after Heasman et al., 2021). (A) The tower showing variations of the subunits attached to it. (B) Variations of the subunit frames and their culture baskets/media. (C) The shellfish tower being deployed. (D) The shellfish tower in position 10m below the surface.
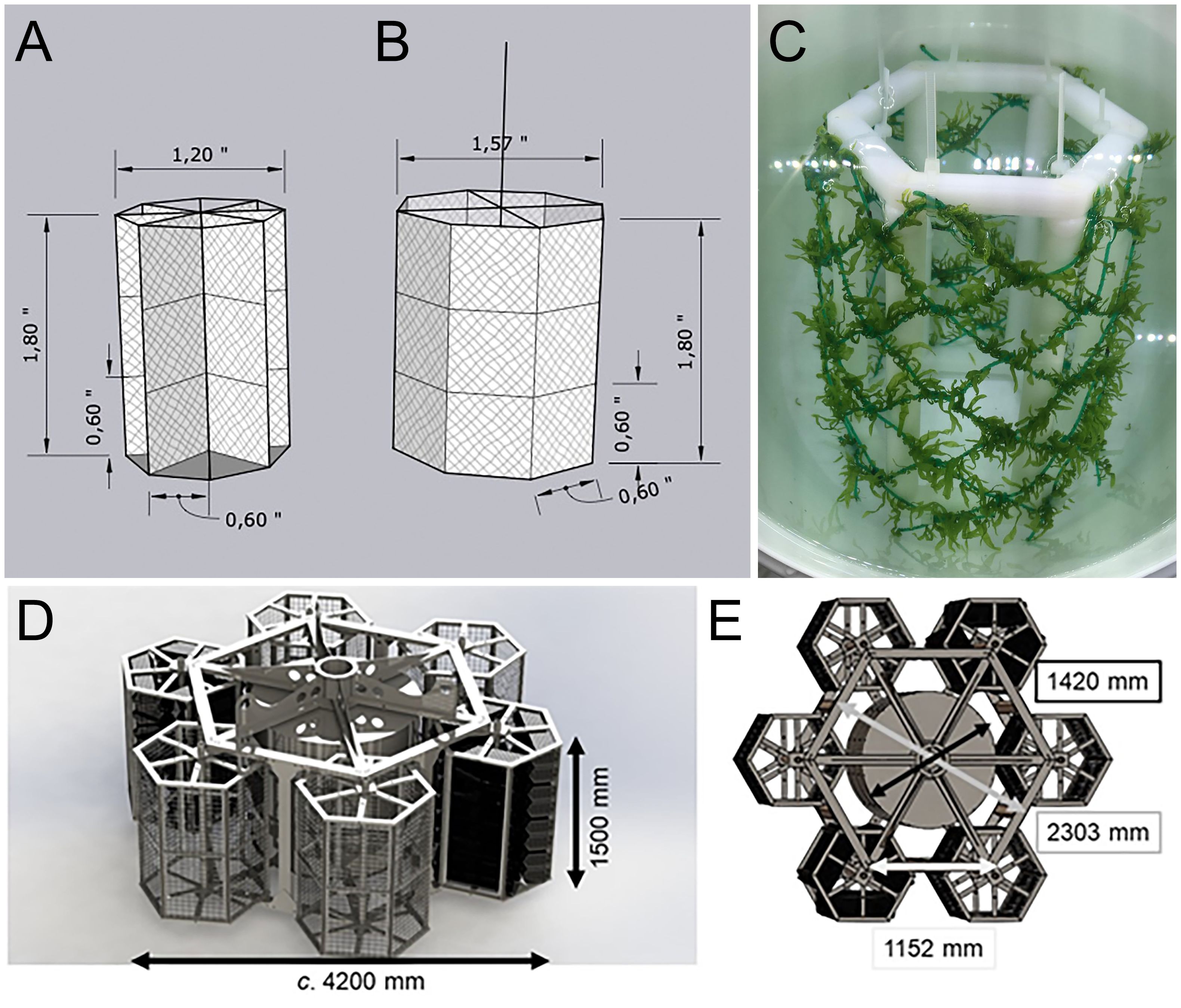
Figure 5. Modified “Seaweed-Tower”. (A) The star design which increases the surface area for growing seaweed. (B) The cylinder system providing a plain surface area for growing seaweed. (C) The chequered design for growing seaweed as a Polyoxymethylene (POM) lab model. (D, E) Dimensions of the Seaweed-Tower from side and top view.
3.2 Macroalgae (seaweed)
A number of macroalgae species are cultivated worldwide, which come from the three groups of green (Chlorophyta), red (Rhodophyta) and brown algae (Phaeophyta) (FAO, 2022). As is the case with other aquaculture species, the majority of macroalgae are cultivated in Asia, with the species such as Laminaria japonica, Kappaphycus spp., Porphyra spp., Undaria pinnatifida and Eucheuma spp. being particularly well represented. In the following, we will only focus on Sugar kelp (Saccharina latissimi) and Wakame (Undaria pinnatifida), as these macroalgae have been tested for cultivation in high-energy environments. The cultivation of macroalgae in the sea has a number of overlaps with mussel farming (see 3.1), as they are also cultivated on substrates, such as horizontal ropes. Production locations have historically been limited to sheltered bays and/or low-energy waters. One of the reasons for this is that most seaweed species cannot be submerged into deeper waters due to the light prerequisites required for photosynthesis. Submerging seaweed deeper in the water column affects the colours of light, the light intensity, and can reduce growth through shading (e.g. sediment load, strong attenuation) (Maltsev et al., 2021). There are exceptions for some macroalgae species, such as Macrocystis spp. that can be submerged down to 80 m below the surface (Tullberg et al., 2022). Nutrients can not only decrease with distance from the coast to the open sea, they can also be variable in the water column. There are regions that are nutrient-rich due to natural upwelling, such as the Humboldt Current (Peru), the Benguela Current (Namibia), the Canary Current off the coast of Galicia (Spain), etc (Kämpf and Chapman, 2016), but there are also areas, where the upper layers are nutrient-poor through limitation of a particular nutrient/element (Moore et al., 2013) or due to the blocked transport by a temperature-induced density gradient, with warm light waters residing on top of heavier cold waters (Ortiz Cortes, 2022). In contrast, nutrient availability in the lower layers of this water column may be higher than at the surface. In such areas, the idea of artificial upwelling as nature-based solution is often mentioned in order to bring nutrients to the water surface and thus bridge the shortage of nutrients and enable seaweed cultivation (Fan et al., 2019), e.g. via mounted or floating pumps (Fan et al., 2020) and/or offshore wind farms (Viúdez et al., 2016). A considerable amount of upwelled water is required (World Ocean Review, 20247) to be of any use. Water also has to be upwelled efficiently in terms of energy usage and maintenance, e.g. solar and airlift systems (Zhang et al., 2024). On reaching the surface however, the colder upwelled water can also sink down from the surface as it is denser (Kemper et al., 2022), and therefore be effectively out of reach of the seaweed. Alternatively, nutrients may be accessed through depth cycling, i.e. dropping seaweed into deeper nutrient rich waters periodically which results in morphological and biochemical variations to seaweed grown in the comparatively nutrient poorer surface waters (Navarrete et al., 2021). It is suggested that these variations may be targeted to improve the economic viability of seaweed culture in the future.
In contrast, there is concern that upwelled water may reduce surface temperatures and effect the carbon balance (Jürchott et al., 2023) which will influence the surface ecology, particularly with regard to carbon uptake, phytoplankton and zooplankton and the associated food pyramid. Therefore, uncertainty regarding the feasibility, effectiveness and potential risks and side effects associated with artificial upwelling still exists (Kemper et al., 2022). There are a number of research projects with Atlantic salmon (Salmo salar) (Rivas et al., 2021), Pacific oysters (Mizuta et al., 2014), blue mussels (Handå et al., 2014), to name a few, that have successfully utilised the principle of artificial upwelling. Although there have been studies conducted on seaweed farming and artificial upwelling (Fan et al., 2020), further research is required to clarify and quantify the issues and benefits to seaweed producers.
Although bio-stimulants, high value foods, and fermented feeds are increasing the value of macroalgae, generally macroalgae only yield a small profit margin and must therefore be cultivated in large-scale farms and with carefully considered efficiency. In Asia, beta components from macroalgae are part of people’s daily diet creating demand and value for the unprocessed products. Elsewhere, it is more often about the components, such as phycocolloids (agar, alginates, carrageenan) as well as bioactive substances and other ingredients (Holdt and Kraan, 2011) which hold greater value than biomass.
3.2.1 Trends of current macroalgae commercial systems
For both sugar kelp and Wakame, cultivation begins in the laboratory, where young sporophytes are grown on ropes according to the reproductive cycle via zoospores. Once the macroalgae is planted at sea, kelp thalli can grow up to 2-5 m long or even longer and will be harvested between 6-8 months after deployment (Pereira and Yarish, 2008; Redmond et al., 2014). Following Buck and Buchholz (2004) and Buck and Langan (2017), macroalgae cultivation techniques in sheltered water bodies usually have a linear structure and can be deployed in the form of longlines, ladders, and horizontal grid systems (Figures 6A, B, D). These systems are the classic forms of cultivation used worldwide and lead to a global production of up to 140 million tonnes per year (90% in Asia) (FAO, 2022). Finer details of the construction and configuration are shown in Figures 6C, E–G.
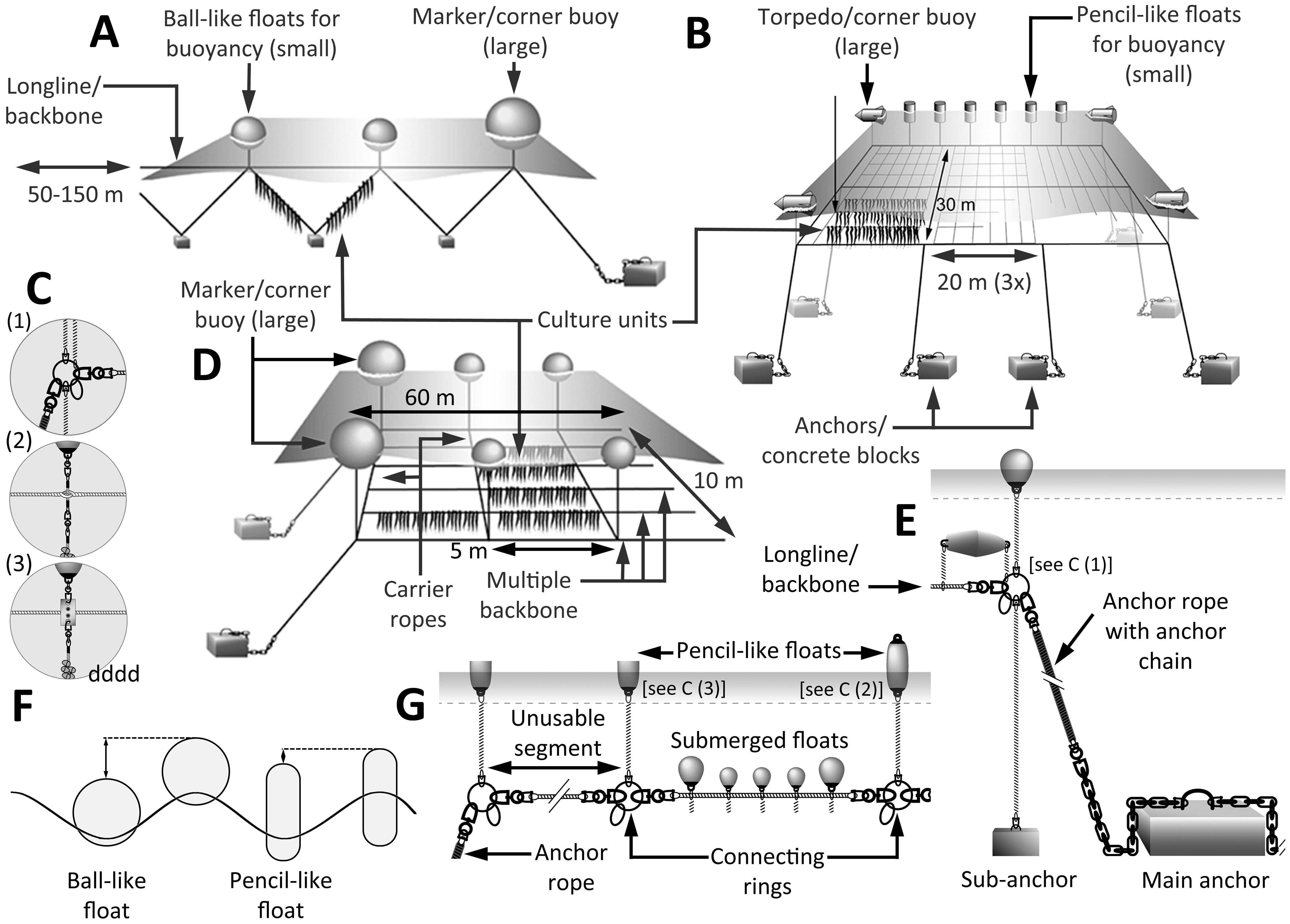
Figure 6. System design and concept of macroalgae cultivation devices. (A) Longline (backbone) system with floating buoyancy and seaweed culture unit hanging perpendicular in the water column. (B) large grid design with floating buoyancy and rectangular culture units. (C) Connection devices with (C1) rings as coupling centre piece; (C2) connection of floats to the backbone within the strands of the rope or (C3) by using metal tiles. (D) Ladder construction with culture units attached to the multiple backbone of the system. (E) Unit at one end of a backbone with different anchors, holding devices (chains, ropes), and floatation. (F) Different buoy shapes and dipping depths when riding the swell. (G) Backbone with floating and submerged flotation as well as the “unusable segment”. Modified after Buck and Langan (2017) and Buck (2007).
