- 1Department of Safety, Chemistry and Biomedical Laboratory Sciences, Western Norway University of Applied Sciences, Haugesund, Norway
- 2Department of Veterinary Population Medicine, College of Veterinary Medicine, University of Minnesota, St. Paul, MN, United States
Brown algae (Phaeophyceae) dominate intertidal and shallow subtidal areas globally, where larger species form extensive underwater forests. These structurally complex and highly productive habitats enhance local biodiversity and support food webs in coastal areas through secondary production, thereby shaping local oceanography and ecology. Macroalgal aquaculture is an important and growing sector, where approximately 40% of all cultivated algae belong to Phaeophyceae. However, both cultured and natural brown algae have been under increasing pressure due to climate-driven factors, such as ocean warming, eutrophication, and herbivore outbreaks. We conducted a comprehensive literature review on abiotic (temperature, light intensity, and UV radiation, nutrients, water motion, salinity, and substrata and sediment) and biotic (bacteria, viruses, fungi, eukaryotic endophytes and endoparasites, epiphytes, and grazers) stressors and illnesses in marine brown macroalgae, as well as brown algae defense mechanisms, and discuss how these parameters may affect the production of a sustainable crop for the aquaculture industry under future climate change scenarios.
1 Brown algae
Eukaryotes developed about 2.7 billion years ago, but multicellular organisms did not evolve from unicellular eukaryotes until at least 1.7 billion years ago (Cooper, 2000). Multicellularity has evolved numerous times within eukaryotes, and brown algae are among the few groups that have reached complex multicellularity, along with animals, fungi, plants, and amoebozoans (Baldauf, 2003; Bringloe et al., 2020). However, brown algae are distantly related to other eukaryotic groups, and their peculiar metabolic, physiological, cellular, and ecological traits, have turned them into the main components of the marine ecosystem, as well as important ecosystem services for humans (Charrier et al., 2008).
Brown algae (Phaeophyceae) are, therefore, multicellular eukaryotic macroalgae (or seaweeds), which comprise 19 orders with a total number of 2,110 species (Guiry and Guiry, 2018). The older orders started diverging during the Mesozoic Era (≈250 Ma), but most of them, including the most ecologically and economically important ones nowadays, diverged during the so- called “Brown Algal Crown Radiation” (Cretaceous, 145–66 Ma) (Bringloe et al., 2020). The distribution of brown algae is determined by climate and geology over time (Bringloe et al., 2020). They can be found in coastal areas all over our oceans and can be divided into temperate (cold and warm), polar, and tropical groups (Bringloe et al., 2020). Brown algae dominate intertidal and shallow subtidal areas globally, where larger species form extensive marine forests that shape local oceanography and ecology (Bringloe et al., 2020). Kelp forests are probably one of the most well-known and studied canopy-forming brown algae; however, they include only members of the order Laminariales. Fucales, Tilopteridales, and Desmarestiales can also form these underwater forests (Bringloe et al., 2020); therefore, due to the similar functions they perform and for practical reasons, the term kelp forest will be used in here when referring to all the previously mentioned orders of brown algae.
Underwater forests can alter local oceanography and ecology by dampening wave surges, which influence water flow, coastal erosion, sedimentation, benthic productivity (primary and secondary), and recruitment (Duggins et al., 1990). They can also influence interspecific competition among algae because their canopy shades the seafloor, allowing low-light intensity species to grow (Santelices and Ojeda, 1984; Dayton, 1985). Kelp forest architecture provides physical habitat, nursery grounds, and food for organisms above the benthic boundary layer, such as marine mammals, fish, crabs, sea urchins, mollusks, other algae, and epibiota (Mann, 1973; Steneck et al., 2002). Coastal vegetated habitats, such as kelp forests among others (e.g., mangrove forests and seagrass meadows), are known to be one of the most productive ecosystems on Earth (Duarte et al., 2013). Atlantic underwater forests of Laminaria hyperborea have been estimated to be between 110 g C m−2 year−1 and 1,780 g C m−2 year−1, whereas phytoplanktonic production ranges between 100 g C m−2 year−1 and 300 g C m−2 year−1 in temperate coastal regions (Mann, 2000; Smale et al., 2013). Approximately 80% of kelp annual production enters the food web in the form of macroalgal detritus, thereby fueling secondary production and making their carbon available to detritivores and the microbial community, thereby supporting complex food webs in coastal zones (Duggins et al., 1989; Steneck et al., 2002; Wernberg et al., 2019). Macroalgal-derived carbon can travel long distances and sink deep enough to contribute to carbon storage. Even the kelp forest itself can represent an important organic carbon reservoir in the ocean, as refractory carbon compounds are contained within the macroalgal tissues (Wada et al., 2008; Krause-Jensen and Duarte, 2014). For example, kelp forests of Laminaria hyperborea in the North-East Atlantic have been estimated to release particulate carbon at a rate of ≈5.71 Tg C/year (Pessarrodona et al., 2018), and to have carbon sequestration potential of 0.46 million tons of carbon per year (Gundersen et al., 2021).
Such productive habitats not only provide us with a wide range of indirect ecosystem services of great ecological value, but also provide us with direct and intrinsic benefits with social and economic value (Figure 1) (Wernberg et al., 2019).
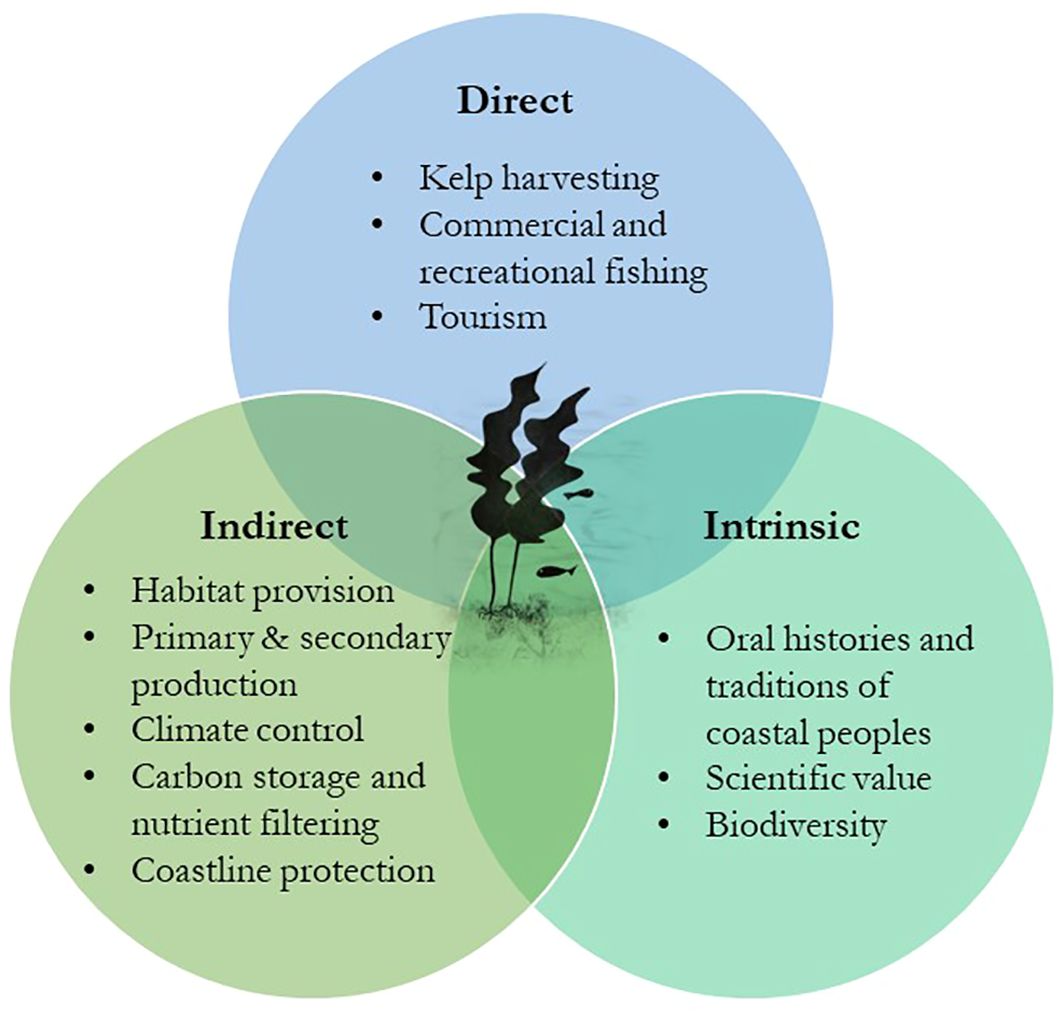
Figure 1 Direct, indirect, and intrinsic ecosystem services of kelp forests. Adapted from the study by Wernberg et al. (2019).
The socioeconomic importance of kelp dates back to some of the oldest times in human history (Erlandson et al., 2007). The consumption of seaweed as food goes back to the Viking age in Norway (Mouritsen et al., 2013), and it remains a traditional food for some coastal communities in Scandinavia today, as well as in Japan, and for the Inuit and Sami peoples (Wernberg et al., 2019). Early humans were aware that seaweed biomass is a rich source of nutrition, even for animals, and their consumption may have helped first colonizers of the American continent to travel along the Pacific rim following the “Kelp Highway” (Erlandson et al., 2007). Moreover, it is thought that the consumption of marine food rich in omega-3 fatty acids and other essential nutrients may have helped the human brain develop in early Homo ancestors (Cornish et al., 2017). Kelps have also remained part of the culture and histories of these coastal peoples, being transmitted through oral tradition, as one of the most important deities in the Maori myth: Hine-nui- (i)-te-po. Hine-nui- (i)-te-po, or the “Great lady of the night,” is considered the queen of the underworld and it has been described to have greenstone eyes, kelp-like hair, teeth like obsidian and a mouth like a barracouta (Perris, 2018).
In addition to direct consumption, kelp forests play a crucial role in coastal fisheries, which benefit local communities not just by providing food but also through tourism, as in the Great Southern Reef in Australia (Wernberg et al., 2019). Growing awareness of the potentially high market value of brown algae has led to an expansion of kelp harvesting and cultivation (Holdt and Kraan, 2011; Kim and Bhatnagar, 2011; Schiener et al., 2014). Today, Brown algae are used in the production of fertilizers and feed for the agriculture and aquaculture industries (Hwang et al., 2013; Correa et al., 2016; Kim et al., 2017), as a current and future food source for an increasing human population (Duarte et al., 2009), for the production of pharmaceuticals (Smit, 2004; Lordan et al., 2011; Batista et al., 2019; Purcell-Meyerink et al., 2021), and for sustainable biofuel production (John et al., 2011; Wargacki et al., 2012; Jung et al., 2013; Song et al., 2015). On this matter, increased environmental awareness and sustainability are pushing towards the use of natural sources for the production of bioactive products (Reboleira et al., 2021). Brown algae polymers such as laminarin, alginate, and fucoidan are excellent structural polysaccharides for fermentation, as well as sugars such as mannuronic and guluronic acids, fucose, and glucose (Reboleira et al., 2021). During fermentation, these carbon molecules are converted into smaller molecules with lower molecular weights (molecular oxidation/reduction), such as acetic, lactic, and other organic acids, through the action of microorganisms, such as Lactobacillus, Leuconostoc, Lactococcus, Streptococcus, and Pediococcus (fermentative gram-positive bacteria) (Monteiro et al., 2021). Ethanol and methane are obtained through seaweed fermentation and represent a sustainable alternative source for the biofuel industry (Reboleira et al., 2021). Fermentation is a highly energy-efficient process compared to other biomass processing technologies that are capable of converting perishable and low-value natural resources into stable and valuable bio-products (Reboleira et al., 2021), as well as making seaweed more palatable, edible, and non-toxic (Bruhn et al., 2019). Therefore, fermentation is a key tool in the discovery, extraction, and processing of novel compounds with industrial applications (Philippsen et al., 2014), and is expected to become more relevant in the future, not just for the biofuel industry, but also for the food, feed, and pharmaceutical industries (Uchida and Miyoshi, 2013).
2 Algal aquaculture
Although wild macroalgal harvesting is still used, the pressure imposed on natural marine ecosystems has led to macroalgal farming, which can occur on land, sea, desert, and even in integrated aquaculture systems (Zhang L. et al., 2022). For example, in 2020, inland aquaculture produced 64,490 tons of fresh weight of algae, whereas coastal and marine aquaculture produced 35,013,089 tons of live weight globally (FAO, 2022). These values represent 0.1% and 51.4% of the total inland, coastal and marine aquaculture, respectively, on a global scale (FAO, 2022). Brown algae exhibit a complex and broad range of cycles, ranging from isomorphic, haploid–diploid (with two morphologically identical generations) to diploid (with only one multicellular generation). Therefore, their cultivation will depend on their life cycle. In kelp species, harvested spores from wild fertile plants can be grown into gametophytes in the laboratory. Sporeling from these microscopic stages (zoospores or gametophytes) are directly seeded onto appropriate substrata such as ropes or nets, which are posteriorly deployed at sea, where the sporophytes will develop further under natural conditions until harvest (Stévant et al., 2017).
To date, the major macroalgal species produced worldwide are Japanese kelp Saccharina japonica, Eucheuma spp., Gracilaria spp., wakame Undaria pinnatifida, nori Porphyra spp., Elkhorn sea moss Kappaphycus alvarezii, Sargassum fusiforme, and Eucheuma denticulatum (FAO, 2022). Among these top macroalgal species, S. japonica, U. pinnatifida, and S. fusiforme are brown algae from the order Laminariales (Saccharina and Undaria species) and Fucales (Sargassum species) representing 35.5%, 8%, and 0.8% of the world’s algal production in 2020, respectively (FAO, 2022).
These kelp species are extensively farmed in East-North Asia (e.g., China, Japan, and Korea) (Figure 2) for human and animal consumption, as well as for their biochemical content (Adams et al., 2008; Song et al., 2015; Kim et al., 2017). Saccharina species are mainly commercially cultivated for alginate, iodine, and mannitol, whereas Undaria species are used for their medicinal nutrients, such as omega-3 fatty acids, essential minerals, and fucoxanthin, and as a food crop (Song et al., 2015). Sargassum species have traditionally been consumed in Asia because of their high nutritional content and are used in traditional Chinese medicine (Song et al., 2015).
The African continent is the world’s second largest producer of algae (Figure 2); however, its production is mainly based on red algae aquaculture, which mainly occurs in Zanzibar (Tanzania), Madagascar, and South Africa (FAO, 2022; Msuya et al., 2022). Ecklonia maxima and Laminaria pallida are two endemic kelp species that dominate the coastal area of South Africa (Troell et al., 2006), and Macrocystis pyrifera is also found in a few small beds in the southwest. The first two brown algae have been collected as beach-casts since the early 1950s to be exported for alginate extraction, and more recently to be used as feed for the abalone industry (Troell et al., 2006; January et al., 2019); however, there has been no commercial aquaculture of kelps in the region to date, except for a pilot aquafarm that is currently being tested (Msuya et al., 2022). There have been many studies on the commercial potential of some brown algae species in Africa, such as Sargassum oligocystum in Kenya for animal and human consumption, and Cystoseira myrica, Padina pavonica, Sargassum fluitans, S. ilifolium, Sargassum sp., Turbinaria triquetra, and T. turbinata for their natural products in Djibouti (Msuya et al., 2022). In Mauritius and Rodrigues, Sargassum aquifolium and Padina gymnospora have also been studied for the development of seaweed-based food items, livestock feed supplements, cosmetics, and bio-fertilizers, and Sargassum spp. have been converted into alginates in Ghana and Nigeria, including the invasive Sargassum muticum in Morocco (Msuya et al., 2022).
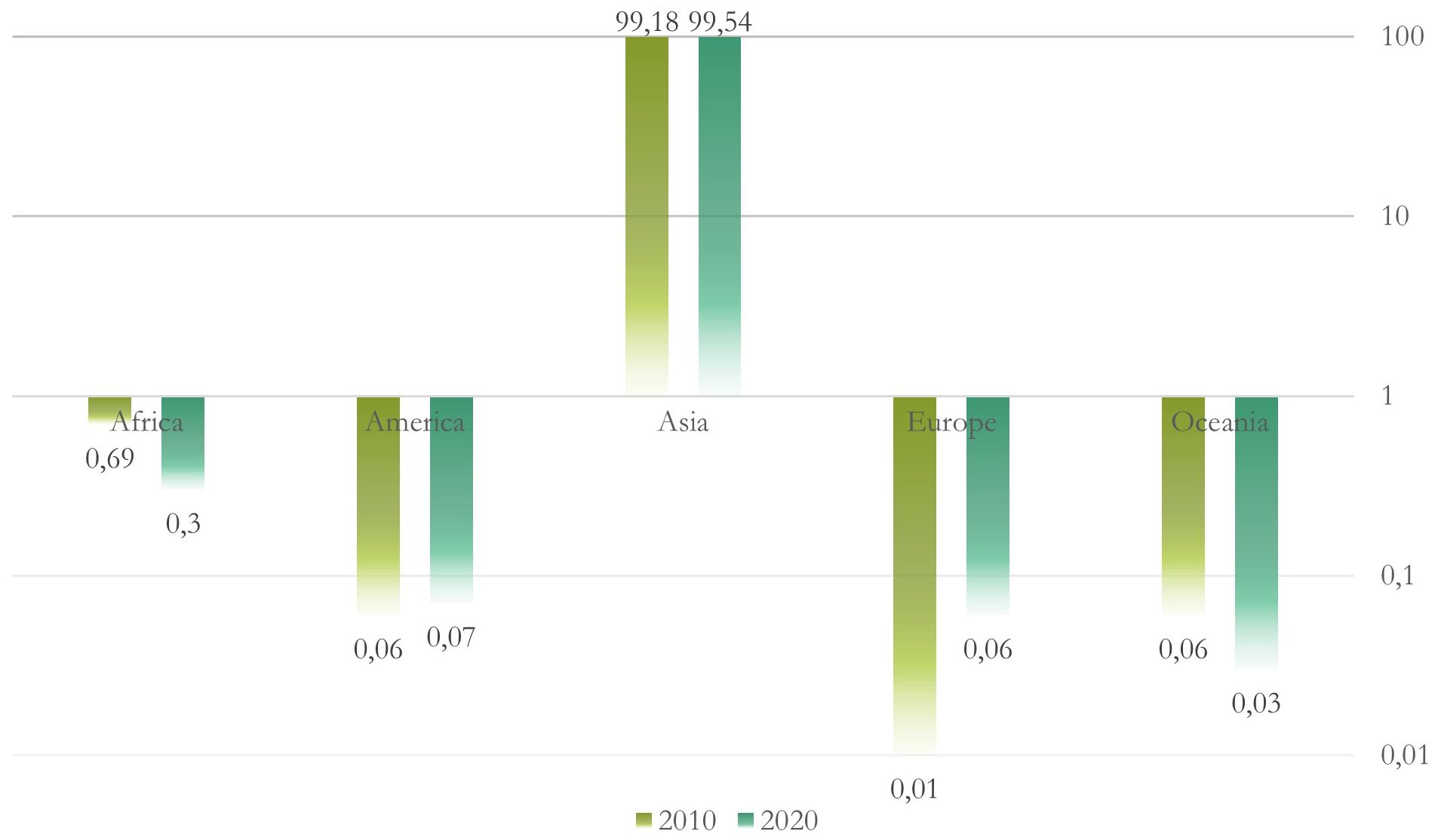
Figure 2 World aquaculture production of algae by continent (in percentage) for 2010 and 2020. Logarithmic scale base 10.
In Western countries (Figure 2), kelp cultivation has become one of the fastest growing industries, and species such as Saccharina latissima and Alaria esculenta have been cultured in the North Atlantic Ocean in the United States (Kim et al., 2017), Canada (Kim et al., 2017), Iceland (Kim et al., 2017), Norway (Broch et al., 2013; Skjermo et al., 2014), Sweden (Kim et al., 2017), Denmark (Marinho et al., 2015), Germany (Buck and Buchholz, 2004), Scotland (Kim et al., 2017), Ireland (Kim et al., 2017), Spain (Peteiro et al., 2016), and Portugal (Kim et al., 2017). In addition, in the eastern Pacific Ocean, M. pyrifera has also grown, especially in Chile (Buschmann et al., 2008; Camus and Buschmann, 2017; Kim et al., 2017) and Alaska (Kim et al., 2017; Stekoll et al., 2021).
Australian algae aquaculture is still under development (Figure 2); however, high species diversity and high endemism are moving forward from the perspective of introducing Australian macroalgae into food and animal feed markets (Phillips, 2001; Biancacci et al., 2022). For example, Sargassum rugosum and Durvillaea species are found only in the southern hemisphere, such as the Australian and New Zealand coastlines (Hurd et al., 2004; January et al., 2019). In New Zealand, ecological harvesting impacts and alginate content of different brown algae (e.g., Ecklonia radiata, Papenfussiella lutea, Myriogloia intestinalis, Cystophora, Undaria, and Durvillaea species, Xiphophora chondrophylla, Hormosira banksii, Lessonia variegata, M. pyrifera, Carpophyllum maschalocarpum, Scytosiphon lomentaria, Marginariella boryana, and Splachnidium rugosum) have also been studied recently (Hurd et al., 2004).
3 Brown algae stressors
Brown algae are influenced by a range of abiotic (e.g., changes in light attenuation, sedimentation, nutrients, salinity, and temperature) and biotic (e.g., epiphytes and grazers) factors (Dayton, 1985) (Figure 3). Most of these factors are not easy to measure because they are interconnected, but in general, adverse environmental conditions generate oxidative stress in marine algae; meaning the excessive production and accumulation of reactive oxygen species (ROS) such as hydrogen peroxide (H2O2), singlet oxygen (1O2), superoxide radical (O2.−) and hydroxyl radical (OH.) (Dring, 2005; Lesser, 2006). Abiotic stressors tend to result in a gradual and continued build-up of ROS because photosynthesis is inhibited and excess energy results in the formation of singlet oxygen, whereas the response to other stressors, like infection or mechanical stress, can be more rapid and intense, but short‐lived production of ROS, or “oxidative burst” (attributed to activation of NADPH oxidases in the plasma membrane). If the algal capacity to remove these reduced oxygen intermediates is insufficient (normally achieved through a high cellular content of antioxidant compounds or a high activity of antioxidant enzymes), cellular lipids, proteins, and DNA can be damaged (Dring, 2005; Lesser, 2006).
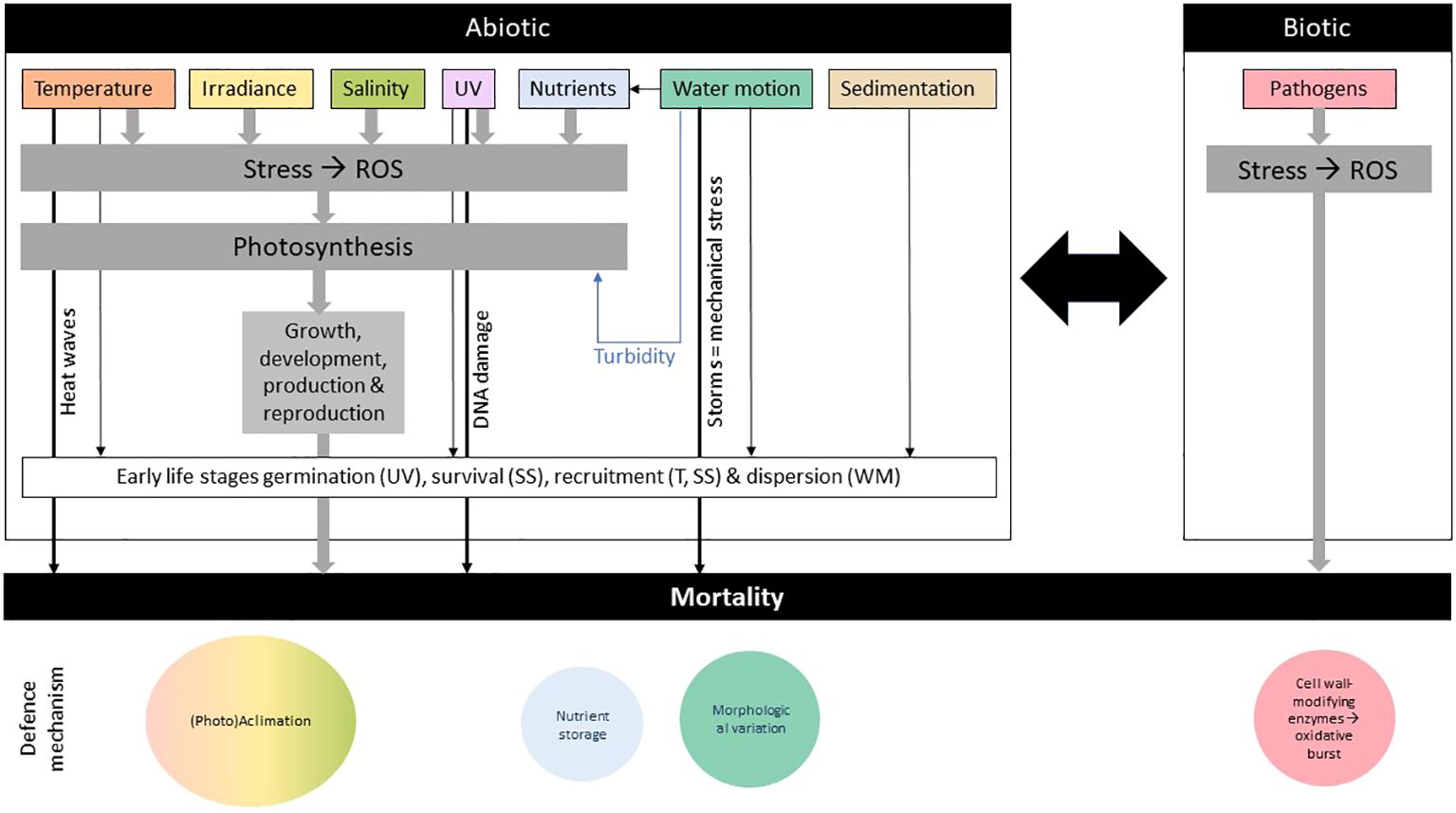
Figure 3 Main biotic and abiotic algal stressors and their respective defense mechanisms. The early life stages refer to spores, gametophytes, and juvenile sporophytes. SS, Sedimentation and Substrate; T, Temperature; WM, Water Motion effect.