3.2.2 Trends enabling advancement of macroalgae into exposed sites
Most cultivation systems for seaweed deployed in exposed areas between 1985-2015 were comprised of ropes (horizontal, vertical) and in some cases cages or rope nets were installed (Fernand et al., 2017). In their comprehensive review, Tullberg et al. (2022) indicated that in order to preserve the basic spatial layout in exposed regions, cultivation systems have to be fixed by multi-point moorings and buoys or the design has to include high internal resistance to cope with compressive loads, resulting in heavier and more expensive structures. The authors recognised that trials of testing cultivation systems revealed the most promising systems for offshore seaweed cultivation seem to be linear systems with macroalgae growing on the sea bottom or in the water column. Though, the authors emphasis that circular systems could catch up with the linear systems when the automation of cultivation processes (i.e. harvesting) are further developed.
Research into macroalgae aquaculture at offshore sites in Germany began in 1992 with an initial prototype of a circular farm design (Lüning and Buchholz, 1996). This system design was improved over the years and ended in the so-called “offshore ring system” for the cultivation of macroalgae (Figures 7A–D) (Buck and Buchholz, 2004). Here, the best results were achieved with the brown macroalgae S. latissima, as it quickly adapts to the harsh weather conditions in exposed marine commercial fishing and aquaculture areas and thus there was no loss due to breakage of the stipes or detachment of the holdfasts (Buck and Buchholz, 2005). A key aspect of why this ring system withstood the harsh conditions in exposed waters well was the anchoring, which consisted of a single-point mooring (anchor stone or screw anchor) with the ring attached to the tether upwards and downwards via a crow’s foot lashing, a knot used to spread the force of the ring over a wider area, preventing damage to the culture unit. This allowed the ring to turn with the current in all directions and be less affected by the waves due to the submerged mode of about 3 metres.
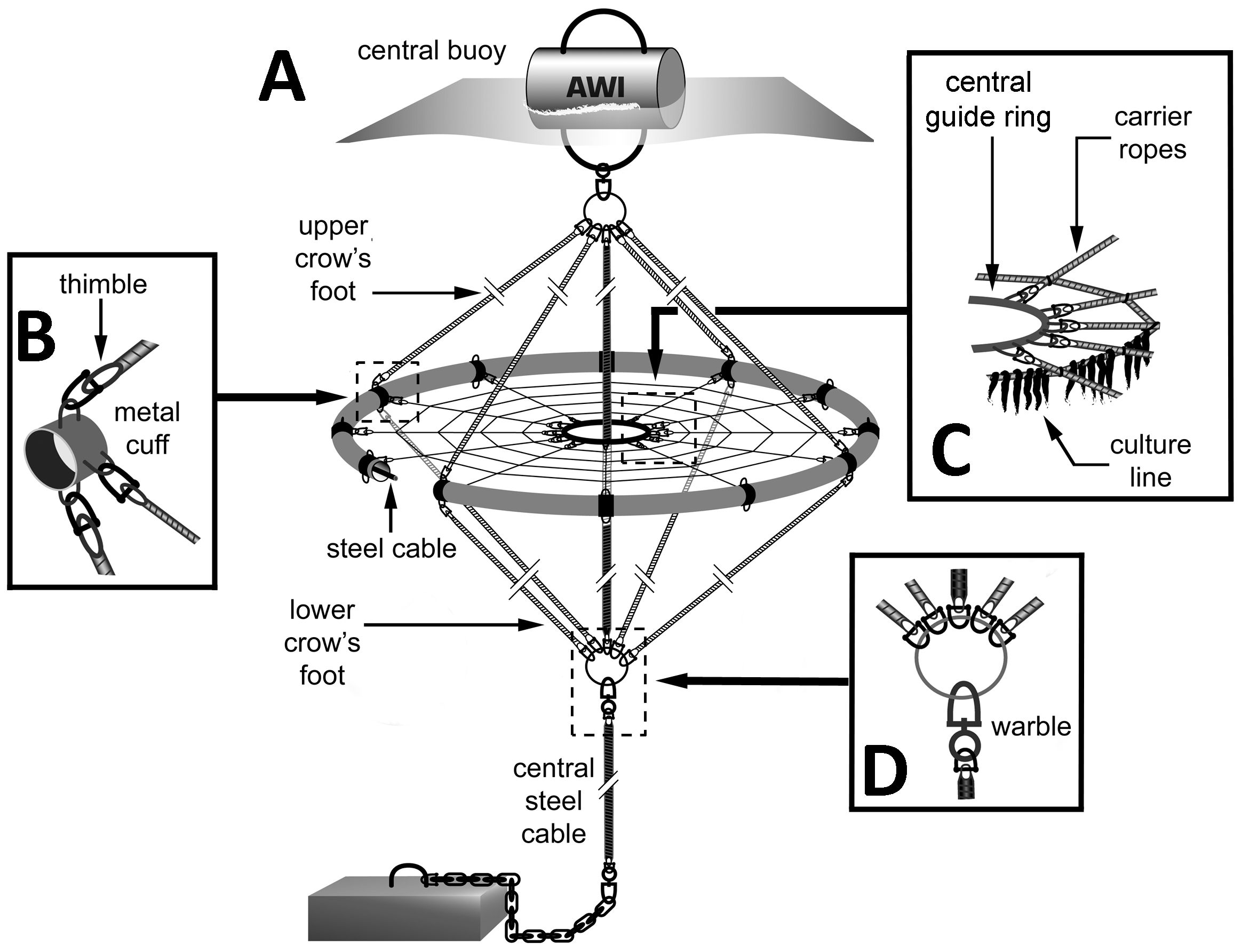
Figure 7. Offshore-ring device for the cultivation of extractive species. (A) Ring construction for the culture of sugar kelp (Saccharina latissima) at exposed locations. (B) Metal cuffs, to which the upper and lower crow’s feet, the ring tube, and the carrier ropes are attached to provide better attachment and strength during rough conditions. (C) Central guide ring with attached carrier ropes and culture lines to avoid entanglement. (D) Transition between the central steel cable of the mooring and that of the lower crow’s foot. (Modified after Buck and Grote, 2018).
Whale entanglements in marine gear are a major concern along the North Atlantic US coast. Although there are no documented whale entanglements with aquaculture equipment in this area, incidences involving fishing gear such as gillnet or pot lines resulted in rope wrapping and knotting around whale fins, flukes, or jaws. To help resolve this issue, a submerged, stiff, composite kelp farm structure was developed and deployed at an exposed site near Saco Bay, Maine (Figures 8–10) (Moscicki et al., 2024; Chambers et al., 2023). This project, called “A Validated Finite Element Modeling Tool for Hydrodynamic Loading and Structural Analysis of Ocean Deployed Macroalgae Farms” was funded by the US Department of Energy ARPA-E’s MARINER program to develop technologies that enable large scale macroalgae cultivation for the purpose of generating material for sustainable human food, animal feed, and biofuel. The Aqua vitae program8 has designed a system that is an air pressure controlled longline system (Strand et al., 2022), however there are controlling intellectual property considerations that limit the transfer of specifics.
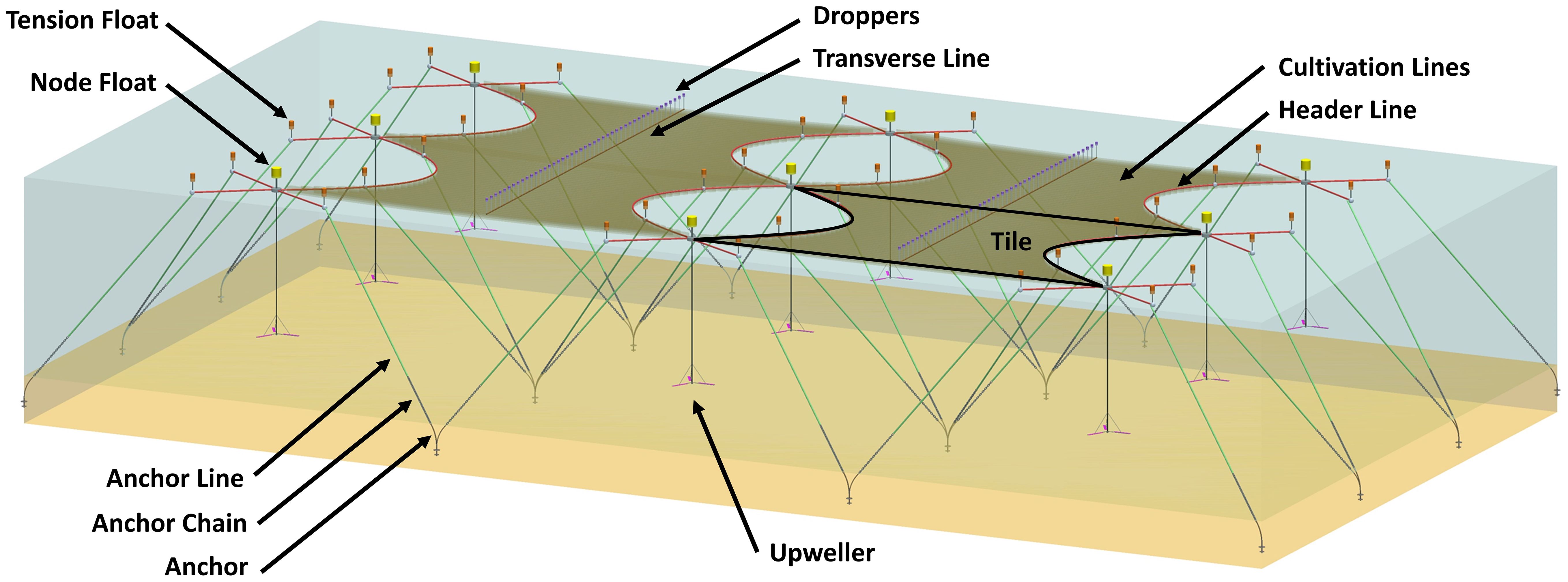
Figure 8. Kelp cultivation structure with four modular “tiles”. Each tile represents a semi-independent array of kelp cultivation lines, deemed “tiles”, each with four associated moorings extending outwards from the corners. Kelp cultivation lines extended parallel along the long side of the “tile”, between header lines on either end.