During the last century, kelp forests have been in decline worldwide most likely due to different processes, such as heatwaves, storms, or herbivore outbreaks (Steneck et al., 2002), which have become increasingly intense over the last two decades as a result of anthropogenic pressure (Steneck et al., 2002; Eggert et al., 2010; Krumhansl et al., 2016; Wernberg et al., 2019). However, climate change has different effects in different areas of the world, and species may be affected differently, depending on their location. For example, in recent years, the Mexican Caribbean coast has received massive atypical influxes of pelagic Sargassum spp (Chávez et al., 2020). These blooms have been attributed to an increase in the nutrient load in the Atlantic Basin via river discharges due to deforestation and other land-use changes upstream, as well as increased sea temperatures, among others (Chávez et al., 2020). While Sargassum’s growth does not suffer adverse effects in this case, the negative impacts associated with these influxes include mortality of nearshore benthic flora and fauna, beach erosion, and pollution, which directly affect ecosystem services such as tourism and involve high management costs (Chávez et al., 2020).
Intensive algal aquaculture itself can be another source of disease outbreaks (Gachon et al., 2010), which can not only be harmful for the aquaculture industry but also for the natural marine ecosystem (Valero et al., 2017). For example, Pyropia farms in Korea experienced an approximately US $1.6 million loss (~24.5% of total sales) during 2012–2013, and about US $1.1 million (~10.7% of total sales), due to disease (Kim et al., 2014). In the Philippines, the disease caused a 15% loss in the production of another red algae, K. alvarezii, between 2011 and 2013, corresponding to a loss of over US$ 310 million (Cook et al., 2016). Within brown algae, up to 42% of sporophyte length was lost daily during 2017–2018 in cultivated S. japonica in China due to disease (Wang et al., 2021).
3.1 Abiotic stressors
There are a few known physiological diseases in brown algae (Table 1) caused by one or more of the environmental factors described below, including environmental pollution (Wang et al., 2014).
3.1.1 Temperature
Globally, sea surface temperatures have increased at a rate of >0.1°C per decade since the mid-20th century (Pörtner et al., 2022). Increased temperatures can directly and indirectly affect brown algae, especially those found at lower latitudes, where temperature plays a strong role in shaping their geographical distribution (Steneck et al., 2002; Wernberg et al., 2019). Species shifts have already been observed (Smale and Moore, 2017), and future projections have consistently shown significant reductions and northward migrations of canopy-forming species (e.g., L. digitata and L. hyperborea) in the southern limits (Breeman, 1990; Eggert et al., 2010; Raybaud et al., 2013; Assis et al., 2016). Although kelp forests are considered to be highly resilient (Krumhansl et al., 2016; Lind and Konar, 2017), and some populations and species may tolerate or adapt better to higher thermal ranges (because of the presence of alleles or genotypes beneficial under future climatic conditions within genetically diverse species) (Smale and Moore, 2017; Vranken et al., 2021), their capacity to respond to physical and biological disturbances might be negatively affected by the reduced ecological performance of kelp recruits (Wernberg et al., 2010). Moreover, marine heatwaves can lead to direct mortality (Wernberg et al., 2019) because they expose species and ecosystems to environmental conditions beyond their tolerance and acclimation limits (Pörtner et al., 2022), replacing kelp forests with other warm-tolerant or even invasive species (Arafeh-Dalmau et al., 2019). Since the 1980s, marine heat waves have become more frequent, intense, and last longer; therefore, global warming will most likely be accompanied by diversity loss and kelp forest removal (Wernberg et al., 2019; Pörtner et al., 2022).
3.1.2 Light intensity and UV radiation
The amount and quality of irradiance are important factors for many physical and biological processes during all life stages of algae (Dayton, 1985), affecting algal growth through its impact on photosynthesis (Stockenreiter et al., 2013). The growth rate of algae is maximal at saturation intensity and decreases with both rising and falling light intensityies (Kaur et al., 2022). Light affects the distribution of marine algae because irradiance in marine ecosystems is highly variable in both space (water depth and latitude) and time (day and season) (Han and Kain (Jones), 1996). Photoadaptation (a change in genotype that occurs over many generations in response to a change in light intensity), however, can help algae survive through these changes in light intensity (Kaur et al., 2022). Changes in pigment types and quantities, growth rate, dark respiration rate, and essential fatty acid supply could be some of the processes that occur during photoadaptation (Fábregas et al., 2004).
It has been shown that the algal establishment stage (gametophytes or young sporophytes) affects the performance of the adult. Light demands can also vary according to the algal life stage; for example, it has been determined that the minimum photon irradiance allowing growth in different kelp species is between 1 µmol m−2 s−1 and 2 µmol m−2 s−1, whereas the growth saturated at 20 µmol m−2 s−1–30 µmol m−2 s−1 of continuous photon irradiance (Han and Kain (Jones), 1996).
Exposure to ultraviolet (UV) light (wavelengths 215 nm–400 nm) has harmful effects on algae, especially UV-B wavebands, affecting their growth and development, and causing oxidative stress (Pessoa, 2012). UV-B radiation directly damages DNA molecules, whereas UV-A radiation induces indirect damage by generating reactive oxygen and hydroxyl radicals (Kaur et al., 2022). It has been shown that early life stages of many macroalgae (meiospore, gametophyte, and juvenile sporophyte) are sensitive to UV (Roleda et al., 2010). For example, an hour of exposure to UV can reduce the germination of meiospores as well as the photosynthetic efficiency of gametophytes and juvenile sporophytes in both L. digitata and S. latissima (Dring et al., 1996).
3.1.3 Nutrients
All algae require macro- (e.g., N, P, and C) and micronutrients (e.g., Fe, Zn, Cu, Mn, and Mo), as well as vitamins (vitamin B12, thiamine, and biotin) to support their growth (Harrison and Hurd, 2001). Among these, nitrate and phosphate are extremely important, and the optimum growth ratio for macroalgae is 30N:1P, with a range from 10:1 to 80:1 (Atkinson and Smith, 1983). Since inorganic nutrient concentrations in surface waters differ according to geographic region and vary throughout the year, they can become limiting (Harrison and Hurd, 2001). Therefore, some kelps, such as Laminaria and Macrocystis, can store nutrients to maintain their growth even during nutrient depletion periods, particularly during summer (Dayton, 1985; Young et al., 2009).
3.1.4 Water motion
According to Gaylord et al. (2012), fluid-dynamic transport and mixing processes affect birth, death, immigration, and emigration rates in kelp forests, and can modulate broader community interactions. Thus, water motion can influence spore transport and settlement, algal population connectivity, and the impact of sedimentation and scour, as well as affect light by moving canopies (Dayton, 1985; Gaylord et al., 2012). Breaking waves and high velocities during storms can also lead to mortality, resulting in the destruction of kelp forests (Seymour et al., 1989), and wave exposure can influence the prevalence of viral infections within Ectocarpales (McKeown et al., 2018). Brown macroalgae can, however, adapt to water motion through morphological variation, such as E. radiata, which has been shown to exhibit wide, thin thalli in sheltered habitats and narrow, thick thalli with thick stipes in exposed habitats (Fowler-Walker et al., 2006).
Most importantly, water motion also influences the macroalgae’s nutrient uptake. High flow rates enhance uptake rates by accelerating nutrient transport through the diffusion boundary layer adjacent to the plant surface, which is faster in turbulent rather than laminar boundary layers (Gerard, 1982). Stagnant or slow-moving waters not only reduce the nutrient uptake rate but also limit the carbon dioxide supply, leading to reduced growth rates (Gerard and Mann, 1979; Kerrison et al., 2015). Therefore, current velocity is positively correlated to photosynthetic and nutrient uptake rates, until these metabolic processes become saturated (velocities of approximately 0.1 m s−1) (Wheeler, 1980; Hurd, 2000; Kerrison et al., 2015).
3.1.5 Salinity
Salinity influences osmotic pressure within algal cells, and both hyper- and hypo-salinity conditions can affect various physiological processes in marine algae (Kerrison et al., 2015; Kaur et al., 2022). Most brown algae, such as Laminaria, can balance salinity stress by increasing mannitol concentrations (Reed et al., 1985); however, under extreme salinity stress, turgor pressure, ion distribution, and organic solutes in the cell can be disrupted, resulting in cell oxidative damage (Tropin et al., 2003; Kaur et al., 2022).
Most macroalgae thrive at salinities between 33 psu and 35 psu, although some algal species are more tolerant than others (Kerrison et al., 2015). For example, when different brown algae collected in Kongsfjorden (Spitsbergen) were exposed to salinities ranging from 5 psu to 60 psu, Fucus distichus was not affected, whereas A. esculenta, S. latissimi, and Laminaria solidungula experienced loss of pigments (bleaching) or even high mortality under hyposaline conditions (Karsten, 2007). In the same study, L. digitata and Saccharina dermatodea survived all salinities, but showed reduced photosynthetic activities in the lowest and highest salt treatments (Karsten, 2007). It has been shown that salinity acclimation mechanisms involve the upregulation and downregulation of genes related to photosynthesis and energy production, cytoskeleton and membrane transport, and stress responses (Rugiu et al., 2020).
3.1.6 Substrata and sedimentation
Most brown algae grow on rocky substrates, especially mature kelp populations; however, some can also grow on sandy bottoms. All of these substrates can be unstable, promoting sediment deposition, burial/smothering, and water turbidity, negatively affecting kelp plants (Dayton, 1985; Araujo et al., 2012). Sediment deposition can interfere with the attachment of microscopic stages of seaweeds, as demonstrated for various kelps, such as M. pyrifera, notably reducing the probability of survival (Devinny and Volse, 1978). Moreover, burial/smothering may also reduce recruitment rates by significantly lowering the survival and germination rates of successfully attached spores as well as by reducing growth and impairing the regeneration ability of adults (Devinny and Volse, 1978; Chapman et al., 2002).
Turbidity can affect photosynthesis in brown algae, and some species such as E. radiata can present higher photosynthetic pigment levels and photosynthetic efficiency at turbid sites, compared to high-light sites, demonstrating a clear capacity to photoacclimate to a degrading light environment (Blain and Shears, 2019).
3.2 Biotic stressors
Macroalgae are colonized by diverse micro- and macroorganisms, that live and grow on (epiphytes) or inside (endophytes), such as bacteria, fungi, viruses, oomycetes, or filamentous algae of all three macroalgal lineages (Dayton, 1985; Bartsch et al., 2008; Gachon et al., 2010; Vallet et al., 2018). While some of these interactions can be neutral or beneficial for the host, others can be detrimental; these are the ones we are going to focus on here.
Brown algae have different defense mechanisms against pathogenic organisms. The first barrier of defense is composed of innate receptors that perceive microbe-associated molecular patterns (MAMPs) (Weinberger, 2007). MAMPs are non-pathogen specific but can, for example, perceive bacterial lipopolysaccharides and lipoteichoic acids (Weinberger, 2007). In addition, macroalgae also possess pathogen-induced molecular patterns (PIMPs) that perceive breakdown products of their own cell walls that are released when they are being attacked by pathogens, such as alginate, in the case of kelps (Weinberger, 2007).
The response after recognition of PIMPs or MAMPs is normally an oxidative burst, but an upregulated expression of defense-related proteins has also been observed in brown algae (Weinberger, 2007). Signaling cascades of oxylipins and oxidized polyunsaturated fatty acids are activated after the perception of external molecular signals, activating the transcription of these compounds (Weinberger, 2007), such as polyphenol oxidase in S. japonica (Lili et al., 2004) and aromatic compounds in L. digitata (Küpper et al., 2002). These secondary metabolites function as primary defenses against herbivores, competitors (allelopathy), pathogens, and fouling organisms (epiphytes) (Jennings and Steinberg, 1997). The most common secondary metabolites in brown algae are phlorotannins, dehydropolymers of phloroglucinol (1,3,5-trihydroxybenzene) with antifouling and anti-herbivory activities, which also serve as UV protectants and antioxidants (Jennings and Steinberg, 1997; Gómez and Huovinen, 2020).
Macroalgae also count with hypersensitive responses such as programmed cell death (apoptosis), in which infected and adjacent cells are sacrificed to control the spread of the disease by the activation of key enzymes such as nucleases and caspases (Weinberger, 2007). Lastly, many macroalgae, including kelp, have haloperoxidases in their cell walls that produce hypohalus acids and halocarbons, such as bromoform, which increases algal protection by interfering with bacterial quorum sensing and biofilm formation (Weinberger, 2007).
3.2.1 Bacteria
Macroalgae are normally colonized by diverse microorganisms such as bacteria. The phyla Proteobacteria, Bacteroidetes, and Firmicutes are among the most abundant bacterial communities found in brown algae (de Mesquita et al., 2019). Bacteria specifically associate with particular macroalgal species and even with certain parts of the algal body, finding a protected microniche from which they can also uptake nutrients released by the alga, such as organic carbon (Goecke et al., 2010). In turn, bacteria can contribute to algal growth and development and even prevent secondary biofouling or pathogen invasion (Goecke et al., 2010; de Mesquita et al., 2019). However, not all algal-bacteria interactions are beneficial, and some bacterial communities can compromise algal tissue and photosynthetic capability, induce diseases, and compromise the algae’s health (Goecke et al., 2010; de Mesquita et al., 2019). With such a long tradition of seaweed cultivation, Asian countries have extensive experience with many of these bacterial diseases (Wang et al., 2014; Ward et al., 2019), such as the Green Rot Disease, one of the major infections affecting the Chinese aquaculture industry of S. japonica, leading to a complete failure of hatchery operations when it breaks out (Li et al., 2020) (Table 2). However, some of these bacteria are believed to be secondary colonizers (de Mesquita et al., 2019). Therefore, while some bacteria can be found on brown algae as commensals, stressful conditions or macroalgal infections may turn them into saprophytes or decomposers (de Mesquita et al., 2019). For example, white rot disease (Table 1) is considered to be a physiological disease, but opportunistic alginic acid-decomposing bacteria might also take advantage of the situation (Wang et al., 2014).
Macroalgae influence bacterial metabolism and quorum sensing, and produce antibiotic compounds that inhibit settlement, growth, and biofilm formation by bacteria (Goecke et al., 2010).
3.2.2 Viruses
The first observations of brown algae infected with viruses were Ectocarpus plants collected around Europe, which presented abnormal sporangia (Sauvageau, 1896). Electron microscopy allowed us to observe infected organs full of densely packed hexagonal virus-like particles in different brown algae species, confirming viral disease (Müller et al., 1990, Müller et al., 1998). The virus type species is Ectocarpus siliculosus virus (ESV-1), which belongs to the genus Phaeovirus, and in turn belongs to the Phycodnaviridae family within the giant virus phylum Nucleocytoviricota (Koonin et al., 2020). Phycodnaviruses comprise large icosahedral viruses with dsDNA genomes ranging from 160 kb to 560 kb (Wilson and Allen, 2011), infecting numerous taxa of algae (Dunigan et al., 2006). However, only a few seaweed viruses have been fully characterized. Phaeoviruses are the only giant viruses known to infect multicellular algae (Müller and Parodi, 1993; Müller et al., 1996a, Müller et al., 1996b). Seven species were grouped within four different families within Ectocarpales, and eight species belonged to three different families of the order Laminariales, commonly known as kelp (McKeown et al., 2017, McKeown et al., 2018) (Table 3).
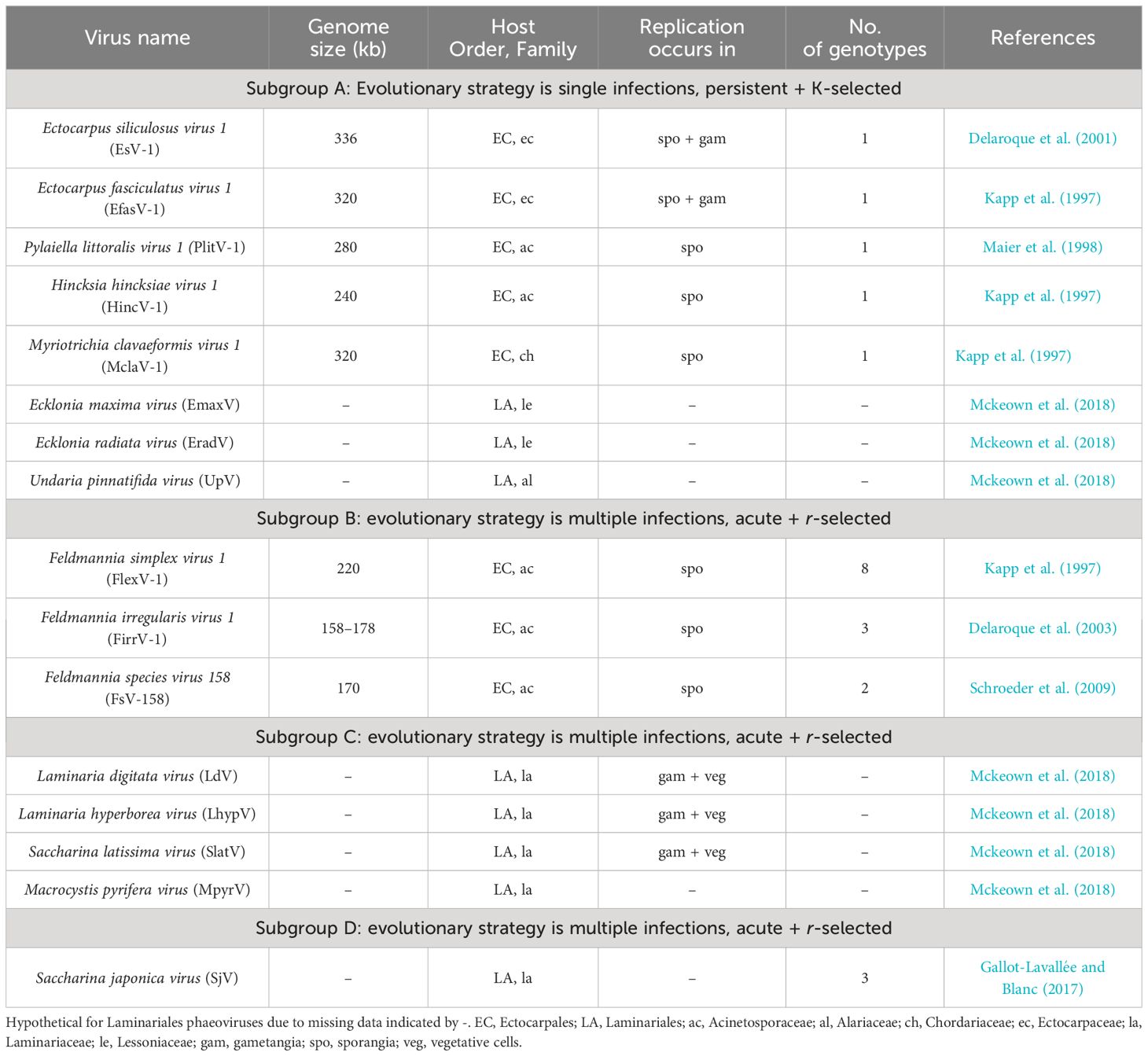
Table 3 Features of Phaeovirus subgroups (McKeown, Brown, n.d.; McKeown et al., 2018).
Viral particles infecting multicellular brown algae enter their wall-less life cycle stages, motile spores, or gamete forms (Müller and Parodi, 1993; Maier et al., 1998, Maier et al., 2002). Upon entry, the genome of the virus can integrate within the host genome without killing it and persist as a provirus (Meints et al., 2008). After the host zoid has settled into a suitable substrate, a copy of the virus is transmitted through mitosis to all the cells of the developing alga (Müller et al., 1990; Maier et al., 2002; Schroeder, 2011). Adult sporophytes or gametophytes carrying the provirus can appear without any visible signals of survival or growth impairment (Müller and Stache, 1992), and with the ability to produce viable spores or gametes that contain the proviral genome (Cock et al., 2010). However, deformed reproductive organs can appear after viral induction, which severely reduces algal reproduction (Grimsley et al., 2012; Schroeder and C., 2015). The persistent life strategy represents a stable evolutionary co-existence of virus and host that is unique within nucleocytoviruses (Schroeder and C., 2015), where r-strategies (phaeoviral subgroups B, C, and D) are more commonly found than K-like ones (phaeoviral subgroup A) (Müller et al., 1998; Schroeder and C., 2015). Despite being widely distributed and highly prevalent (Sengco et al., 1996; Müller et al., 2000; Schroeder, 2011; McKeown et al., 2018; Ruiz Martínez et al., 2023; Schroeder et al., 2023), Phaeoviruses, as well as other potential viruses infecting brown algae, remain to be discovered (Ruiz Martínez et al., 2023).
3.2.3 Fungi and fungus-like organisms
Macroalgae harbor a large diversity of filamentous fungi that colonize their inner tissues without causing any apparent damage or disease (Vallet et al., 2018). Obligate fungal endosymbionts such as Stigmidium ascophylli were first described in the brown algae Ascophyllum nodosum and Pelvetia canaliculata in the 1900s (Cotton, 1907), and they have been suggested to protect their algal host from desiccation, while obtaining nutrients in exchange (Garbary, 2005). However, many fungal endophytes are opportunistic pathogens that can produce toxic metabolites that are detrimental to their hosts (Vallet et al., 2018) (Table 4). Like other parasites, they can affect the host’s growth, fecundity, and mortality, regulating host population dynamics and influencing the community structure (Marcogliese, 2004). In this section, parasitic marine fungi and oomycetes are reviewed together since the last ones are normally referred to as “fungus-like” organisms and it is considered that they have similar ecological roles as true zoosporic fungi, even if they do not belong to the kingdom Fungi (Hassett et al., 2019). However, Oomycota are globally distributed zoosporic heterokonts that phylogenetically branch within the Stramenopila–Alveolata–Rhizaria superkingdom (Burki and Keeling, 2014).
Brown algae defend themselves against fungi through oxidative bursts and chemical defenses (Amsler and Fairhead, 2005), such as E. siliculosus, which induces oxidative stress and halogen metabolism in response to Eurychasma dicksonii infection (Strittmatter et al., 2016), and Lobophora variegate, which produces the cyclic lactone lobophorolide, which presents high antifungal activity (Kubanek et al., 2003).
3.2.4 Algae endophytes and other eukaryotic endoparasites
Many algae from all three macroalgal lineages, especially filamentous brown algae of the order Ectocarpales, are known to live as kelp epi-endophytes, such as Laminariocolax or Laminarionema, producing galls and severe thallus deformations, leading to biomass loss and decay (Bringloe et al., 2021) (Table 5). Since certain species are found both inside and outside their algal hosts, a clear distinction between epi- and endophytes is not always possible (Peters et al., 2003). For example, some algal endophytes may grow entirely within their host, but form reproductive structures on the host’s surface (Peters and Schaffelke, 1996). Both endophytic and epiphytic algae (reviewed in Okida, 1960) are listed in Table 5. Endophyte zoospores are produced in plurilocular sporangia and can attach to and penetrate the healthy host surface without needing an open wound in the host for successful invasion of the host (Eggert et al., 2010). Disruption of host tissues and the fact that some endophytes can also obtain nutrients from them affect host survival and reproductive potential (Correa and McLachlan, 1992; Faugeron et al., 2000).
3.2.5 Epiphytes
Epiphytes attach directly or live in tubes that are attached to the algae’s thallus or fronds. Among these fouling organisms are hydroids, bryozoans, bivalve mollusks, tubiculous amphipods, tubiculous polychaetes, and colonial tunicates (Dixon et al., 1981) (Table 6). Algae carrying epiphytes can experience mechanical problems on the thallus and blade deformities due to their extra weight, as well as surface friction and drag, chemical changes at the host–epiphyte interface, and competition for light and nutrients (Dixon et al., 1981; Correa and McLachlan, 1992). Moreover, collateral effects can also occur, such as increased susceptibility to secondary pathogens, accidental grazing by carnivores or herbivores eating epiphytes, or increased blade loss during storms (Dixon et al., 1981; Correa and McLachlan, 1992; James et al., 2020). Consequently, algal growth and production can be impaired, affecting the seaweed industry for farmed brown algal species.
Field observations from different parts of the world, such as the North-East Atlantic or the western Baltic Sea, have documented massive rates of infection prevalence by endophytic brown algae (Eggert et al., 2010).
Macroalgae can combine chemical responses synergistically with physical responses, such as epithelial sloughing, and possibly gelatinous and microtopographically designed surfaces, to defend themselves from epiphytes (da Gama et al., 2014). Epithelial sloughing or periodic shedding of surface tissue has been reported in some brown algae, such as A. nodosum, Halidrys siliquosa, E. menziesii, Myagropsis myagroides, and Sargassum spp (da Gama et al., 2014).
3.2.6 Grazers
A wide variety of herbivores, including sea urchins, gastropods, crustaceans, and fishes, consume live kelp sporophytes, gametophytes, and their spores (Dayton, 1985; Duffy and Hay, 1990) (Table 7). Therefore, grazing can alter recruitment, demography, and seaweed abundance (Leonard, 1994). Grazers can remove large amounts of seaweed biomass, especially sea urchins, provoking trophic cascades that can affect the entire marine ecosystem (Pinnegar et al., 2000; Iken, 2012). For example, kelp forests and their associated invertebrates and fishes can disappear into an urchin-barren state, where few seaweeds, except for encrusting corallines, prevail (Steneck et al., 2002). Even if smaller invertebrates do not exert such pressure on macroalgae, they can lead to increased mortality by tunneling into the holdfast, causing the sporophyte to become dislodged (Lobban, 1978).