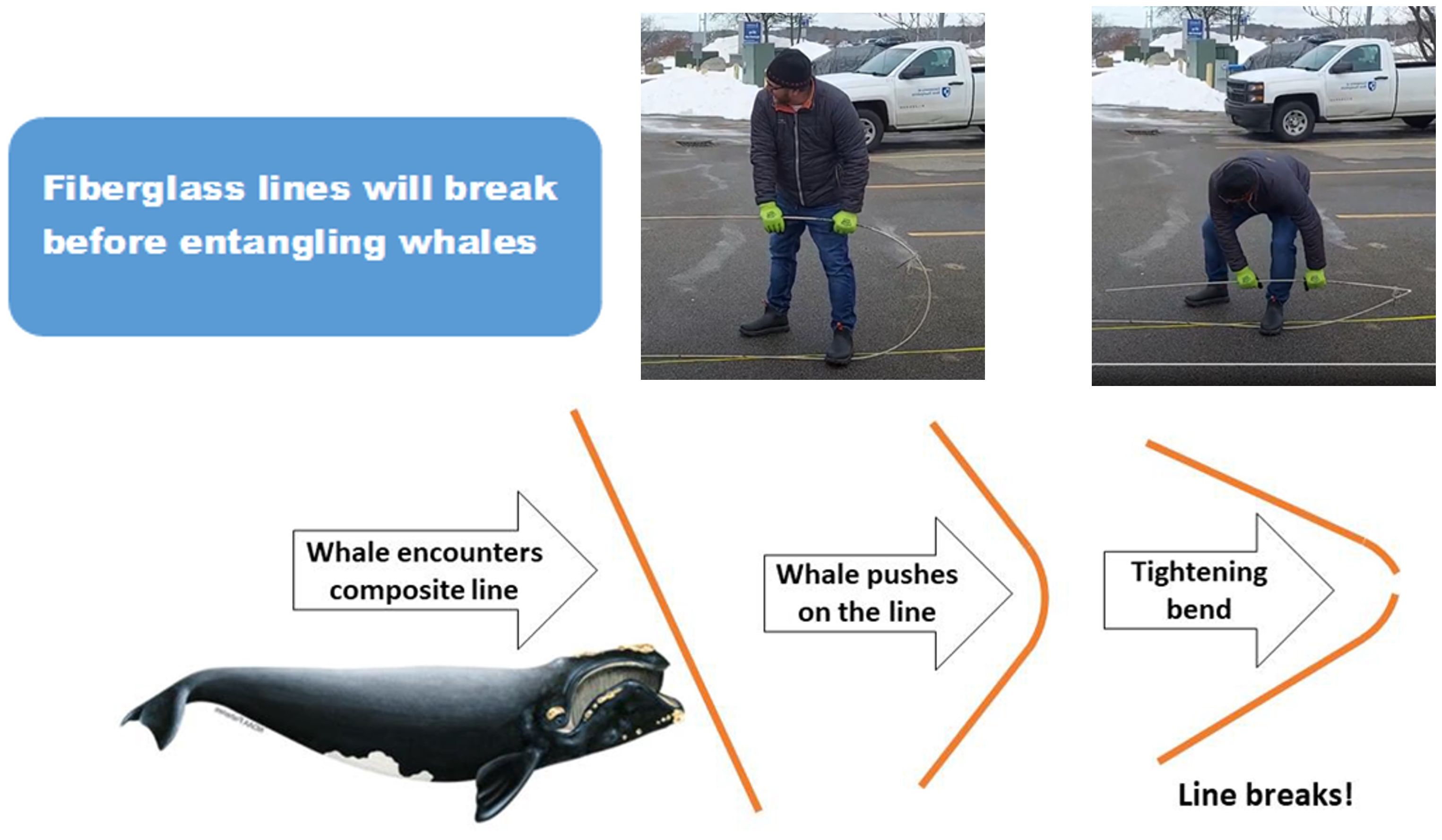
Figure 9. An illustration of a 12 mm diameter fiberglass rod bending and breaking, by a human. The same would happen, in theory, if a whale hit the rod, bending, breaking and swimming on, resulting in loose lines to entangle marine mammals.
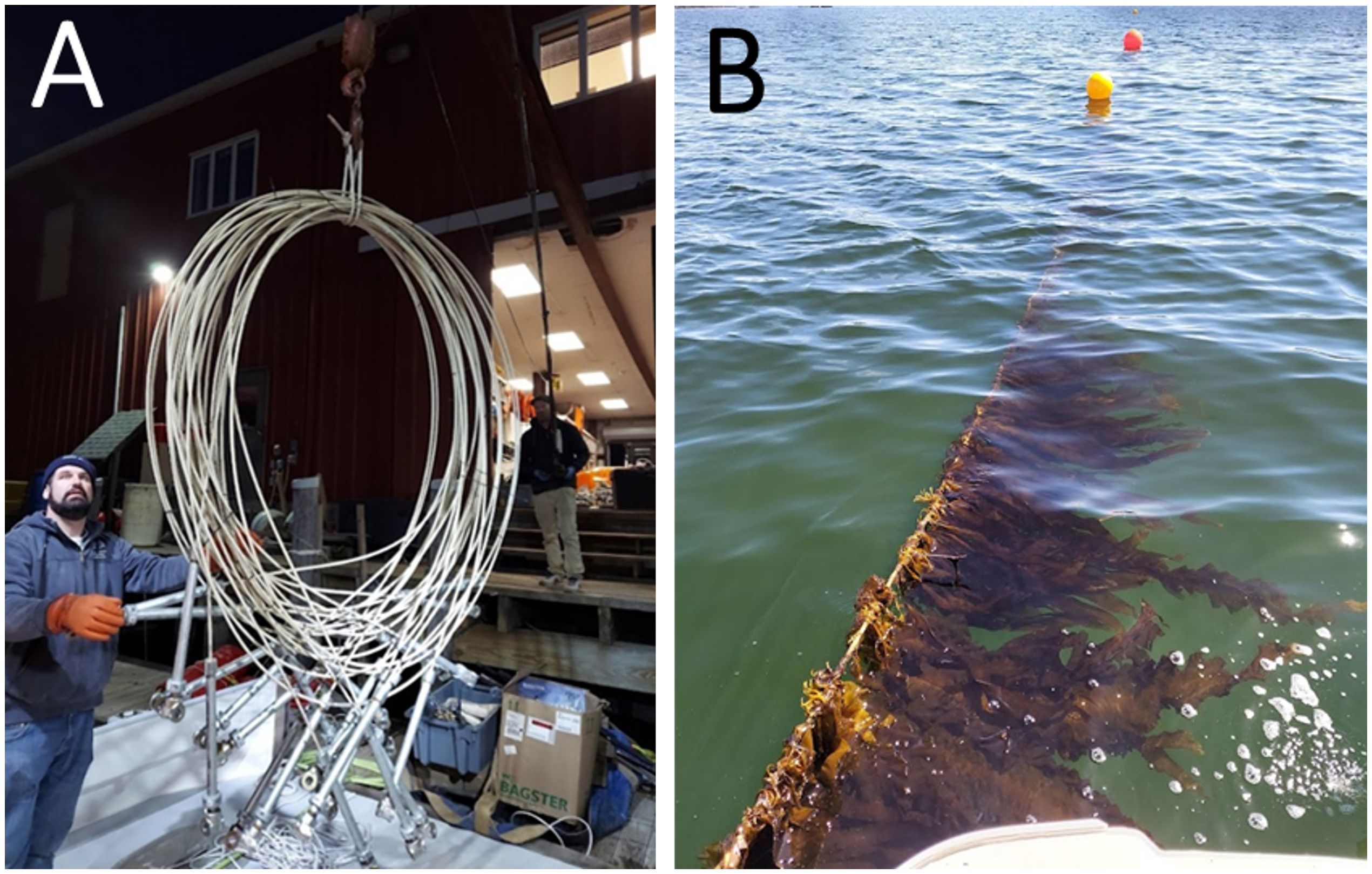
Figure 10. (A) Fiberglass rebar with terminations being loaded onto a vessel for deployment. (B) Photo of sugar kelp (Saccharina latissima) growing on the fiberglass rod.
3.2.3 Examples of exposed macroalgae aquaculture farms
Worldwide macroalgae aquaculture farms, which are located in highly exposed waters, are rare. Most of the farm structures that have been and are being built in such high-energy environments are more for research purposes and are still at an early stage of development. Therefore, in the present paper we decided to include examples of farms that can at least partially experience strong currents and higher waves.
A good example of a farm that is exposed to medium to large waves and currents, cultivating sugar kelp is Ocean Rainforest Sp/F based off the coast of the Faroe Islands. The technology of the farm is called ‘Macroalgal Cultivation Rig’ (MACR). The 500m long horizontal backbone is tensioned 5-10m below the water surface, held in position by up to four steel anchoring systems at the beginning and end, and buoyed into position in the water column. The line is connected between buoyancy devices and the cross lines, which makes operations and maintenance (O&M) easier. With this system, about 30 tonnes of sugar kelp per hectare per year can be achieved (Bak et al., 2020).
Engineering tools have been adopted by the University of New Hampshire in the Gulf of Maine. This has allowed kelp farming systems based on parallel, tensioned longlines to move to sites that may be exposed at certain times of the year. Finite element modelling (AquaFE) and physical scale testing in a wave basin provides preliminary data for their survival offshore (Moscicki et al., 2022). In addition, environmental monitoring buoys are placed close to the farm and measure temperature, dissolved oxygen, salinity, chlorophyll, dissolved organic matter, turbidity, pH, and nitrate as well as currents and waves. Storm events are recorded and loads on the mooring lines are measured to validate and update the AquaFE modelling software that aids in these efforts (Fredriksson et al., 2023).
The above-mentioned project funded by the ARPA-E-program was based on a farm that was exposed to stronger currents and higher waves at certain times of the year (Costa-Pierce and Fredriksson, 2022). It was moored by thirty-six 6.5m long helical anchors and utilised 12mm diameter fiberglass rebar for kelp culture and mooring lines. Special terminations were developed to attach the fiberglass rods to other lines in the farm via shackles. The farm was tensioned by surface buoys that maintained the grid structure 2.5m below surface during storm and tidal events. Fiberglass rod was chosen as it was similar in cost to traditional kelp culture lines and has a high tensile strength (10,000 kg). It can be rolled in a coil for shipment and deployment. Most notable is when it is bent to a certain radius, it will snap similar to uncooked spaghetti, avoiding wrapping marine mammals as rope does. This aspect makes this material attractive in reducing whale entanglement (Figures 9, 10). The farm was seeded in the fall of 2021 with juvenile kelp from Atlantic Sea Farms in Biddeford, Maine. It was harvested in the spring of 2022 at a yield of 8 kg/m (Chambers, personal experience). All the structures were removed from the site for further analysis onshore. No interactions with marine mammals were observed during the deployment. Analysis of the composite rod after deployment is ongoing. The composite kelp farm survived winter storms with waves up to 6 m in height (Chambers, personal experience). The positive results warrant further investigation.
For the farming of Ulva sp. In the EEZ of the German Bight, a design adapted from the Shellfish Tower was deployed (Heasman et al., 2021; Landmann et al., 2019) (Figures 5A–E). This design is referred to as the ‘Seaweed Tower’. The outer subunits were modified in such a way that cross bars were attached to create the largest possible surface area, either in a ‘star design’ from the inside to the outside (Figure 5A) or completely to the outside referred to as the cylinder system (Figure 5B). Both designs were built to measure the shading and therefore favour the design with the largest surface area. Laboratory tests were carried out in advance with many different rope arrangements, with the greatest yield being achieved with a chequered design (Figure 5C). Two of the six subunits will be used to hold gear (baskets) for the culture of eastern Oysters (Crassostrea virginica) in a test trial (Figures 5D, E).
3.3 Marine Finfish
Marine finfish are the most diverse group of marine species that are farmed although only a few species have been produced or trialled in exposed environments. Salmonids, primarily Atlantic salmon (Salmo salar) and steelhead trout (Oncorhynchus mykiss) have received the most attention as they are the predominant species produced in several major aquaculture nations, particularly those with advanced net pen industries (e.g. Norway, Chile, Scotland, Canada; FAO, 2022). Salmonids are well suited to production in high-energy environments, being athletic fish with a high optimal swim speed (Quinn et al., 2011; Hvas and Oppedal, 2017) and seeing benefits from forced exercise that strong currents can create (Waldrop et al., 2017; Prescott et al., 2023). Salmon, however, are less well suited for production in submerged net pens since they have physostomal swim bladders and require regular exposure to an air-water interface to maintain neutral buoyancy. Research on this topic indicates that culturing salmonids in submerged pens may be feasible by using submerged air pockets or raising the pen to the surface at set intervals (Sievers et al., 2021). Lindfors (2022) explored the underlying causes of different industry development paths between the offshore salmon industries in Norway and Tasmania, although the focus was on regional differences at the industry and regulatory levels.
Other species are receiving attention, particularly those suitable for tropical climes where the occurrence of hurricanes, typhoons, and other extreme weather events make almost any location at risk of encountering high energy conditions. Cobia (Rachycentron canadum) and several species of Seriola have been produced in commercial farms at exposed locations. Most species being farmed or considered for production at exposed sites are higher value fish as the high unit price is needed to overcome higher equipment depreciation and operating expenses. That price pressure may lessen as more high energy farms are started, enabling equipment to be produced in larger quantities, more vendor options to become available, and operating procedures to streamline.
3.3.1 Trends of current marine finfish commercial aquaculture systems
Marine finfish in sheltered locations are overwhelmingly produced using floating gravity pens (Lien et al., 2007). Most utilise a rim made of HDPE plastic pipe although some use steel frames. These styles of pens are cost effective and have seen some innovation over the past decade, mostly through increases in size and changes in the netting materials.
The pens are typically moored to a grid system composed of surface floats connected to anchors such that tension is maintained on all components (Huang et al., 2008). The consistent tension minimises movement and wear on the connection points and reduces anchor movement from the shock loads generated when a slack line comes quickly tensioned which often occurs when the tidal direction changes.
The fish are fed using one of two methods. Air-driven feed lines connected to a barge are the predominant method for delivering feed at salmon farms while farms in other locations often use “feed cannons” or are hand fed. In both cases, feed is delivered at the surface. Other farm operations such as harvesting are also dependent on surface access to the pen.
3.3.2 Trends enabling advancement of marine finfish farming into exposed sites
There are three broad categories with respect to net pen design employed in marine fish aquauclture; flexible gravity pens designed to conform to wave motion, rigid megastructures designed to resist wave energy, and submersible pens designed to evade the strongest surface energy (Wang et al. (2024) although Chu et al. (2020) describe 5 classifications of net pens using similar categories). Each of these strategies offers different advantages and disadvantages that make them each more or less preferable for a given project. The energy tolerance ranges that are appropriate for the net pen types are subject to the energy characteristics which affect equipment choice (i.e. a flexible gravity pen may be suitable for a site with 3m waves and a 15 second period, but not at a site with 3m waves and an 8 second period). Further, other project considerations such as business strategy, risk tolerance, available capital, distance from shore, technological sophistication, and personnel experience will influence the optimal equipment type.
Flexible gravity pens designed for open ocean environments differ from their nearshore counterparts being larger and utilising more highly engineered designs and materials. These pens are generally less robust than submersible pens or megastructures but are a cost-effective option for sites with less extreme conditions or longer period waves.
Rigid megastructures such as SalMar Aker Ocean’s Ocean Farm 2 or Pan Ocean Aquaculture and De Maas’ Semisubmersible Spar Fish Farm resemble offshore oil platforms more than aquaculture net pens. Nordlak’s HavFarm is another model that uses different design concepts. The HavFarm is 430m long and 54m wide (Wang et al., 2019; Chu et al., 2020) giving it an appearance similar to a large ship, and it has the ability to be propelled or rotate around a mooring point off its bow. The steel frame of these structures is strong enough to resist waves of up to 15m in height9. In addition to resisting surface energy, the megastructures allow for sensors and farm systems to be installed and powered. Chu et al. (2020) mentions feed silos and distribution systems, as well as water desalination units and oxygen generator on board the platforms. These systems are expected to reduce operating costs and help mitigate the high capital expenditure which, along with their large scale, could make the final cost of products sold similar to traditional systems.
Submersible pens can have different designs with some being fully enclosed at all times and designed to be operated in a submerged position, while others resemble surface pens and are intended to be operated at the surface most of the time to enable operations similar to traditional pens (Chu et al., 2020). The main driver to submerge pens is to escape the highest energy encountered near the surface (Lopez et al., 2024) but submerged pens may also be selected to reduce visual impacts, access cooler or more stable water temperatures, discourage theft or vandalism, or mitigate surface-based risks such as sea lice or some harmful algal species. Submersible pens that are operated at the surface such as Innovasea’s SeaProtean10, Badinotti’s Oceanis11, 12Akva’s Atlantis, or the SeaFisher being developed by the Blue Economy Cooperative Research Centre (Wang et al., 2023), are more compatible with existing equipment (e.g. harvest systems) and farming protocols. Submersible pens (Figures 11, 12) require deep water to accommodate free clearance above the pen to dissipate energy, the pen’s height, and free clearance below the pen to disperse effluence. This can limit the available locations suitable for submersible pens and often leads to farms being sited further from shore than traditional farms, imposing a logistical hurdle on the farmer. Waters deeper than 50m are usually sufficient for submersible pens although the minimum water depth varies based on the pen model. Large megastructure style pens also require deeper waters with the Ocean Farm 1 designed to be deployed in 100 – 300m of water (Chu et al., 2020). The top few meters of the water column often have other characteristics that farmers prefer to avoid. Sea lice and other parasites are more abundant near the surface (Nelson et al., 2017). In fact, several novel pen designs have been tested that exclude the top few meters of the water column from the pen and have shown reduced sea lice infections (Oppedal et al., 2017).
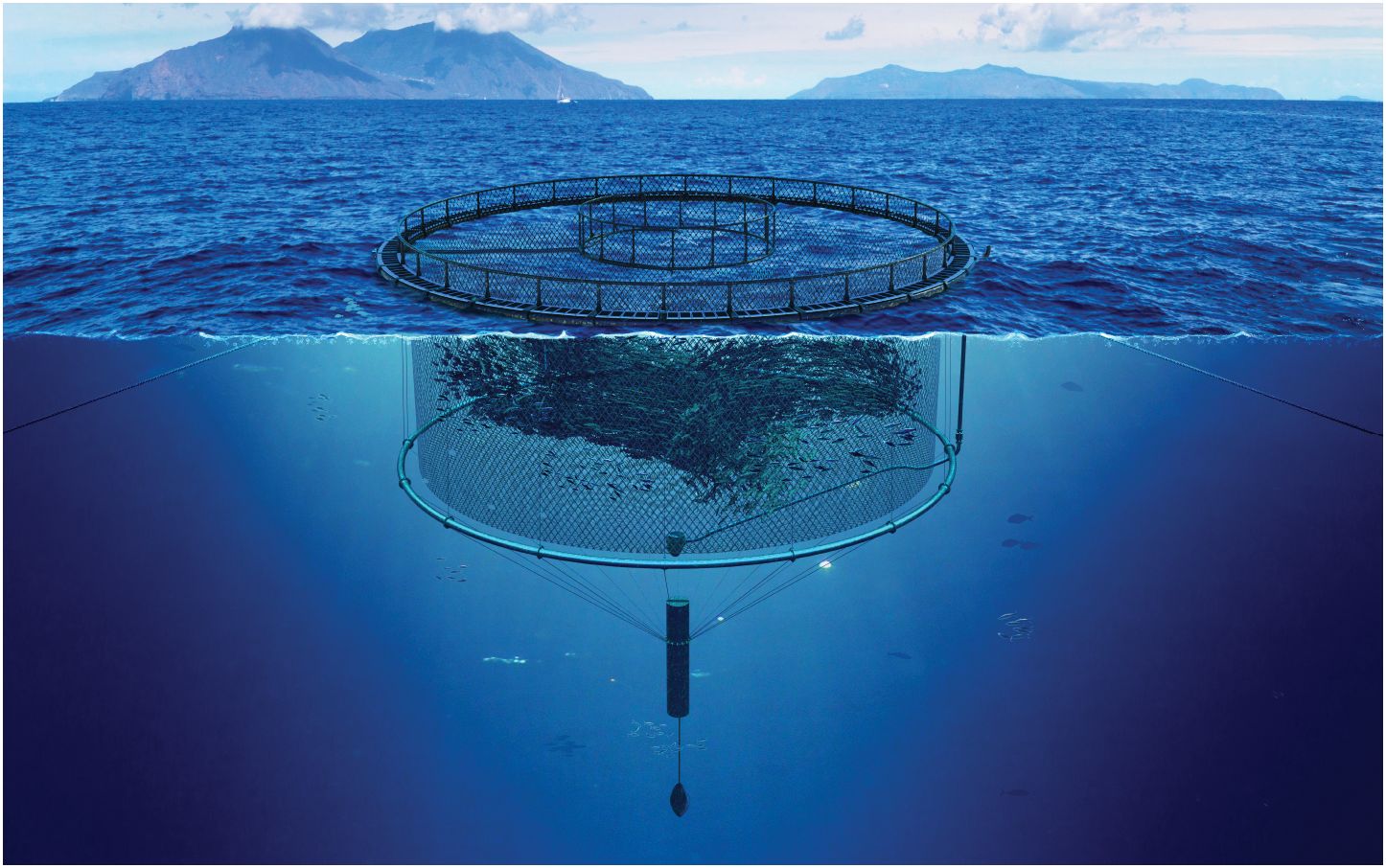
Figure 11. Innovasea’s SeaProtean pen is designed to look and operate like a surface pen, but with the ability to submerge during inclement weather.
Norwegian development licenses have been effective in encouraging the development of novel farming systems design for exposed environments (Føre et al., 2022). This includes both net pens as well as de-lousing, collection of waste, improved fish welfare, and surveillance systems which are important for the success of exposed farms although these innovations are not focused on exposed farms per se. Føre et al. (2022) report that 37% of the applications for development licenses and 39% of awarded licences were focused on open ocean environments (which they define as sites with an expected significant wave height above 4m). Of the 9 awarded open ocean development licenses, four were submersible and five were rigid structures. Chu et al. (2020) describes prototype marine finfish aquaculture pen designs that are not yet in commercial or semi-commercial use.
3.3.3 Examples of exposed marine finfish aquaculture farms
Open Blue Sea Farms13, located in Panamá, grows cobia (Rachycentron canadum) in 64 m of water. The site is exposed to the full fetch of the Caribbean Sea causing rough but manageable conditions during normal operations but conditions during a 50-year storm can be quite extreme (Heasman et al., 2024). To manage these forces, they use submersible SeaStation™ net pens to reduce the energy experienced by their equipment. They also use several operational strategies to streamline farm work. The submerged pens require a feed system that delivers feed underwater, so the farm uses a system called the FlowFeeder from Innovasea which transports feed to the pens in water and delivers it to pens while submerged. They also use a wireless sensor array to provide live data on temperature, oxygen, waves, and currents so farm managers can react faster to changing conditions. The farm has been operating at a commercial scale for over a decade.
Blue Ocean Mariculture, located in Hawai’i, experiences both rough normal conditions and episodic extreme events from hurricanes. During normal operating conditions, the farm is in the lee of the Big Island of Hawaii creating moderate energy conditions. However, the 50-year return conditions show extreme high energy conditions (Heasman et al., 2024). Blue Ocean Mariculture also uses SeaStation pens which have helped them withstand storms such as Hurricane Douglas (2020), Hurricane Erick (2019), and Hurricane Olivia (2018).
Santomar, located in Baja California Sur, Mexico, is a more protected site with calm conditions most of the time, but experiences hurricanes which can cause potentially damaging conditions (Heasman et al., 2024). Santomar uses Evolution Pens and SeaProtean (Figures 11 and 12A, B) pens supplied by Innovasea which look and operate like surface pens most of the time but can be submerged as needed when inclement weather is anticipated. This solution has worked well for Santomar as the frequency and severity of hurricanes requires a technical solution to make the farm feasible, but does not warrant adjustments to operating protocols that fully submerged pens would require. They are affected by hurricanes every few years, most recently Olaf in 2021, Bud in 2018, and Newton in 2016.
SalMar Aker Ocean operates two Atlantic salmon farms in exposed conditions. The Ocean Farm 1 is located near Hábranden, Norway, has a significant wave height of 5.5m and their Arctic Offshore Farming site near Fellesholmen, Norway, which has a significant wave height of 6.6m (Romuld, 2024). Both farm sites use rigid megastructure type pens. The Ocean Farm 1 is 64m tall and 110m in diameter, while the two systems at the Arctic Offshore Farming site are 78.5m tall and 78m in diameter (Romuld, 2024). The Arctic Ocean Farming site uses a double net with the top net 10m below the surface, limiting the salmon’s access to surface waters.
3.3.4 Other important developments “Attachment and Release systems”
If a structure is submerged to the point where there is only a marker float on the surface, a release mechanism is required to allow the structure to the surface for operations and maintenance. There are limited options at this time although there are some functional systems in pre commercial development. The most functional at this time is the patented set and forget system (Figure 13) found in conjunction with the Shellfish tower Heasman et al. (2021). It is a mechanism consisting of a cylindrical housing which is shaped with tapered sides. A cone of two halves is placed in this taper.