Algal defense strategies against herbivores have been divided into non-coexistence and coexistence strategies, with the latter encompassing structural and chemical defenses (Lubchenco and Gaines, 1981; Duffy and Hay, 1990; Iken, 2012) (Figure 4).
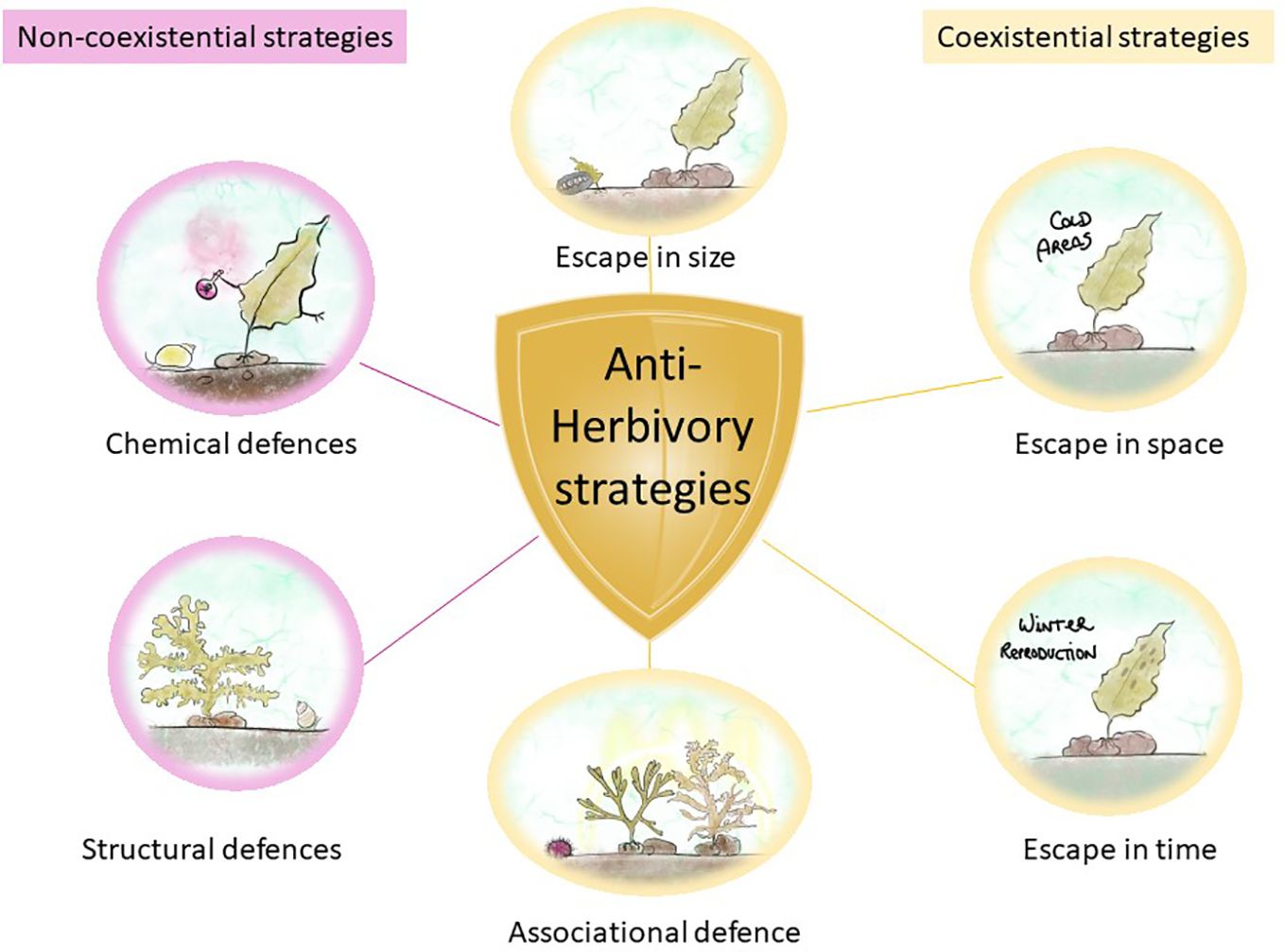
Figure 4 Algal defense strategies against herbivores. Non-coexistential strategies in magenta and coexistential strategies in yellow.
In non-coexistence strategies, the encounter of seaweeds with herbivores is minimized, and this is accomplished by escaping in time, space, and size and by associational defense (Iken, 2012). Escaping in time and space is a self-explanatory strategy that occurs when seaweeds escape grazing by completing important life history phases (e.g., initial growth or reproduction) in places or moments where herbivores are not present or active (Iken, 2012). Escape in size occurs when seaweeds outgrow the sporeling stage, becoming less susceptible to grazing, such as the chiton Katharina tunicate, which only grazes on sporelings but not on adult kelp Hedophyllum sessile (Markel and DeWreede, 1998). Associational defense occurs when macroalgae associate with other organisms that provide them with chemical or structural protection from grazers, such as the decreased consumption of Sargassum furcatum by sea urchin Lytechinus variegatus when protected by the chemicals of Dictyota sp (Pereira et al., 2010). These strategies are the result of chance or co-evolutionary processes (Iken, 2012).
Coexistence strategies lower the herbivore’s attraction to a seaweed because of the specific structural or chemical traits of the alga (Iken, 2012). There are two types of defenses: structural and chemical. The first are also known as mechanical or morphological defenses, and they involve the inclusion of calcium carbonate into algal cells (Paul and Hay, 1986). For example, F. distichus produces adventitious branches after mechanical damage to the plant apices, preventing further grazing by the snails Littorina sitkana and L. scutulata (even if a chemical defense may also be present at the same time) (Van Alstyne, 1989). Chemical defenses are secondary metabolites that function as herbivore deterrents and mostly reduce the palatability or nutritional quality of the seaweed or can also reduce the fitness and survivorship of the herbivore through toxic effects (Iken, 2012), as explained in the previous section. For example, the snail Lacuna vincta avoids grazing on the intercalary meristem of Laminaria longicruris due to its high phenolic content (Amsler and Fairhead, 2005).
4 Disease breading grounds
Marine ecosystems are currently threatened by global climate change. These climate-driven changes, especially ocean warming and acidification, alter species distributions and biodiversity and are expected to have profound ecological, social, and economic implications (Wernberg et al., 2016) (Figure 5). An alarming decrease in the density and biomass of canopy-forming kelps has been reported worldwide (Dayton et al., 1999); for instance, S. latissima beds were strongly reduced and partly replaced by filamentous turf algae along the southwest and Skagerak coasts of Norway, and between 2002 and 2018, approximately 80% of the populations disappeared (Moy and Christie, 2012). Temperature sets distributional limits for kelps and other macroalgae, and latitudinal range shifts in marine macroalgae have already been observed (Lima et al., 2007). Kelp decline and community structure changes have been linked to climate change and marine heatwaves (MHWs) in many studies overall (Wernberg et al., 2010; Raybaud et al., 2013; Arafeh-Dalmau et al., 2019). Filbee-Dexter et al. (2020) experimentally demonstrated a relationship between MHWs and high kelp loss in Southern Norway, where mortality was strongly linked to the physiological changes that kelp experiences when temperature goes beyond their lethal thresholds, that is, tissue damage, increased dislodgment, and reduced photosynthetic and reproductive performance (Filbee-Dexter et al., 2020).
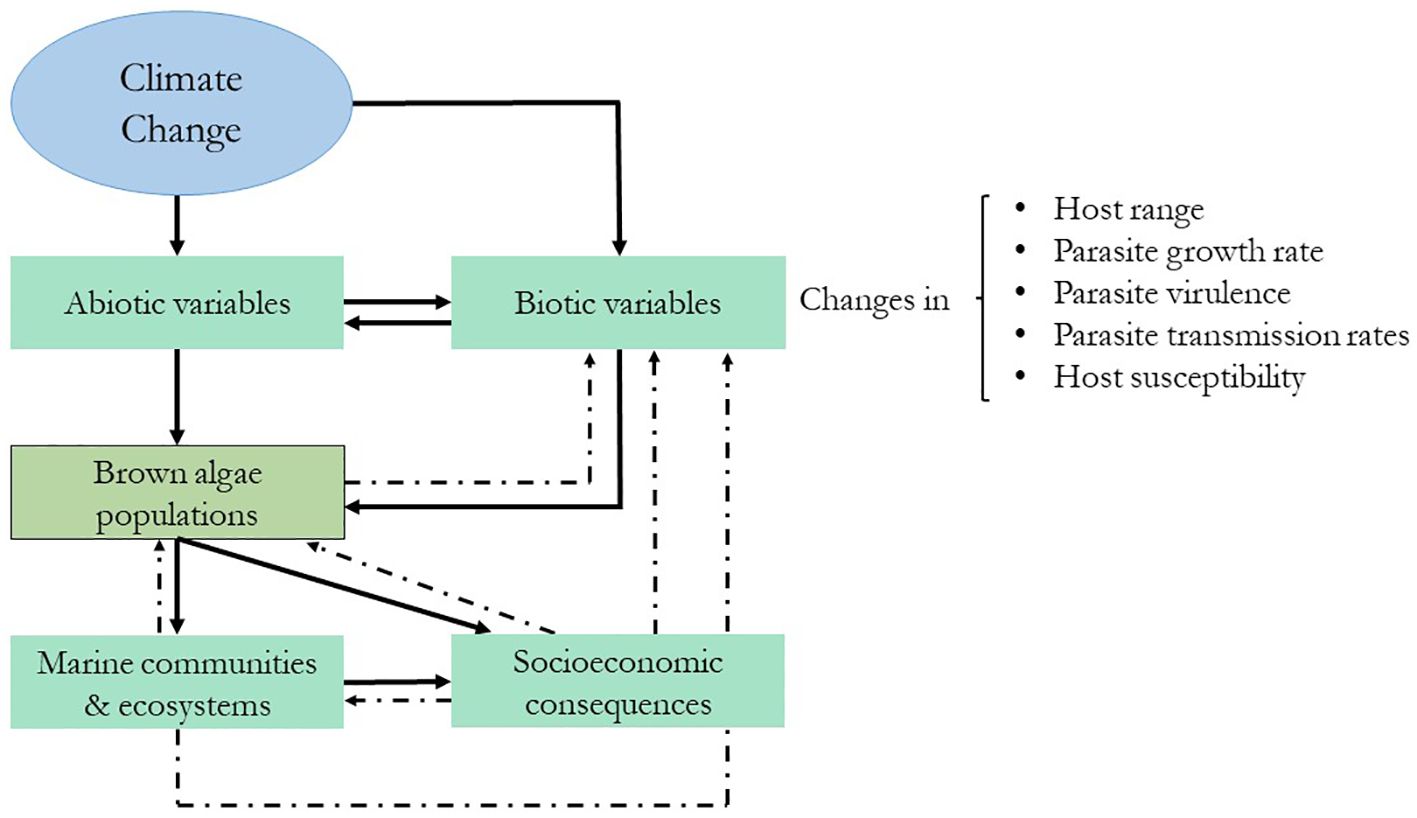
Figure 5 Schematic representation of the expected cascading effects of climate change on brown algae and their stressors. The dashed lines represent feedback effects. Modified from Marcogliese (2008).
Abiotic changes can also affect pre-established biotic interactions between algae and their competitors, colonizers, grazers, and pathogens (Eggert et al., 2010) (Figure 5). Based on terrestrial ecosystems, Harvell et al. (2002) proposed that host–pathogen interactions will become more frequent and intense in the future and that they will be affected by (1) more rapid pathogen development owing to increased growth rates of the pathogen at higher temperatures, (2) increased winter survival of the pathogen, and (3) increased host susceptibility at higher temperatures. The effects of climate change on marine algae–pathogen interactions are poorly understood; however, herbivore/epiphyte studies predict cascading effects on primary producers (Harley et al., 2012) (Figure 5). For example, warmer sea surface temperatures are associated with increases in some herbivore populations and a concomitant decline in certain algal species (Hart and Scheibling, 1988; Ling, 2008). However, it is difficult to make general predictions, since these changes will not be homogeneous or affect all organisms equally. Indirect effects of climate change can also alter these expectations; for example, acidification can negatively affect calcified herbivores, and changes in nutrient availability can also alter algal palatability (Harley et al., 2012). Epiphytic bryozoans in eastern Canada have also increased in number due to sea warming events, leading to drastic reductions in the percentage cover of Saccharina longicruris beds (Saunders et al., 2010). Field observations from different parts of the world have documented massive rates of infection prevalence by endophytes (Eggert et al., 2010), viruses (Ruiz Martínez et al., 2023), and bacteria (Harvell et al., 1999).
With the worldwide intensification of seaweed aquaculture and climate change-driven changes, an increase in the prevalence of diseases and pests is expected (Ward et al., 2019). Disease outbreaks can also occur if infected algae are introduced accidentally, which is a potential treatment for natural algal populations, not to mention the economic impact of diseases on the seaweed industry (Sugumaran et al., 2022) (Figure 5).