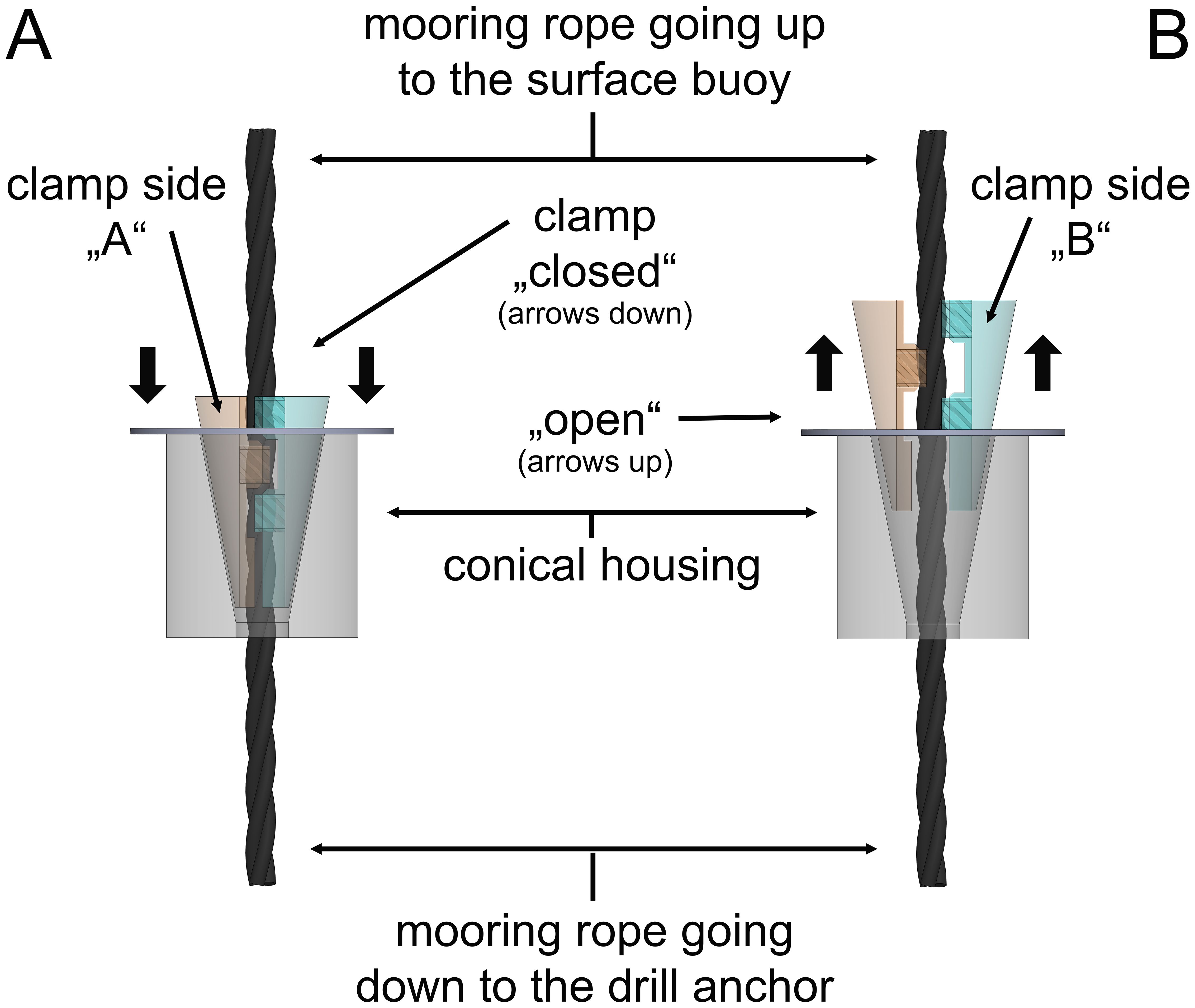
Figure 13. The set and forget clamp showing the housing in which there is a cone shaped taper into which the two half shells (green and tan) encompassing the rope are situated. (A) the clamps (green) and its opposing clamp (tan) are shown seated and the rope clamped between them. Pushing the housing down releases the two clamps (B) (green) and its opposing clamp (tan) which liberates the rope and allowing free movement.
Each half that sits in the taper has a toothed groove of the appropriate rope diameter (when under tension the rope diameter will reduce). The mooring rope goes through this mechanism. A hydraulically driven unit is attached to the top of the mooring rope which moves down the rope pushing the shellfish tower before it. At the desired depth the unit will stop and start coming up the mooring rope. The rising shellfish tower results in the mooring rope interacting with the two halves of the cones (green and tan in Figure 13) and the halves are pulled into the taper and tighten onto the rope holding the structure in place. When the structure is required to be released, the hydraulically driven unit or decoupler is sent down the mooring rope to disengage the two halves in the taper, releasing the rope and the buoyancy brings the shellfish to the surface.
A second system is being tested in New Zealand with the research project Nga Punga o te Moana14, which is used to attach ropes under tension. It relies on a coupler which consists of a male and female assembly, each attached to the ends of a rope that one needs to join. This system is being used on submerged longline systems to pull the structure under water and affix it such that it remains submerged. A simple release line is on the surface which can be used to trigger a release mechanism which allows the structure to surface.
4 Modern system design
4.1 Modelling
Several precursors were required to advance exposed aquaculture including computing tools such as finite element methods [FEM] and computer assisted design [CAD] (Fredriksson et al., 2007). These methods started in 1959 (Clough and Wilson, 1999) and only became available after breakthroughs in the late 1990’s regarding mathematics and rapid advances of the computer science (Liu et al., 2022).
Modelling is an effective means of testing new aquaculture structures in their early phases of development. It allows for rapid exploration of different prototypes under a variety of ocean scenarios.
Static modelling (the state of a structure at a specific point in time) is useful to determine whether there is sufficient buoyancy and ballast in the optimal places of an aquaculture structure for it to sit in the desirable part of the water column. Dynamic modelling (focusing on the system behaviour and interactions between components) is necessary to capture the influences of waves and currents.
For simpler line-based structures, programs such as Orcaflex™ are relatively straightforward and fast-solving model. Some limitations of programs such as Orcaflex are that they cannot easily replicate objects with complex geometries and they only account for the effect of the structure on flow – which may be important where parts of the structure are blocking flow or cause flow to accelerate downstream. However, Orcaflex also does not model internal flow structures such as where some parts of the flow go around the object while other parts go through (e.g. an intake and pipe).
For structures with unusual geometries, structures where turbulent processes are important, or where drag and lift dynamics are important, a more complex CFD (computational fluid dynamics) model (e.g. fluent, openFOAM, reef3d) will likely need to be adopted. The more complex the model, the more computational power is required to solve it, and the longer it will take to solve. This can slow down the modelling process.
After initial sandbox design, modelling can also be undertaken to further refine aspects of design. Reducing drag, optimising lift, or reducing turbulence generation will require more complex CFD modelling. Modelling does however provide the means to study, test and de-risk innovative ideas quickly and relatively cheaply prior to committing to the development and deployment of prototype structures.
Wave tanks, flow tanks, and various other test tanks that can simulate ocean conditions are more readily available than they have been in the past, usually through academic institutions. These facilities allow for the testing of scaled-down models which can generate representative empirical data on drag coefficients or relevant factors (Swift et al., 2006).
4.2 Materials
Materials are advancing and there are an increasing number of materials available for structures and crops. Different materials offer durability, corrosion resistance, abrasion resistance, longevity, flexibility, cost effectiveness, and recyclability, however there are few, if any, that will offer all these parameters. Selection of materials is incredibly important as they will have a significant influence on the success of any enterprise.
HDPE is used across aquaculture as it has excellent strength, toughness, erosion and UV resistance, and inertness (Wesley, 2020). In shellfish, macroalgae, and marine finfish aquaculture it is used primarily for floatation. Floats range in size from marker floats and 300l backbone (header rope) support floats (Goseberg et al., 2017; Newell et al., 2021) to large diameter circular pipes which provide floatation for fish pens (Fredriksson et al., 2007). Baskets and trays used in shellfish aquaculture are made from HDPE (Newell et al., 2021). HDPE can however be fouled with some variation depending on the colour of the substrate and the positioning (geographically and in the water column) of the substrate (Freitas et al., 2023).
Fish pen floatation rings have been made with HDPE or other polymers. There is increasing research into the behaviour of pen nets in various conditions (Chen et al., 2019, Liu et al., 2020; Fan et al., 2023) and use of alternatives to the polymers such as alloys (Chen et al., 2019; Sclodnick et al., 2020; Gümpel et al., 2021) for the nets of fish pens.
Polyethylene and polypropylene are also used extensively for ropes to secure the structures (moorings etc.) but also for the growing media (e.g. mussel cultivation ropes) (Newell et al., 2021) and are well suited to the demands required of them (Maddah, 2016; Arantzamendi et al., 2023). Synthetic fibre ropes are a good replacement for steel cables both in terms of weight and corrosion and they can be combined in terms of mixing the fibres or by constructing the ropes in different ways (e.g. eight strand or double braded etc.) (Foster, 2002). Cost is, however, a serious consideration with some fibres (e.g. Kevlar and Dynema®) as while they have improved properties in terms of strength and stretch, but they are more expensive (Foster, 2002).
As useful as these plastics are, they are contributing to the microplastic load in the marine habitat (Gomiero et al., 2020; Iheanacho et al., 2023). While alternatives ropes of other materials are being considered (Arantzamendi et al., 2024), the true benefits, durability, and cost of these new ropes do not appear to have been assessed.
Exposed environments lend themselves to different materials for marine finfish containment nets. Low energy sites often use a woven net of nylon or Dyneema (an ultra-high-molecular-weight polyethylene), which are also used at exposed sites, but the stronger forces make other options that can reduce drag more attractive. Some farms are using an extruded monofilament of PET plastic called Kikko net which creates less drag and fouls slower than fibrous nets (Bannister et al., 2019). Other farms are using copper alloys which are also smoother than fibrous nets and the copper provides a natural fouling retardant (Yigit et al., 2018; Bannister et al., 2019). Foster (2008) provides information and outlines a number of materials that are used increasingly in the development of exposed aquaculture while Ashby (2016) highlights the complexities and properties of materials in general, some of which could be used in aquaculture.
4.3 Future developments
The ongoing development of genetic tools will have significant implications in advancing the production of efficient and environmentally tolerant aquatic organisms. This is a necessity when taking organisms into different environments and in view of the influences of climate change. More rapid growth rates through selective breeding reduce the time organisms are in production, which in turn reduces the exposure period, husbandry requirements, and costs. Value added characteristics (e.g. uniform sizes and shapes), will contribute to the viability of operations. The production of triploid bivalves has the benefits of maintaining condition of the animal for longer periods than diploid organisms, alleviating the necessity to lose condition and harvest prospects during inclement weather at inshore but mainly at exposed sites. New materials such as composite growing rods are being tested which address some issues (e.g. marine mammal entanglement) as they will not wrap or fold over appendages and entangle the organism (Moscicki et al., 2024).
Environmental and structure sensors will become more important with increasing exposure. Knowledge of the conditions at the site, under the water and on the structure, will significantly improve management, efficiencies, survivability, and reduce costs. Fortunately, sensors are becoming cheaper and more robust, however the ability to overlay data sets to develop predictability and assessment tools is still in its infancy.
5 Discussion
In terms of knowledge, development, and advancement of commercial production in exposed sites, marine finfish aquaculture are far in advance of bivalve aquaculture, which in turn are more advanced than macroalgae production. Some of the advancement can be related to the value and demand for fish but every fish is cultivated for a single purpose - food (with a few cultivated species to assist with husbandry such as lumpfish, Cyclopterus lumpus) i.e. a protein source. There is generally sufficient value in the fish for expansion to exposed sites to be viable. Bivalves are also cultivated for food but are of lower value and therefore expansion is more tentative and requires far larger space. Although macroalgae have been cultivated for millennia for food (Buschmann et al., 2017), their true value in terms of uses is widespread (Farghali et al., 2023) and still being discovered. Macroalgae are also known to change their characteristics when subjected to higher energy and different light regimes (Maltsev et al., 2021). Macroalgae species occupy a large spectrum of habitats, and each has their particular biochemistry and physical attributes (Buschmann et al., 2017). Therefore, each macroalgae species will need to be analysed for these attributes and also for how the energy and light variations influence them. The opportunities in macroalgae are extensive, however, the cultivation/husbandry/processing methods, O&M, equipment, etc. still need to be developed for a large proportion of the macroalgae species. The few species that have been developed (e.g. Saccharina sp., Laminaria sp., Undaria sp.) provide the foundation of future development and the basic knowledge required to progress.
Although there are specific requirements for the farming of bivalves, macroalgae and marine finfish, there are some common characteristics which are found across all exposed ocean structures: all are subjected to greater forces; all will require more robust design; modified and more robust mooring systems are required; and suitable and cost-efficient materials must be identified.
To endure the greater energy in exposed situations, increased structural integrity and maintenance requirements are needed which result in escalating costs (Chu et al., 2020; Dewhurst et al., in review). Operators can therefore design structures which can tolerate sea surface conditions (Wang et al., 2022) and improve efficiencies in other ways (e.g. sensors, food efficiencies, automation) (Solvang et al., 2021; Wu et al., 2022; Scholtens et al., 2023), or they can avoid the main surface energy by semi or fully submerging structures (Wang et al., 2023). If the structures are linear, they can be orientated such that they respond less aggressively to the main energy sources (waves and water currents) reducing wear, maintenance, and crop loss15.
Macroalgae aquaculture is a challenge in that, with the exception of a few deep-water kelps, species must remain close to the surface in the sunlight. Novel approaches are being developed to address the ability of structures to tolerate the surface energy (St-Gelais et al., 2022), be marine mammal friendly, and be commercially viable (Moscicki et al., 2024). In some respects, however, the (in)ability of the specific species of macroalgae tolerance of energy being propagated may override the energy tolerance of the cultivation structure as the macroalgae may be damaged before the structure is stressed.
6 Conclusions
6.1 Limitations
This manuscript mentions social license, however as it is a topic deserving of its own manuscript it is covered elsewhere in this special issue by Krause et al. (2024). This is not intended to disrespect or diminish the importance and implications of social licence aspects in any way. It has been assumed that due diligence in this regard has, and will continue to be, considered should any party wish to expand into exposed ocean aquaculture.
There are many parameters that should be considered when selecting a site for aquaculture. One of these is the provision of power for vessels and operation of equipment (as found on some marine finfish farms). Consideration should be given to the efficiency and carbon footprint of the derived power. This has not been discussed in this manuscript due to the diverse and now expanding (e.g. battery and hydrogen fuel cells) opportunities provided by alternative power sources.
6.2 Current obstacles
● De-risking the expansion into exposed oceans is key. Only in recent years have the risks (cost and environment) become known as exposed ocean endeavours are successful, which is providing greater enthusiasm to innovate and succeed in this environment. However, there is still some way to go.
● The exposed ocean aquaculture environment is relatively unknown from an oceanographic perspective simply due to the fact that many of the sites have not been assessed for aquaculture parameters. Assessments are required to determine the species and equipment required for the site. Certain assessments take time, e.g. growth rates, fouling assemblages, hydrodynamics over a season, etc. These assessments can be costly and require permits which are time consuming.
● Social licence is very important and not limited to exposed ocean usage. However, a lack of knowledge of a site (and aquaculture in general) can lead to misconceptions and inadvertent conclusions leading to complications with permits, etc.
● Materials to be used on the exposed sites are available, however there are requirements that will facilitate increased reliability, durability, and efficiencies. In addition, they would preferably be recyclable and relatively inexpensive. They should also lead to future semi or full automation. There are very few, if any, materials which currently can meet all these requirements.
● It is unknown how some species will respond to the environment and new structures. Therefore, new methods (husbandry, harvesting, and operations) need to be developed. This will be followed by health and safety requirements for the crews.
● In some instances (e.g. macroalgae) products and markets need to be further developed. There is an issue of balance between the demands of the markets and the expansion of aquaculture sites. The ability to expand both simultaneously in sync with each other is a desirable but difficult task.
● The value of products grown in exposed ocean sites have to cover the extra cost of equipment and operations that the exposed environment imposes on a business.
The advancements that have enabled farmers and researchers to utilise higher energy environments have come primarily from: engineers identifying issues and advancing technical feasibility of materials and structures; researchers studying species suitability and requirements and the environment and net benefits. These advancements have been successful as the examples described indicate. However, the expansion of the aquaculture industry into exposed waters and the contribution to sustainable development goals (Troell et al., 2023) has not been as fast or extensive as the market demand for seafood or spatial opportunities in exposed waters warrants. The perceived risk, increased capital costs, and more difficult operations remain as major deterrents for new entrants into this sub-sector.
One of the motivations of this manuscript is to broaden awareness for the advancements that make exposed farming feasible, and to highlight the success that some groups are having. Yet, further work is required to get the cost of goods sold from exposed farms consistently in line with products from wild fisheries or sheltered farms as they are effectively perfect substitutes, or new to the market.
Data availability statement
The original contributions presented in the study are included in the article/supplementary material. Further inquiries can be directed to the corresponding author.
Author contributions
KH: Conceptualization, Funding acquisition, Investigation, Methodology, Project administration, Supervision, Writing – original draft, Writing – review & editing. NS: Investigation, Methodology, Writing – original draft, Writing – review & editing. TS: Conceptualization, Investigation, Methodology, Validation, Writing – original draft, Writing – review & editing. MC: Investigation, Methodology, Validation, Writing – original draft, Writing – review & editing. BC: Methodology, Validation, Writing – original draft, Writing – review & editing. TD: Methodology, Validation, Writing – original draft, Writing – review & editing. WI: Methodology, Validation, Writing – original draft, Writing – review & editing. BB: Conceptualization, Investigation, Methodology, Validation, Visualization, Writing – original draft, Writing – review & editing.
Funding
The author(s) declare financial support was received for the research, authorship, and/or publication of this article. This manuscript was partially funded by the New Zealand Ministry of Business, Innovation and Employment through the “Nga Puinga o te Moana” program (CAWX2102).
Conflict of interest
Author BC-P was employed by the company Ecological Aquaculture International, LLC. Author TD was employed by the company Kelson Marine Co. TS works is employed by Innovasea.
The remaining authors declare that the research was conducted in the absence of any commercial or financial relationships that could be construed as a potential conflict of interest.
Publisher’s note
All claims expressed in this article are solely those of the authors and do not necessarily represent those of their affiliated organizations, or those of the publisher, the editors and the reviewers. Any product that may be evaluated in this article, or claim that may be made by its manufacturer, is not guaranteed or endorsed by the publisher.
Footnotes
- ^ International Council for the Exploration of the sea
- ^ ICES Working Group Open Ocean Aquaculture
- ^ ICEA Working Group on Social and Economic Dimensions of Aquaculture
- ^ Advanced Research Projects Agency – Energy, U.S. Department of Energy
- ^ www.Smartfarm.no
- ^ www.flipfarm.com
- ^ A Boost to the Biological Carbon Pump « World Ocean Review
- ^ https://aquavitaeproject.eu/
- ^ David & Lucil Packard Foundation 2019
- ^ www.innovasea.com/open-ocean-aquaculture/submersible-aquaculture-systems/seaprotean-pen/
- ^ https://www.badinotti.com/marine/submergible-cages/
- ^ https://www.akvagroup.com/sea-based/deep-farming-lice-control/
- ^ https://openblue.com
- ^ Nga Punga o te Moana, Ministry of Business, Innovation and Employment funded project, New Zealand.
- ^ www.openocean@cawthron.org.nz
References
Arantzamendi L., Andrés M., Basurko O. C., Suárez M. J. (2023). Circular and lower impact mussel and seaweed aquaculture by a shift towards bio-based ropes. Rev. Aquac. 15, 1010–1019. doi: 10.1111/raq.12816
Arantzamendi L., Andrés M., Suárez M. J., van der Schueren L., Aguinaga M. (2024). Assessing the mechanical properties of biobased versus fossil-based ropes and their impact on the productivity and quality of mussel (Mytilus galloprovincialis) in longline aquaculture: Toward decarbonization. Aquaculture 588, 740919. doi: 10.1016/j.aquaculture.2024.740919
Ashby M. F. (2016). Materials Selection in Mechanical Design, 5th Edition. (Burlington, MA: Butterworth-Heinemann), 660 p.
Bak U. G., Gregersen Ó., Infante J. (2020). Technical challenges for offshore cultivation of kelp species: lessons learned and future directions. Botanica Marina 63, 341–353. doi: 10.1515/bot-2019-0005
Bannister J., Sievers M., Bush F., Bloecher N. (2019). Biofouling in marine aquaculture: a review of recent research and developments. J. Bioadhesion Biofilm Res. 35, 631–648. doi: 10.1080/08927014.2019.1640214
Bourque F., Myrand B. (2014). Potentiel de production mytilicole en milieu ouvert aux Iles-de-la-Madeleine (Merinov, R&D Report, 14-09), 33 p.
Buck B. H. (2007). Experimental trials on the feasibility of offshore seed production of the mussel mytilus edulis in the german bight: Installation, technical requirements and environmental conditions. Helgoland Mar. Res. 61, 87–101. doi: 10.1007/s10152-006-0056-1
Buck B. H., Krause G., Rosenthal H. (2004). Extensive open ocean aquaculture development within wind farms in germany: the prospect of offshore co-management and legal constraints. Ocean Coast. Manage. 47 (3-4), 95–122. doi: 10.1016/j.ocecoaman.2004.04.002
Buck B. H., Bjelland H. V., Bockus A., Chambers M., Costa-Pierce B. A., Dewhurst T., et al. (2024a). Resolving the term “offshore aquaculture” by decoupling “exposed” and “distance from the coast. Front. Aquac. 3, 1428056. doi: 10.3389/faquc.2024.1428056
Buck B. H., Buchholz C. M. (2004). The offshore-ring: a new system design for the open ocean aquaculture of macroalgae. J. Appl. Phycol. 16, 355–368. doi: 10.1023/B:JAPH.0000047947.96231.ea
Buck B. H., Buchholz C. M. (2005). Response of offshore cultivated Laminaria saccharina to hydrodynamic forcing in the North Sea. Aquaculture 250, 674–691. doi: 10.1016/j.aquaculture.2005.04.062
Buck B. H., Grote B. (2018). “Seaweed in High energy environments: protocol to move saccharina cultivation offshore,” in Protocols for Macroalgae Research. Eds. Charrier B., Wichard T., Reddy C. R. K. (Boca Raton: CRC Press, Taylor & Francis Group)ISBN-13: 978-1498796422 - ISBN-10: 1498796427. doi: 10.1201/b21460
Buck B. H., Isbert W., Durst S. (2024b). “Application for the construction, operation or modification of facilities used for oceanographic investigations pursuant to Section 1 (2) No. 4 Marine Facilities Act (measuring stations)”, in Installation of a test facility for the culture of mussels and algae. OLAMUR EU project, code: Horizon-Miss-2021-Ocean-04-01, Grant agreement ID: 101094065.
Buck B. H., Langan R. (2017). “Aquaculture perspective of multi-use sites in the open ocean,” in The Untapped Potential for Marine Resources in the Anthropocene. Eds. Bela B. H., Richard L. (Springer, Cham), 404.
Buschmann A. H., Camus C., Infante J., Neori A., Israel A., Hernández-González M. C., et al. (2017). Seaweed production: overview of the global state of exploitation, farming and emerging research activity. Eur. J. Phycol. 52, 391–406. doi: 10.1080/09670262.2017.1365175
Chambers M. D., Howell W. H., Langan R., Celikkol B., Fredriksson D. (2003). Status of open ocean aquaculture in New Hampshire. Open Ocean Aquaculture: from Research to Reality. Baton Rouge, Louisiana: The World Aquaculture Society. JEL Contribution Series, (388), 233–246.
Chambers M., Langan R., Howell W., Celikkol B., Watson K. W., Barnaby R., et al. (2007). Recent Developments at the University of New Hampshire Open Ocean Aquaculture Site. Bull. Aquacul. Assoc. Canada, 105–103. Available at: http://aquacultureassociation.ca/wp-content/uploads/bsk-pdf-manager/2022/02/25-Cod-Aquaculture-105-3.pdf.
Chambers M. D., Moscicki Z., Lynn P., Dewhurst T., Zotter B. (2023). A new paradigm in offshore macroalgae farming: reducing the risk of marine mammal entanglement. World Aquac., 65–67.
Chen C., Liu H., Huang Y., Yang J., Liang X., Zhang C., et al. (2019). Numerical simulation of mechanical characteristics of a metal net for deep-sea aquaculture. J. Ocean Univ. China 18, 1273–1281. doi: 10.1007/s11802-019-4079-z
Chu Y. I., Wang C. M., Park J. C., Lader P. F. (2020). Review of cage and containment tank designs for offshore fish farming. Aquaculture 519, 734928. doi: 10.1016/j.aquaculture.2020.734928
Clough R. W., Wilson E. L. (1999). “Early finite element research at Berkeley,” in Fifth US national conference on computational mechanics, (University of California, Berkeley), pp 1–35.