5 A sustainable future for aquaculture
Without a coordinated global effort to support seaweed research, the investigation and knowledge transfer of technical capacities, protection of wild seaweed populations, and promotion of environmental, gender-responsive, and socially inclusive approaches to upscaling the seaweed industry are not possible. To address this issue, the GlobalSeaweedSTAR team published an international policy brief with a list of eight recommendations for improving the resilience and sustainability of the industry (Cottier-Cook et al., 2021). The key to sustainable aquaculture practices relies on biosecurity and mitigation measures (based on the identification of diseases and pests, and reviewed in [Behera et al., 2022) and (Sugumaran et al., 2022)] that should be further (1) developed into clear international policies and regulations. However, without proper knowledge and training regarding pathogen diversity, host range, and interactions with their hosts, it will be more difficult to predict the potential ecological consequences of climate change on algae–pathogen interactions in the future. This requires the (2) Development of gender-responsive regional and national capacity-building initiatives. It is extremely important to (3) develop regional and national seed stocks and biosecuring nurseries, and (4) maintain the genetic diversity of wild stocks by preventing the introduction of non-indigenous species and encouraging the development of indigenous strains/varieties for commercial cultivation. (5) Advanced assessment tools should be used to perform risk-based analyses of management options at multiple scales and to enable economic diversification. (6) The integration of seaweed with other fed-aquaculture species and other maritime activities should be incentivized. At the same time, (7) channeling support for long-term investment in promoting the beneficial aspects of the industry may help farmers implement the previously cited practices that will help build resilience to the impacts of climate change. The final recommendation is to (8) establish a network of Regional Seaweed Research Networks, to obtain the necessary scientific knowledge in which all previous studies will be based. As researchers, we have this responsibility, and this review intends to consolidate all previous efforts made on the topic while keeping our focus on future progress.
Author contributions
ER: Conceptualization, Funding acquisition, Visualization, Writing – original draft, Writing – review & editing. DS: Supervision, Writing – review & editing. GT: Writing – review & editing. IH: Funding acquisition, Supervision, Writing – review & editing.
Funding
The author(s) declare financial support was received for the research, authorship, and/or publication of this article. This research was funded by the Research Council of Norway, through project “ViralICE: Viral diversity and Interactions in a Changing Environment on kelp,” grant number 314108.
Conflict of interest
The authors declare that the research was conducted in the absence of any commercial or financial relationships that could be construed as a potential conflict of interest.
Publisher’s note
All claims expressed in this article are solely those of the authors and do not necessarily represent those of their affiliated organizations, or those of the publisher, the editors and the reviewers. Any product that may be evaluated in this article, or claim that may be made by its manufacturer, is not guaranteed or endorsed by the publisher.
References
Adams J. M., Gallagher J. A., Donnison I. S. (2008). Fermentation study on Saccharina latissima for bioethanol production considering variable pre-treatments. J. Appl. Phycology 21, 569–574. doi: 10.1007/s10811-008-9384-7
Akiyama K. (1977a). On the Olpidiopsis disease of juveniles Undaria pinnatifida in field culture. Bull. Tohoku Reg. Fish Res. Lab. 37, 43–49.
Akiyama K. (1977b). Preliminary report on Streblonema disease in Undaria. Bull. Tohoku Regional Fisheries Res. Lab. 37, 39–41.
Amsler C. D., Fairhead V. A. B. T.-A. (2005). Defensive and sensory chemical ecology of brown algae. Incorporating Advances in Plant Pathology 43, 1–91. doi: 10.1016/S0065–2296(05)43001–3
Apt K. (1988). Etiology and development of hyperplasia induced by Streblonerna sp (Phaeophyta) on members of the Laminariales (Phaeophyta). J. Phycol 24, 28–34. doi: 10.1111/j.1529-8817.1988.tb04453.x
Arafeh-Dalmau N., Montaño-Moctezuma G., Martinez J. A., Beas-Luna R., Schoeman D. S., Torres-Moye G. (2019). Extreme marine heatwaves alter kelp forest community near its equatorward distribution limit. Front. Mar. Sci. 6. doi: 10.3389/fmars.2019.00499
Araujo R., Arenas F., Åberg P., Sousa-Pinto I., Serrão E. A. (2012). The role of disturbance in differential regulation of co-occurring brown algae species: Interactive effects of sediment deposition, abrasion and grazing on algae recruits. J. Exp. Mar. Biol. Ecol. 422–423, 1–8. doi: 10.1016/j.jembe.2012.04.006
Assis J., Lucas A. V., Bárbara I., Serrão E.Á. (2016). Future climate change is predicted to shift long-term persistence zones in the cold-temperate kelp Laminaria hyperborea. Mar. Environ. Res. 113, 174–182. doi: 10.1016/j.marenvres.2015.11.005
Atkinson M. J., Smith S. V. (1983). C:N:P ratios of benthic marine plants. Limnology Oceanography 28, 568–574. doi: 10.4319/lo.1983.28.3.0568
Baldauf S. (2003). The deep roots of eukaryotes. Science 300, 1703–1706. doi: 10.1126/science.1085544
Bartsch I., Wiencke C., Bischof K., Buchholz C. M., Buck B. H., Eggert A., et al. (2008). The genus Laminaria sensu lato: recent insights and developments. Eur. J. Phycology 43, 1–86. doi: 10.1080/09670260701711376
Batista P. S. P., de Morais A. M. M. B., Pintado M. M. E., de Morais R. M. S. C. (2019). Alginate: Pharmaceutical and Medical Applications BT - Extracellular Sugar-Based Biopolymers Matrices. Eds. Cohen E., Merzendorfer H. (New York City: Springer International Publishing), 649–691. doi: 10.1007/978–3-030–12919-4_16
Beattie D. T., Lachnit T., Dinsdale E. A., Thomas T., Steinberg P. D. (2018). Novel ssDNA Viruses Detected in the Virome of Bleached, Habitat-Forming Kelp Ecklonia radiata. Front. Mar. Sci. 4. doi: 10.3389/fmars.2017.00441
Behera D. P., Ingle K. N., Mathew D. E., Dhimmar A., Sahastrabudhe H., Sahu S. K., et al. (2022). Epiphytism, diseases and grazing in seaweed aquaculture: A comprehensive review. Rev. Aquaculture 14, 1345–1370. doi: 10.1111/raq.12653
Bernard M. S., Strittmatter M., Murúa P., Heesch S., Cho G. Y., Leblanc C., et al. (2019). Diversity, biogeography and host specificity of kelp endophytes with a focus on the genera Laminarionema and Laminariocolax (Ectocarpales, Phaeophyceae). Eur. J. Phycology 54, 39–51. doi: 10.1080/09670262.2018.1502816
Bernstein B. B., Jung N. (1979). Selective pressures and coevolution in a kelp canopy community in Southern California. Ecol. Monogr. 49, 335–355. doi: 10.2307/1942488
Biancacci C., Sanderson J. C., Evans B., Callahan D. L., Francis D. S., Skrzypczyk V. M., et al. (2022). Nutritional composition and heavy metal profiling of Australian kelps cultured in proximity to salmon and mussel farms. Algal Res. 64, 102672. doi: 10.1016/j.algal.2022.102672
Blain C. O., Shears N. T. (2019). Seasonal and spatial variation in photosynthetic response of the kelp Ecklonia radiata across a turbidity gradient. Photosynthesis Res. 140, 21–38. doi: 10.1007/s11120-019-00636-7
Breeman A. M. (1990). Expected effects of changing seawater temperatures on the geographic distribution of seaweed species. Expected Effects Climatic Change Mar. Coast. Ecosystems. Developments Hydrobiology 57, 69–76. doi: 10.1007/978–94-009–2003-3_9
Breen P. A., Mann K. H. (1976). Destructive grazing of kelp by sea urchins in Eastern Canada. J. Fisheries Res. Board Canada 33, 1278–1283. doi: 10.1139/f76-164
Bringloe T. T., Sauermann R., Krause-Jensen D., Olesen B., Klimova A., Klochkova T. A., et al. (2021). High-throughput sequencing of the kelp Alaria (Phaeophyceae) reveals epi-endobiotic associations, including a likely phaeophycean parasite. Eur. J. Phycology 56, 494–504. doi: 10.1080/09670262.2021.1882704
Bringloe T. T., Starko S., Wade R. M., Vieira C., Kawai H., de Clerck O., et al. (2020). Phylogeny and evolution of the brown algae. Crit. Rev. Plant Sci. 39, 281–321. doi: 10.1080/07352689.2020.1787679/SUPPL_FILE/BPTS_A_1787679_SM9001.DOCX
Broch O. J., Ellingsen I. H., Forbord S., Wang X., Volent Z., Alver M. O., et al. (2013). Modelling the cultivation and bioremediation potential of the kelp Saccharina latissima in close proximity to an exposed salmon farm in Norway. Aquaculture Environ. Interact. 4, 187–206. doi: 10.3354/aei00080
Bruhn A., Brynning G., Johansen A., Lindegaard M. S., Sveigaard H. H., Aarup B., et al. (2019). Fermentation of sugar kelp (Saccharina latissima)—effects on sensory properties, and content of minerals and metals. J. Appl. Phycology 31, 3175–3187. doi: 10.1007/s10811-019-01827-4
Buck B. H., Buchholz C. M. (2004). The offshore-ring: A new system design for the open ocean aquaculture of macroalgae. J. Appl. Phycology 16, 355–368. doi: 10.1023/B:JAPH.0000047947.96231.ea
Buschmann A. H., Hernández-González M. D. C., Varela D. (2008). Seaweed future cultivation in Chile: Perspectives and challenges. Int. J. Environ. pollut. 33, 432–456. doi: 10.1504/IJEP.2008.020571
Camus C., Buschmann A. H. (2017). Macrocystis pyrifera aquafarming: Production optimization of rope-seeded juvenile sporophytes. Aquaculture 468, 107–114. doi: 10.1016/j.aquaculture.2016.10.010
Chapman A. S., Albrecht A. S., Fletcher R. L. (2002). Differential effects of sediments on survival and growth of Fucus Serratus embryos (Fucales, Phaeophyceae). J. Phycology 38, 894–903. doi: 10.1046/j.1529-8817.2002.t01-1-02025.x
Charrier B., Coelho S. M., le Bail A., Tonon T., Michel G., Potin P., et al. (2008). Development and physiology of the brown alga Ectocarpus siliculosus: two centuries of research. New Phytol. 177, 319–332. doi: 10.1111/j.1469-8137.2007.02304.x
Chávez V., Uribe-Martínez A., Cuevas E., Rodríguez-Martínez R. E., van Tussenbroek B. I., Francisco V., et al. (2020). Massive influx of pelagic sargassum spp. on the coasts of the mexican caribbean 2014–2020: challenges and opportunities. Water 12 (10), 2908. doi: 10.3390/w12102908
Chen D., Lin G., Shen S. (1979). Studies on alginic acid decomposing bacteria I. Action of alginic acid decomposing bacteria and alginase on Laminaria japonica. Oceanol Limnol Sin. 10, 329–333.
Chess J. R. (1993). Effects of the Stipe-Boring Amphipod Peramphithoe stypotrupetes (Corophioidea: Ampithoidae) and Grazing Gastropods on the Kelp Laminaria setchellii. J. Crustacean Biol. 13, 638–646. doi: 10.2307/1549094
Cock J. M., Sterck L., Rouzé P., Scornet D., Allen A. E., Amoutzias G., et al. (2010). The Ectocarpus genome and the independent evolution of multicellularity in brown algae. Nature 465, 617–621. doi: 10.1038/nature09016
Coles J. W. (1958). Nematodes parasitic on sea weeds of the genera ascophyllum and fucus. J. Mar. Biol. Assoc. United Kingdom 37, 145–155. doi: 10.1017/S0025315400014892
Conlan K. E., Chess J. R. (1992). Phylogeny and ecology of a kelp-boring amphipod, peramphithoe stypotrupetes, new species (Corophioidea: Ampithoidae). J Crustac Biol. 12, 410–422. doi: 10.2307/1549035
Cook E.-J., Nagabhatla N., Badis Y., Campbell M., Chopin T., et al. (2016). Safeguarding the future of the global seaweed aquaculture industry. United Nations Univ., 1–12.
Cooper G. M. (2000). The Cell: A Molecular Approach. 2nd edition (Sunderland (MA: Sinauer Associates). Available at: https://www.ncbi.nlm.nih.gov/books/NBK9841/.
Cornish M. L., Critchley A. T., Mouritsen O. G. (2017). Consumption of seaweeds and the human brain. J. Appl. Phycology 29, 2377–2398. doi: 10.1007/s10811-016-1049-3
Correa T., Gutiérrez A., Flores R., Buschmann A. H., Cornejo P., Bucarey C. (2016). Production and economic assessment of giant kelp Macrocystis pyrifera cultivation for abalone feed in the south of Chile. Aquaculture Res. 47, 698–707. doi: 10.1111/are.2016.47.issue-3
Correa M. J. A., Martínez Mosqueira E. A. (2017) Factors Associated With Host Specificity in Sporocladopsis Novae-Zelandiae (Chlorophyta). Available online at: https://repositorio.uc.cl/handle/11534/20315.