Costa-Pierce B. A., Fredriksson D. W. (2022). A Validated Finite Element Modeling Tool for Hydrodynamic Loading and Structural Analysis of Ocean Deployed Macroalgae Farms (Biddeford, Maine (US: Technical Report No. DOE-UNE-00917. University of New England), 245 pp. doi: 10.2172/2371708
Fan Z. Q., Liang Y. H., Yun-Peng Z. (2023). Review of the research on the hydrodynamics of fishing cage nets. Ocean Engineering 276, 114192. doi: 10.1016/j.oceaneng.2023.114192
Fan W., Zhang Z., Yao Z., Xiao C., Zhang Y., Zhang Y., et al. (2020). A sea trial of enhancing carbon removal from Chinese coastal waters by stimulating seaweed cultivation through artificial upwelling. Appl. Ocean Res. 101, 102260. doi: 10.1016/j.apor.2020.102260
Fan W., Zhao R., Yao Z., Xiao C., Pan Y., Chen Y., et al. (2019). Nutrient removal from Chinese coastal waters by large-scale seaweed aquaculture using artificial upwelling. Water 11, 1754. doi: 10.3390/w11091754
FAO (Food and Agriculture Organization of the United Nations). (2022). The State of World Fisheries and Aquaculture 2022. Towards Blue Transformation (Rome: FAO).
Farghali M., Mohamed I. M., Osman A. I., Rooney D. W. (2023). Seaweed for climate mitigation, wastewater treatment, bioenergy, bioplastic, biochar, food, pharmaceuticals, and cosmetics: a review. Environ. Chem. Lett. 21 (1), 97–152. doi: 10.1007/s10311-022-01520-y
Fernand F., Israel A., Skjermo J., Wichard T., Timmermans K. R., Golberg A. (2017). Offshore macroalgae biomass for bioenergy production: Environmental aspects, technological achievements and challenges. Renewable Sustain. Energy Rev. 75, 35–45. doi: 10.1016/j.rser.2016.10.046
Føre H. M., Thorvaldsen T., Osmundsen T. C., Asche F., Tveterås R., Fangertun J. T., et al. (2022). Technological innovations promoting sustainable salmon (Salmo salar) aquaculture in Norway. Aquac. Rep. 24, 101115. doi: 10.1016/j.aqrep.2022.101115
Foster J. (2008). “Emerging technologies in marine aquaculture,” in Offshore Aquaculture in the United States: Economic Considerations, Implications & Opportunities, July 2008 (Pre-Publication Copy). Available at: http://aquaculture.noaa.gov.
Foster G. P. (2002). Advantages of fiber rope over wire rope. J. Ind. Textiles 32 (1), 67–75. doi: 10.1106/152808302031656
Fredriksson D. W., DeCrew J. C., Tsukrov I. (2007). Development of structural modeling techniques for evaluating HDPE plastic net pens used in marine aquaculture. Ocean Engineering 34, 2124–2137. doi: 10.1016/j.oceaneng.2007.04.007
Fredriksson D. W., St-Gelais A. T., Dewhurst T., Coleman S., Brady D. C., Costa-Pierce B. A. (2023). Mooring tension assessment of a single line kelp farm with quantified biomass, waves, and currents. Front. Sec. Mar. Fish Aquac. Living Res. 10. doi: 10.3389/fmars.2023.1178548
Freitas V., Gonçalves O., Dolbeth M., Ramos S., Morais J., Ozorio R. O. D. A., et al. (2023). Optimization of plastic polymers for shellfish aquaculture infrastructures: in situ antifouling performance assessment. Front. Mar. Sci. 10, 1229634. doi: 10.3389/fmars.2023.1229634
Gagnon M. (2024). Status of off-bottom mariculture in wave-exposed environments. Part 1. Global inventory of extractive species commercial farms in temperate waters. Frontiers in Aquaculture 3, 1411749.
Galparsoro I., Murillas A., Pinarbasi K., Sequeira A. M., Stelzenmüller V., Borja Á., et al. (2020). Global stakeholder vision for ecosystem-based marine aquaculture expansion from coastal to offshore areas. Rev. Aquac. 12, 2061–2079. doi: 10.1111/raq.12422
Gibbs M. T. (2009). Implementation barriers to establishing a sustainable coastal aquaculture sector. Mar. Policy 33, 83–89. doi: 10.1016/j.marpol.2008.04.004
Gomiero A., Haave M., Bjorøy Ø., Herzke D., Kögel T., Nikiforov V., et al. (2020). Quantification of microplastic in fillet and organs of farmed and wild salmonids-a comparison of methods for detection and quantification. Report Number 8-2020, NORCE, Bergen, Norway.
Gonzalez-Poblete E., Rojo C., Norambuena R. (2018). Blue mussel aquaculture in Chile: small or large scale industry? Aquaculture 493, 113–122. doi: 10.1016/j.aquaculture.2018.04.026
Goseberg N., Chambers M. D., Heasman K., Fredriksson D., Fredheim A., Schlurmann T. (2017). Technological approaches to longline-and cage-based aquaculture in open ocean environments (Springer International Publishing, Switzerland), 71–95.
Gümpel P., Dornbierer U., Hörtnagl A., Bogatzky T. (2021). “New development: high-strength stainless steel as a sustainable material for aquaculture,” in Salmon Aquaculture (IntechOpen). doi: 10.5772/intechopen.98680
Güneralp B., Reba M., Hales B. U., Wentz E. A., Seto K. C. (2020). Trends in urban land expansion, density, and land transitions from 1970 to 2010: A global synthesis. Environ. Res. Lett. 15, 044015. doi: 10.1088/1748-9326/ab6669
Handå A., McClimans T. A., Reitan K. I., Knutsen Ø., Tangen K., Olsen Y. (2014). Artificial upwelling to stimulate growth of non-toxic algae in a habitat for mussel farming. Aquac. Res. 45, 1798–1809. doi: 10.1111/are.12127
Harvey M., Barrett L. T., Morris R. L., Swearer S. E., Dempster T. (2024). Ocean sprawl: The global footprint of shellfish and algae aquaculture and its implications for production, environmental impact, and biosecurity. Aquaculture 586, 740747. doi: 10.1016/j.aquaculture.2024.740747
Heasman K. G., Sclodnick T., Goseberg N., Scott N., Chambers M., Dewhurst T., et al. (2024). Utilisation of the site assessment energy indices for aquaculture in exposed waters: biology, technology, operations and maintenance. Front. Aquac. 3, 1427168. doi: 10.3389/faquc.2024.1427168
Heasman K. G., Scott N., Smeaton M., Goseberg N., Hildebrandt A., Vitasovich P., et al. (2021). New system design for the cultivation of extractive species at exposed sites-Part 1: System design, deployment, and first response to high-energy environments. Appl. Ocean Res. 110, 102603. doi: 10.1016/j.apor.2021.102603
Holdt S. L., Kraan S. (2011). Bioactive compounds in seaweed: functional food applications and legislation. J. Appl. Phycol. 23, 543–597. doi: 10.1007/s10811-010-9632-5
Huang C.-C., Tang H.-J., Lui J.-Y. (2008). Effects of waves and currents on gravity-type cages in the open sea. Aquac. Eng. 38, 105–116. doi: 10.1016/j.aquaeng.2008.01.003
Hvas M., Oppedal F. (2017). Sustained swimming capacity of Atlantic salmon. Aquac. Environ. Interactions 9, 361–369. doi: 10.3354/aei00239
Idhalla M., Nhhala H., Kassila J., Ait Chattou E. M., Orbi A., Moukrim A. (2017). Comparative production of two mussel species (Perna perna and Mytilus galloprovincialis) reared on an offshore submerged longline system in Agadir, Morocco. International Journal of Scientific & Engineering Research 8 (6), 1203–1213. doi: 10.19044/esj.2018.v14n12p229
Iheanacho S., Ogbu M., Bhuyan M. S., Ogunji J. (2023). Microplastic pollution: An emerging contaminant in aquaculture. Aquac. Fisheries 8, 603–616. doi: 10.1016/j.aaf.2023.01.007
Inglis G. J., Hayden B. J., Ross A. H. (2000). An overview of factors affecting the carrying capacity of coastal embayments for mussel culture (Hamilton, New Zealand: NIWA) Report number: Client Report: CHC00/69, 38 pages.
Jürchott M., Oschlies A., Koeve W. (2023). Artificial upwelling—A refined narrative. Geophysical Res. Lett. 50, e2022GL101870. doi: 10.1029/2022GL101870
Kamermans P., Capelle J. J. (2019). “Global production of marine bivalves. Trends and challenges,” in Goods and services of marine bivalves. Ed. Smaal A. C., Ferreira J. G., Grant J., Petersen J. K., Strand Ø. (Switzerland: Springer Nature).
Kämpf J., Chapman P. (2016). “The functioning of coastal upwelling systems,” in Upwelling Systems of the World (Springer, Cham). doi: 10.1007/978-3-319-42524-5_2
Kemper J., Riebesell U., Graf K. (2022). Numerical flow modelling of artificial ocean upwelling. Front. Mar. Sci. 8. doi: 10.3389/fmars.2021.804875
Kimmel W. M., Beauchamp P. M., Frerking M. A., Kline T. R., Vassigh K. K., Willard D. E., et al. (2020). Technology readiness assessment best practices guide. SP-20205003605.
Krause G., Weitzman J., Rector M. E., Filgueira R., Burg Svd., Dankel D. J., et al. (2024). The social science of offshore aquaculture: uncertainties, challenges and solution-oriented governance needs. Front. Aquac. 3, 1384037. doi: 10.3389/faquc.2024.1384037
Landmann J., Ongsiek T., Goseberg N., Heasman K., Buck B. H., Paffenholz J. A., et al. (2019). Physical modelling of blue mussel dropper lines for the development of surrogates and hydrodynamic coefficients. J. Mar. Sci. Eng. 7, 65. doi: 10.3390/jmse7030065
Lien E., Fredheim A. (2001). Development of longtube mussel systems for cultivation of mussels (Mytilis edulis). In: Proceedings of OOA IV, Open Ocean Aquaculture IV, St Andrews, New Brunswick, Canada, 137–141.
Lien E., Sunde L. M., Midling K. (2007). “Aquaculture technology,” in Aquaculture Research: From Cage to Consumption. Eds. Thomassen M., Gudding R., Norberg B., Jørgensen L. (Oslo, Norway: The Research Council of Norway), 304.
Lindfors E. T. (2022). Radical path transformation of the Norwegian and Tasmanian salmon farming industries. Regional Studies Regional Sci. 9, 757–775. doi: 10.1080/21681376.2022.2148555
Liu W. K., Li S., Park H. S. (2022). Eighty years of the finite element method: birth, evolution, and future. Arch. Computat. Methods Eng. 29, 4431–4453. doi: 10.1007/s11831-022-09740-9
Lojek O., Goseberg N., Føre H. M., Dewhurst T., Bölker T., Heasman K. G., et al. (2024). Hydrodynamic exposure - On the quest to deriving quantitative metrics for mariculture sites. Front. Aquac., 1388280.
Lopez J., Hurtado C. F., Toledo J. P., Suazo G., Zamora V., Queirolo D., et al. (2024). Structural integrity of a submersible sea cage exposed to extreme oceanographic conditions. J. World Aquac. Soc. 55, e13052. doi: 10.1111/jwas.13052
Liu H. F., Bi C. W., Zhao Y. P. (2020). Experimental and numerical study of the hydrodynamic characteristics of a semisubmersible aquaculture facility in waves. Ocean Engineering 214, 107714. doi: 10.1016/j.oceaneng.2020.107714
Lüning K., Buchholz C. (1996). Massenkultur mariner makroalgen bei helgoland zur gewinnung vonPhykokolloiden und zur verwendung als biosorptionsmittel (Helgoland: BAH), 75 pp.