Correa J. A., McLachlan J. L. (1992). Endophytic algae of Chondrus crispus (Rhodophyta). IV. Effects on the host following infections by Acrochaete operculata and A. heteroclada (Chlorophyta). Mar. Ecol. Prog. Ser. 81, 73–87. doi: 10.3354/meps081073
Cottier-Cook E., Nagabhatla N., Asri A., Beveridge M., Bianchi P., Bolton J., et al. (2021). Ensuring the sustainable future of the rapidly expanding global seaweed aquaculture industry – a vision. United Nations University Institute on Comparative Regional Integration Studies and Scottish Association for Marine Science Policy Brief. ISBN 978-92-. Oban, Argyll, Scotland.
Cotton A. D. (1907). Notes on marine pyrenomycetes. Trans. Br. Mycological Soc. 3, 92–99. doi: 10.1016/S0007-1536(07)80023-4
da Gama B. A. P., Plouguerné E., Pereira R. C. (2014). “Chapter fourteen - the antifouling defence mechanisms of marine macroalgae,” in Sea Plants, vol. 71 . Ed. Bourgougnon B. R. (Amsterdam: Elsevier Academic Press), 413–440. doi: 10.1016/B978–0-12–408062–1.00014–7
Dangeard P. (1934). Sur la présence à Roscoff d’uneChytridiale parasite des Ectocarpacées, l’Eurychasmadicksonii(Wright) Magnus. Ann. Protist. 4, 69–73.
Dayton P. (1985). Ecology of kelp communities. Annu. Rev. Ecol. Systematics 16, 215–245. doi: 10.1146/annurev.es.16.110185.001243
Dayton P., Tegner M., Edwards P., Riser K. (1999). Temporal and spatial scales of kelp demography: The role of oceanographic climate. Ecol. Monogr. 69, 219–250. doi: 10.1890/0012-9615(1999)069[0219:TASSOK]2.0.CO;2
Dean T. A., Schroeter S. C., Dixon J. D. (1984). Effects of grazing by two species of sea urchins (Strongylocentrotus franciscanus and Lytechinus anamesus) on recruitment and survival of two species of kelp (Macrocystis pyrifera and Pterygophora californica). Mar. Biol. 78, 301–313. doi: 10.1007/BF00393016
Delaroque N., Boland W., Müller D. G., Knippers R. (2003). Comparisons of two large phaeoviral genomes and evolutionary implications. J. Mol. Evol. 57, 613–622. doi: 10.1007/s00239-003-2501-y
Delaroque N., Müller D. G., Bothe G., Pohl T., Knippers R., Boland W., et al. (2001). The complete DNA sequence of the Ectocarpus siliculosus virus EsV-1 genome. Virology 287, 112–132. doi: 10.1006/viro.2001.1028
de Mesquita M. M. F., Crapez M. A. C., Teixeira V. L., Cavalcanti D. N. (2019). Potential interactions bacteria-brown algae. J. Appl. Phycology 31, 867–883. doi: 10.1007/s10811-018-1573-4
Devinny J. S., Volse L. A. (1978). Effects of sediments on the development of Macrocystis pyrifera gametophytes. Mar. Biol. 48, 343–348. doi: 10.1007/BF00391638
Dixon J., Schroeter S. C., Kastendiek J. (1981). Effects of the encrusting bryozoan, membranipora membranacea, on the loss of blades and fronds by the giant kelp, macrocystis pyrifera (laminariales). J. Phycology 17, 341–345. doi: 10.1111/j.1529-8817.1981.tb00860.x
Dring M. (2005). “Stress resistance and disease resistance in seaweeds: the role of reactive oxygen metabolism,” in Incorporating Advances in Plant Pathology, vol. 43. (Amsterdam: Elsevier Academic Press), 175–207. doi: 10.1016/S0065–2296(05)43004–9
Dring M. J., Makarov V., SchosChina E., Lorenz M., Lüning K. (1996). Influence of ultraviolet-radiation on chlorophyll fluorescence and growth in different life-history stages of three species of Laminaria (phaeophyta). Mar. Biol. 126, 183–191. doi: 10.1007/BF00347443
Duarte C. M., Holmer M., Olsen Y., Soto D., Marbà N., Guiu J., et al. (2009). Will the oceans help feed humanity? BioScience 59, 967–976. doi: 10.1525/bio.2009.59.11.8
Duarte C. M., Losada I. J., Hendriks I. E., Mazarrasa I., Marbà N. (2013). The role of coastal plant communities for climate change mitigation and adaptation. Nat. Climate Change 3, 961–968. doi: 10.1038/nclimate1970
Duffy J. E. (1990). Amphipods on seaweeds: partners or pests? Oecologia 83, 267–276. doi: 10.1007/BF00317764
Duffy J. E., Hay M. E. (1990). Seaweed adaptations to herbivory. BioScience 40, 368–375. doi: 10.2307/1311214
Duggins D. O., Eckman J. E., Sewell A. T. (1990). Ecology of understory kelp environments. II. Effects of kelps on recruitment of benthic invertebrates. J. Exp. Mar. Biol. Ecol. 143, 27–45. doi: 10.1016/0022-0981(90)90109-P
Duggins D. O., Simenstad C. A., Estes J. A. (1989). Magnification of secondary production by kelp detritus in coastal marine ecosystems. Science 245, 170–173. doi: 10.1126/science.245.4914.170
Dunigan D. D., Fitzgerald L. A., Van Etten J. L. (2006). Phycodnaviruses: A peek at genetic diversity. Virus Res. 117, 119–132. doi: 10.1016/j.virusres.2006.01.024
Eggert A., Peters A. F., Küpper F. C. (2010). “The potential impact of climate change on endophyte infections in kelp sporophytes,” in Seaweeds and their Role in Globally Changing Environments. Cellular Origin, Life in Extreme Habitats and Astrobiology, vol. 15 . Eds. Seckbach A., Einav J., Israel R. (Springer, Dordrecht), 139–154. doi: 10.1007/978–90-481–8569-6_9
Ellertsdóttir E., Peters A. (1997). High prevalence of infection by endophytic brown algae in populations of Laminaria spp. (Phaeophyceae). Mar. Ecol. Prog. Ser. 146, 135–143. doi: 10.3354/meps146135
Endo H., Sato Y., Kaneko K., Takahashi D., Nagasawa K., Okumura Y., et al. (2021). Ocean warming combined with nutrient enrichment increases the risk of herbivory during cultivation of the marine macroalga Undaria Pinnatifida. ICES J. Mar. Sci. 78, 402–409. doi: 10.1093/icesjms/fsaa069
Erlandson J. M., Graham M. H., Bourque B. J., Corbett D., Estes J. A., Steneck R. S. (2007). The kelp highway hypothesis: marine ecology, the coastal migration theory, and the peopling of the Americas. J. Island Coast. Archaeology 2, 161–174. doi: 10.1080/15564890701628612
Ezura Y., Yamamoto H., Kimura T. (1988). Isolation of a marine bacterium that produces red-spots on the culture bed of makonbu Laminaria japonica cultivation. Nippon Suisan Gakkaishi 54, 665–672. doi: 10.2331/suisan.54.665
Fábregas J., Maseda A., Domínguez A., Otero A. (2004). The cell composition of Nannochloropsis sp. changes under different irradiances in semicontinuous culture. World J. Microbiol. Biotechnol. 20, 31–35. doi: 10.1023/B:WIBI.0000013288.67536.ed
FAO (2022). “The state of world fisheries and aquaculture 2022. Towards blue transformation,” in The State of World Fisheries and Aquaculture 2022 (Rome, Italy: FAO). doi: 10.4060/CC0461EN
Farlow W. G. (1889). On some new or imperfectly known algae of the United States I. Bull. Torrey Botanical Club 16, 1–12. doi: 10.2307/2477165
Faugeron S., Martínez E. A., Sánchez P. A., Correa J. A. (2000). Infectious diseases in Mazzaella laminarioides (Rhodophyta): estimating the effect of infections on host reproductive potential. Dis. Aquat. Organisms 42, 143–148. doi: 10.3354/dao042143
Filbee-Dexter K., Wernberg T., Grace S. P., Thormar J., Fredriksen S., Narvaez C. N., et al. (2020). Marine heatwaves and the collapse of marginal North Atlantic kelp forests. Sci. Rep. 10, 13388. doi: 10.1038/s41598–020-70273-x
Fowler-Walker M. J., Wernberg T., Connell S. D. (2006). Differences in kelp morphology between wave sheltered and exposed localities: morphologically plastic or fixed traits? Mar. Biol. 148, 755–767. doi: 10.1007/s00227-005-0125-z
Gachon C. M. M., Sime-Ngando T., Strittmatter M., Chambouvet A., Kim G. H. (2010). Algal diseases: spotlight on a black box. Trends Plant Sci. 15, 633–640. doi: 10.1016/j.tplants.2010.08.005
Gallot-Lavallée L., Blanc G. (2017). A glimpse of nucleo-cytoplasmic large DNA virus biodiversity through the eukaryotic genomics window. Viruses 9, 17. doi: 10.3390/v9010017
Garbary R. J. D. (2005). Ascophyllum and Its Symbionts. VIII. Interactions Among Ascophyllum nodosum (Phaeophyceae), Mycophycias ascophylli (Ascomycetes) and Elachista fucicola (Phaeophyceae). Algae 20, 363–368. doi: 10.4490/ALGAE.2005.20.4.353
Gaylord B., Nickols K. J., Jurgens L. (2012). Roles of transport and mixing processes in kelp forest ecology. J. Exp. Biol. 215, 997–1007. doi: 10.1242/jeb.059824
Gerard V. A. (1982). In situ water motion and nutrient uptake by the giant kelp Macrocystis pyrifera. Mar. Biol. 69, 51–54. doi: 10.1007/BF00396960
Gerard V. A., Mann K. H. (1979). Growth and production of Laminaria Longicrucis (Phaeophyta) populations exposed to different intensities of water movement. J. Phycology 15, 33–41. doi: 10.1111/j.1529-8817.1979.tb02958.x
Goecke F., Labes A., Wiese J. (2010). Chemical interactions between marine macroalgae and bacteria. Mar. Ecol. Prog. Ser. 409, 267–299. doi: 10.3354/meps08607
Gómez I., Huovinen P. (2020). Brown Algal Phlorotannins: An Overview of Their Functional Roles BT - Antarctic Seaweeds: Diversity, Adaptation and Ecosystem Services. Eds. Gómez I., Huovinen P. (New York City, U.S.: Springer International Publishing), 365–388. doi: 10.1007/978–3-030–39448-6_18
Gonzalez M. A., Goff L. J. (1989). The red algal epiphytes Microcladia Coulteri and M. Californica (rhodophyceae, ceramiaceae). II. Basiphyte specificity. J. Phycology 25, 558–567. doi: 10.1111/j.1529-8817.1989.tb00262.x
Grimsley N. H., Thomas R., Kegel J. U., Jacquet S., Moreau H., Desdevises Y. (2012). “Chapter nine - genomics of algal host-virus interactions,” in Advances in Botanical Research, vol. 64 . Ed. Piganeau G. (Amsterdam, The Netherlands: Elsevier Academic Press). doi: 10.1016/B978–0-12–391499–6.00009–8
Guiry M. D., Guiry G. M. (2018). AlgaeBase. World-wide electronic publication (Galway: National University of Ireland). Available at: http://www.algaebase.org.
Gundersen H., Rinde E., Bekkby T., Hancke K., Gitmark J. K., Christie H. (2021). Variation in population structure and standing stocks of kelp along multiple environmental gradients and implications for ecosystem services. Front. Mar. Sci. 8. doi: 10.3389/fmars.2021.578629
Hagerman L. (1966). The macro- and microfauna associated with Fucus serratus L., with some ecological remarks. Ophelia 3, 1–43. doi: 10.1080/00785326.1966.10409631
Han T., Kain (Jones) J. M. (1996). Effect of photon irradiance and photoperiod on young sporophytes of four species of the Laminariales. Eur. J. Phycology 31, 233–240. doi: 10.1080/09670269600651431
Harley C. D. G., Anderson K. M., Demes K. W., Jorve J. P., Kordas R. L., Coyle T. A., et al. (2012). EFFECTS OF CLIMATE CHANGE ON GLOBAL SEAWEED COMMUNITIES. J. Phycology 48, 1064–1078. doi: 10.1111/j.1529-8817.2012.01224.x
Harrison P., Hurd C. (2001). Nutrient physiology of seaweeds: Application of concepts to aquaculture. Cahiers Biologie Mar. 42, 71–82.