Maddah H. A. (2016). Polypropylene as a promising plastic: A review. Am. J. Polym. Sci. 6, 1–11. doi: 10.5923/j.ajps.20160601.01
Maltsev Y., Maltseva K., Kulikovskiy M., Maltseva S. (2021). Influence of light conditions on microalgae growth and content of lipids, carotenoids, and fatty acid composition. Biology 10, 1060. doi: 10.3390/biology10101060
Mascorda Cabre L., Hosegood P., Attrill M. J., Bridger D., Sheehan E. V. (2021). Offshore longline mussel farms: a review of oceanographic and ecological interactions to inform future research needs, policy and management. Rev. Aquac. 13, 1864–1887. doi: 10.1111/raq.12549
Mckindsey C. W., Archambault P., Callier M. D., Frederic O. (2011). Influence of suspended and off-bottom mussel culture on the sea bottom and benthic habitats: a review. Can. J. Zool. 89, 622–646. doi: 10.1139/z11-037
Milewski I. (2001). “Impacts of salmon aquaculture on the coastal environment: a review,” in Marine aquaculture and the environment: A meeting for stakeholders in the Northeast. Eds. Tlusty M. F., Bengston D. A., Halvorson H. O., Oktay S. D., Pearce J. B., Rheault R. B. Jr. (Cape Cod Press, Falmouth, MA), 166–197.
Mizuta D. D., Kasai A., Ishll K. I., Yamaguchi H., Nakata H. (2014). Effects of artificial upwelling on the environment and reared oyster Crassostrea gigas in Omura Bay, Japan. Bull. Japanese Soc. Fisheries Oceanogr. 78, 13–27.
Moore C. M., Mills M. M., Arrigo K. R., Berman-Frank I., Bopp L., Boyd P. W., et al. (2013). Processes and patterns of oceanic nutrient limitation. Nat. Geosci. 6, pp.701–pp.710. doi: 10.1038/ngeo1765
Morro B., Davidson K., Adams T. P., Falconer L., Holloway M., Dale A., et al. (2022). Offshore aquaculture of finfish: Big expectations at sea. Rev. Aquac. 14, 791–815. doi: 10.1111/raq.12625
Moscicki Z., Dewhurst T., MacNicoll M., Lynn P., Sullivan C., Chambers M., et al. (2022). “Using finite element analysis for the design of a modular offshore macroalgae farm,” in The 9th Conference on Computational Methods in Marine Engineering (Marine 2021), 1 (1), 1–11. doi: 10.2218/marine2021.6855
Moscicki Z., Swift M. R., Dewhurst T., MacNicoll M., Chambers M. D., Tsukrov I., et al. (2024). Design, deployment, and operation of an offshore seaweed cultivation structure. Aquac. Eng. 105, 102413. doi: 10.1016/j.aquaeng.2024.102413
Navarrete I. A., Kim D. Y., Wilcox C., Reed D. C., Ginsburg D. W., Dutton J. M., et al. (2021). Effects of depth-cycling on nutrient uptake and biomass production in the giant kelp Macrocystis pyrifera. Renewable Sustain. Energy Rev. 141. doi: 10.1016/j.rser.2021.110747
Nelson E. J., Robinson S. M. C., Feindel N., Sterling A., Byrne A., Pee Ang K. (2017). Horizontal and vertical distribution of sea lice larvae (Lepeophtheirus salmonis) in and around salmon farms in the Bay of Fundy, Canada. J. Fish Dis. 41, 885–899. doi: 10.1111/jfd.12692
Newell C., Heasman K., Smaal A., Jiang Z. (2021). “Mussel aquaculture,” in Molluscan Shellfish Aquaculture: A practical guide. Ed. Shumway S. E. (5M Printing, London, UK), 107–148.
Oppedal F., Samsing F., Dempster T., Wright D., Bui S., Stein L. (2017). Sea lice infestation levels decrease with deeper ‘snorkel’ barriers in Atlantic salmon sea-cages. Pest Manage. Sci. 73, 1935–1943. doi: 10.1002/ps.2017.73.issue-9
Ortiz Cortes J. (2022). Phytoplankton community responses to ocean artificial upwelling-Prospects of ecosystem service enhancement. Doctoral dissertation, Christian-Albrechts-Universität zu Kiel, Kiel, Germany.
Pereira R., Yarish C. (2008). Mass production of marine macroalgae. In. Encyclopedia Ecol. 18, 2236–2247. doi: 10.1016/B978-008045405-4.00066-5
Prescott L. A., Symonds J. E., Walker S. P., Miller M. R., Semmens J. M., Carter C. G. (2023). Long-term sustained swimming improves swimming performance in Chinook salmon, Oncorhynchus tshawytscha, with and without spinal scoliosis. Aquaculture 573, 739629. doi: 10.1016/j.aquaculture.2023.739629
Quinn T. P., Chamberlin J., Brannon E. L. (2011). Experimental evidence of population-specific marine spatial distributions of chinook salmon, oncorhynchus tshawytscha. Environ. Biol. Fishes 92, 313–322.
Redmond S., Green L., Yarish C., Kim J., Neefus C. (2014). New england seaweed culture handbook-nursery systems (Connecticut Sea Grant CTSG-14-01), 92 pp. Available at: http://seagrant.uconn.edu/publications/aquaculture/handbook.pdf.
Rivas J., Baquedano J. L., Valledor R., Jerez G., Albistur P., Donoso-Bravo A. (2021). CFD-based simulation assessment of an artificial upwelling antibloom system for a salmon farming site. Aquac. Eng. 95, 102182. doi: 10.1016/j.aquaeng.2021.102182
Romuld T. S. (2024). Salmar Aker Ocean – Status and current strategic focus (Bergen, Norway: North Atlantic Seafood Forum 2024).
Scholtens M., Dodds K., Walker S., Clarke S., Tate M., Slattery T., et al. (2023). Opportunities for improving feed efficiency and spinal health in New Zealand farmed Chinook salmon (Oncorhynchus tshawytscha) using genomic information. Aquaculture 563, 738936. doi: 10.1016/j.aquaculture.2022.738936
Sclodnick T., Sutton S., Selby T., Dwyer R., Gace L. (2020). Environmental impacts of brass mesh nets on open ocean aquaculture pens in tropical marine environments. Aquaculture 524, 735266. doi: 10.1016/j.aquaculture.2020.735266
Sievers M., Korsøen Ø., Warren-Mayers F., Oppedal F., Macaulay G., Folkedal O., et al. (2021). Submerged cage aquaculture of marine fish: A review of the biological challenges and opportunities. Rev. Aquac. 14, 106–119. doi: 10.1111/raq.12587
Smaal A. C., van Duren L. (2019). “Bivalve aquaculture carrying capacity: concepts and assessment tools,” in Goods and services of marine bivalves. Ed. Smaal A. C. (Springer, Dordrecht), pp 451–pp 483.
Solvang T., Bale E. S., Broch O. J., Handa A., Alver M. (2021). Automation concepts for industrial-scale production of seaweed. Front. Mar. Sci. 8, 613093. doi: 10.3389/fmars.2021.613093
Stevens C., Plew D., Hartstein N., Fredriksson D. (2008). The physics of open-water shellfish aquaculture. Aquac. Eng. 38, 145–160. doi: 10.1016/j.aquaeng.2008.01.006
St-Gelais A. T., Fredriksson D. W., Dewhurst T., Miller-Hope Z. S., Costa-Pierce B. A., Johndrow K. (2022). Engineering a low-cost kelp aquaculture system for community-scale seaweed farming at nearshore exposed sites via user-focused design process. Front. Sustain. Food Syst. 6, 848035. doi: 10.3389/fsufs.2022.848035
Strand Å., Bailey J., Rydstedt A., James P., Legat J., Sühnel S. (2022). Overview of culture systems for low trophic species (Tromsø: AquaVitae), 62 pages. doi: 10.5281/zenodo.6531530
Swift M. R., Fredriksson D. W., Unrein A., Fullerton B., Patursson O., Baldwin K. (2006). Drag force acting on biofouled net panels. Aquac. Eng. 35, 292–299. doi: 10.1016/j.aquaeng.2006.03.002
Tayler D., Nielsen P., Bak F. (2022). Experiences with a prototype submersible mussel cultivation system (Italy: Innovative solutions in a changing world. EAS conference Rimini).
Troell M., Costa-Pierce B., Stead S., Cottrell R. S., Brugere C., Farmery A. K., et al. (2023). Perspectives on aquaculture’s contribution to the Sustainable Development Goals for improved human and planetary health. J. World Aquac. Soc. 54, 251–342. doi: 10.1111/jwas.12946
Tullberg R. M., Nguyen H. P., Wang C. M. (2022). Review of the status and developments in seaweed farming infrastructure. J. Mar. Sci. Eng. 10, 1447. doi: 10.3390/jmse10101447
Viúdez A., Fernández-Pedrera Balsells M., Rodríguez-Marroyo R. (2016). Artificial upwelling using offshore wind energy for mariculture applications. Sci. Mar. 80S1, 235–248. doi: 10.3989/scimar.04297.06B
Waldrop T., Summerfelt S., Mazik P., Good C. (2017). The effects of swimming exercise and dissolved oxygen on growth performance, fin condition and precocious maturation of early-rearing Atlantic salmon Salmo salar. Aquac. Res. 49, 801–808. doi: 10.1111/are.13511
Wang C. M., Chu Y. I., Baumeister J., Zhang H. (2024). “Recent developments in offshore fish pens,” in Proceedings of the Third World Conference on Floating Solutions. WCFS 2023. Lecture Notes in Civil Engineering, vol. 465 . Eds. Ikoma T., Tabeta S., Lim S. H., Wang C. M. (Springer, Singapore). doi: 10.1007/978-981-97-0495-8_26
Wang C. M., Chu Y., Baumeister J., Zhang H., Jeng D.-S., Abdussamie N. (2022). “Offshore fish farming: challenges and developments in fish pen designs,” in Global Blue Economy. Eds. Nazrul I. M., Bartell S. M. (CRC Press, Boca Raton, FL, USA), 87–128, ISBN: ISBN 9781003184287.
Wang C. M., Chu Y.-I., Baumeister J., Zhang H., Qiao Y.-P., Karampour H., et al. (2023). SeaFisher—A submersible high-density polyethylene offshore fish pen. J. Mar. Sci. Eng. 11, 1795. doi: 10.3390/jmse11091795
Wang C. M., Chu Y. I., Park J. C. (2019). Moving offshore for fish farming. J. Aquac. Mar. Biol. 8, 38–39. doi: 10.15406/jamb.2019.08.00240
Wesley J. J. A. (2020). Investigating the effect of accelerated aging on the mechanical properties of HDPE used in Norwegian fish farms. Master’s thesis, NTNU, Trondheim, Norway.
Wijsman J. W. M., Troost K., Fang J., Roncarati A. (2019). “Global production of marine bivalves. Trends and challenges,” in Goods and services of marine bivalves. Ed. Smaal A. C., Ferreira J. G., Grant J., Petersen J. K., Strand Ø. (Switzerland: Springer Nature), 591.
Wu Y., Duan Y., Wei Y., An D., Liu J. (2022). Application of intelligent and unmanned equipment in aquaculture: A review. Comput. Electron. Agric. 199, 107201. doi: 10.1016/j.compag.2022.107201
Yigit M., Celikkol B., Ozalp B., Bulut M., Dwyer R. L., Yilmaz S., et al. (2018). Comparision of copper alloy mesh with conventional nylon nets in offshore cage farming of gilthead seabream (Sparus aurata). Aquaculture Stud. 18 (1), 57–65. doi: 10.1016/j.compag.2022.107201
Keywords: aquaculture structures, marine bivalves, macroalgae, seaweed, exposed ocean, marine finfish
Citation: Heasman KG, Scott N, Sclodnick T, Chambers M, Costa-Pierce B, Dewhurst T, Isbert W and Buck BH (2024) Variations of aquaculture structures, operations, and maintenance with increasing ocean energy. Front. Aquac. 3:1444186. doi: 10.3389/faquc.2024.1444186
Received: 05 June 2024; Accepted: 16 September 2024;
Published: 31 October 2024.
Edited by:
Charles Weirich, NOAA National Sea Grant Office, United StatesReviewed by:
Damon Howe, University of Tasmania, AustraliaKevin Madley, NOAA Physical Sciences Laboratory, United States
Gonzalo Tampier, Austral University of Chile, Chile
Copyright © 2024 Heasman, Scott, Sclodnick, Chambers, Costa-Pierce, Dewhurst, Isbert and Buck. This is an open-access article distributed under the terms of the Creative Commons Attribution License (CC BY). The use, distribution or reproduction in other forums is permitted, provided the original author(s) and the copyright owner(s) are credited and that the original publication in this journal is cited, in accordance with accepted academic practice. No use, distribution or reproduction is permitted which does not comply with these terms.
*Correspondence: Kevin G. Heasman, a2V2aW4uaGVhc21hbkBjYXd0aHJvbi5vcmcubno=