Hart M. W., Scheibling R. E. (1988). Heat waves, baby booms, and the destruction of kelp beds by sea urchins. Mar. Biol. 99, 167–176. doi: 10.1007/BF00391978
Harvell C. D., Kim K., Burkholder J. M., Colwell R. R., Epstein P. R., Grimes D. J., et al. (1999). Emerging marine diseases–climate links and anthropogenic factors. Science 285, 1505–1510. doi: 10.1126/science.285.5433.1505
Harvell C. D., Mitchell C. E., Ward J. R., Altizer S., Dobson A. P., Ostfeld R. S., et al. (2002). Climate warming and disease risks for terrestrial and marine biota. Sci. (New York N.Y.) 296, 2158–2162. doi: 10.1126/science.1063699
Hassett B. T., Thines M., Buaya A., Ploch S., Gradinger R. (2019). A glimpse into the biogeography, seasonality, and ecological functions of arctic marine Oomycota. IMA Fungus 10, 6. doi: 10.1186/s43008-019-0006-6
Holdt S. L., Kraan S. (2011). Bioactive compounds in seaweed: Functional food applications and legislation. J. Appl. Phycology 23, 543–597. doi: 10.1007/s10811-010-9632-5
Hurd C. L. (2000). Water motion, marine macroalgal physiology, and production. J. Phycology 36, 453–472. doi: 10.1046/j.1529-8817.2000.99139.x
Hurd C. L., Nelson W. A., Falshaw R., Neill K. F. (2004). History, current status and future of marine macroalgal research in New Zealand: Taxonomy, ecology, physiology and human uses. Phycological Res. 52, 80–106. doi: 10.1111/j.1440-1835.2004.tb00318.x
Hwang E. K., Gong Y. G., Hwang I.-K., Park E.-J., Park C. S. (2013). Cultivation of the two perennial brown algae Ecklonia cava and E. stolonifera for abalone feeds in Korea. J. Appl. Phycology 25, 825–829. doi: 10.1007/s10811-012-9941-y
Iken K. (2012). Grazers on Benthic Seaweeds BT - Seaweed Biology: Novel Insights into Ecophysiology, Ecology and Utilization. Eds. Wiencke C., Bischof K. (Berlin, Heidelberg: Springer), 157–175. doi: 10.1007/978–3-642–28451-9_8
Ishikawa Y., Saga N. (1989). “The diseases of economically valuable seaweeds and pathology in Japan,” in Current topics in marine biotechnology. Eds. Miyachi S., Karube I., Ishida Y. (Fuji Technology Press Ltd, Tokyo), 215–218.
James A. K., English C. J., Nidzieko N. J., Carlson C. A., Wilbanks E. G. (2020). Giant kelp microbiome altered in the presence of epiphytes. Limnology Oceanography Lett. 5, 354–362. doi: 10.1002/lol2.10157
January G. G., Naidoo R. K., Kirby-McCullough B., Bauer R. (2019). Assessing methodologies for fucoidan extraction from South African brown algae. Algal Res. 40, 101517. doi: 10.1016/j.algal.2019.101517
Jennings J. G., Steinberg P. D. (1997). Phlorotannins versus other factors affecting epiphyte abundance on the kelp Ecklonia radiata. Oecologia 109, 461–473. doi: 10.1007/s004420050106
Jiang J., Ma Y., Zhang Z., Xu H. (1997). The histopathological study on “green decay diseases” of Undaria pinnatifida in Dalian. J. Dalian Fisheries Univ. 12, 7–12.
John R. P., Anisha G. S., Nampoothiri K. M., Pandey A. (2011). Micro and macroalgal biomass: A renewable source for bioethanol. Bioresource Technol. 102, 186–193. doi: 10.1016/j.biortech.2010.06.139
Jung K. A., Lim S.-R., Kim Y., Park J. M. (2013). Potentials of macroalgae as feedstocks for biorefinery. Bioresource Technol. 135, 182–190. doi: 10.1016/j.biortech.2012.10.025
Kang J. W. (1982a). Some seaweed diseases occurred at seaweed farms along the south-eastern coast of Korea. Bull. Korean Fisheries Soc. 14, 65–170.
Kang J. W. (1982b). Some seaweed diseases occurred at seaweed farms along the south-eastern coast of Korea. Bull. Korean Fisheries Soc. 14, 165–170.
Kapp M., Knippers R., Müller D. G. (1997). New members of a group of DNA viruses infecting brown algae. Phycological Res. 45, 85–90. doi: 10.1111/j.1440-1835.1997.tb00067.x
Karling J. S. (1944). Phygomyxa algarum n.gen., n.sp., an unusual parasite with plasmodiophoralean and protomyxean characteristics. Am. J. Bot. 31, 38–52. doi: 10.1002/j.1537-2197.1944.tb08000.x
Karsten U. (2007). Research note: Salinity tolerance of Arctic kelps from Spitsbergen. Phycological Res. 55, 257–262. doi: 10.1111/j.1440-1835.2007.00468.x
Kaur M., Saini K. C., Ojah H., Sahoo R., Gupta K., Kumar A., et al. (2022). Abiotic stress in algae: response, signaling and transgenic approaches. J. Appl. Phycology 34, 1843–1869. doi: 10.1007/s10811-022-02746-7
Kawai H., Tokuyama M. (1995). Laminarionema elsbetiae gen.etsp.nov.(Ectocarpales,Phaeophyceae), a new endophyte in Laminaria sporophytes. Phycological Res. 43, 185–190. doi: 10.1111/j.1440-1835.1995.tb00024.x
Kerrison P. D., Stanley M. S., Edwards M. D., Black K. D., Hughes A. D. (2015). The cultivation of European kelp for bioenergy: Site and species selection. Biomass Bioenergy 80, 229–242. doi: 10.1016/j.biombioe.2015.04.035
Kim S. K., Bhatnagar I. (2011). Physical, chemical, and biological properties of wonder kelp-laminaria. Adv. Food Nutr. Res. 64, 85–96. doi: 10.1016/B978-0-12-387669-0.00007-7
Kim G. H., Moon K.-H., Kim J.-Y., Shim J., Klochkova T. A. (2014). A revaluation of algal diseases in Korean Pyropia (Porphyra) sea farms and their economic impact. Algae 29, 249–265. doi: 10.4490/algae.2014.29.4.249
Kim J. K., Yarish C., Hwang E. K., Park M., Kim Y. (2017). Seaweed aquaculture: cultivation technologies, challenges and its ecosystem services. ALGAE 32, 1–13. doi: 10.4490/algae.2017.32.3.3
Kito H., Akiyama K., Sasaki M. (1976). Electron microscopic observations on the diseased thalli of Undaria pinnatifida (Harvey) Suringar, caused by parasitic bacteria. Bull. Tokoju Regional Fisheries Res. Lab. 36, 67–73.
Kohlmeyer J. (1972). Parasitic Haloguignardia oceanica (Ascomycetes) and Hyperparasitic Sphaceloma cecidii sp. nov. (Deuteromycetes) in Drift Sargassum in North Carolina. J. Elisha Mitchell Sci. Soc. 88, 255–259.
Koonin E. V., Dolja V. V., Krupovic M., Varsani A., Wolf Y. I., Yutin N., et al. (2020). Global organization and proposed megataxonomy of the virus world. Microbiol. Mol. Biol. Reviews: MMBR 84 (2), 1–33. doi: 10.1128/MMBR.00061-19
Krause-Jensen D., Duarte C. M. (2014). Expansion of vegetated coastal ecosystems in the future Arctic. Front. Mar. Sci. 1. doi: 10.3389/fmars.2014.00077
Krishnamurthy V. (1972). A revision of the species of the algal genus Porphyra occurring on the Pacific coast of North America. Pac Sci. 26, 24–49.
Krumhansl K. A., Okamoto D. K., Rassweiler A., Novak M., Bolton J. J., Cavanaugh K. C., et al. (2016). Global patterns of kelp forest change over the past half-century. Proc. Natl. Acad. Sci. United States America 113, 13785–13790. doi: 10.1073/pnas.1606102113
Kubanek J., Jensen P. R., Keifer P. A., Sullards M. C., Collins D. O., Fenical W. (2003). Seaweed resistance to microbial attack: A targeted chemical defense against marine fungi. Proc. Natl. Acad. Sci. 100, 6916–6921. doi: 10.1073/pnas.1131855100
Küpper F. C., Müller D. G. (1999). Massive occurrence of the heterokont and fungal parasites Anisolpidium, Eurychasma and Chytridium in Pylaiella littoralis (Ectocarpales, Phaeophyceae). NOVA HEDWIGIA 69, 381–389. doi: 10.1127/nova.hedwigia/69/1999/381
Küpper F. C., Müller D. G., Peters A. F., Kloareg B., Potin P. (2002). Oligoalginate recognition and oxidative burst play a key role in natural and induced resistance of sporophytes of laminariales. J. Chem. Ecol. 28, 2057–2081. doi: 10.1023/a:1020706129624
Lei F., Chenhui Z., Qi L. I. N., Longchen T., Zhongyan H. (2022). Microbial community structure and its relationship with environmental factors on Hole-Rotten disease of Saccharina japonica in coastal Putian. J. Fisheries China 46, 1721–1731. doi: 10.11964/jfc.20210112619
Lein T. E., Sjøtun K., Wakili S. (1991). Mass-occurrence of a brown filamentous endophyte in the lamina of the kelp Laminaria hyperborea (Gunnerus) foslie along the southwestern coast of Norway. Sarsia 76, 187–193. doi: 10.1080/00364827.1991.10413474
Leonard G. H. (1994). Effect of the bat star Asterina miniata (Brandt) on recruitment of the giant kelp Macrocystis pyrifera C. Agardh. J. Exp. Mar. Biol. Ecol. 179, 81–98. doi: 10.1016/0022-0981(94)90018-3
Lesser M. P. (2006). Oxidative stress in marine environments: biochemistry and physiological ecology. Annu. Rev. Physiol. 68, 253–278. doi: 10.1146/annurev.physiol.68.040104.110001
Li J., Pang S., Shan T., Su L. (2020). Changes of microbial community structures associated with seedlings of Saccharina japonica at early stage of outbreak of green rotten disease. J. Appl. Phycol 32, 1323–1327. doi: 10.1007/s10811-019-01975-7
Li Y., Zhang S., Sun Y., Wang H., Chen W. (2021). Pathogen identification and causative analysis of ulceration of sporophyte of seaweed Undaria pinnatifida. J. Dalian Ocean Univ. 36 (2), 248–253.
Li W., Zhang T., Tang X., Wang B. (2010). Oomycetes and fungi: important parasites on marine algae. Acta Oceanologica Sin. 29, 74–81. doi: 10.1007/s13131-010-0065-4
Lili W., Xuexi T., Peiyu W. M., Chuanguo L. (2004). Studies on physiological and biochemical changes of laminaria japonica during the occurrence of rot disease(II)–changes of PAL activity, PPO activity and polyphenol content. Adv. Mar. Sci. 22, 73–76.
Lima F. P., Ribeiro P. A., Queiroz N., Hawkins S. J., Santos A. M. (2007). Do distributional shifts of northern and southern species of algae match the warming pattern? Global Change Biol. 13, 2592–2604. doi: 10.1111/j.1365–2486.2007.01451.x
Lind A. C., Konar B. (2017). Effects of abiotic stressors on kelp early life-history stages. ALGAE 32, 223–233. doi: 10.4490/algae.2017.32.8.7
Ling S. D. (2008). Range expansion of a habitat-modifying species leads to loss of taxonomic diversity: a new and impoverished reef state. Oecologia 156, 883–894. doi: 10.1007/s00442-008-1043-9
Ling F., Egan S., Zhuang Y., Chang L., Xiao L., Yang Q., et al. (2022). Epimicrobiome shifts with bleaching disease progression in the brown seaweed saccharina japonica. Front. Mar. Sci. 9. doi: 10.3389/fmars.2022.865224
Liu C., Wang L., Wang M., Tang X. (2002). Difference analysis of infection activity of alginic acid decomposing bacteria infecting Laminaria japonica. Mar. Sci. 26, 44–47.
Lobban C. S. (1978). The growth and death of the Macrocystis sporophyte (Phaeophyceae, Laminariales). Phycologia 17, 196–212. doi: 10.2216/i0031-8884-17-2-196.1
Lobban C. S., Baxter D. M. (1983). Distribution of the Red Algal Epiphyte Polysiphonia lanosa on Its Brown Algal Host Ascophyllum nodosum in the Bay of Fundy, Canada. Botanica Marina 26 (11), 533–538. doi: 10.1515/botm.1983.26.11.533
Lordan S., Ross R. P., Stanton C. (2011). Marine bioactives as functional food ingredients: potential to reduce the incidence of chronic diseases. Mar. Drugs 9, 1056–1100. doi: 10.3390/md9061056
Lubchenco J., Gaines S. D. (1981). A unified approach to marine plant-herbivore interactions. I. Populations and communities. Annu. Rev. Ecol. Systematics 12, 405–437. doi: 10.1146/annurev.es.12.110181.002201
M. H., Boraso A. (1979). Ciclos de los bosques de Macrocystis pyrifera en Bahía Camarones, prov. del Chubut, Argentina. Ecosur ISSN 0325–108x 6, 165–184.
Ma Y., Yang Z., Wan L., Ge M., Zhang K. (1998). Pathogenic bacteria of spot decay disease found in Undaria pinnatifida. Paper presented at the Proceedings of International Symposium on Progress and Prospect of Marine Biotechnology (ISPPMB 1998), Qingdao, China. Journey of Fishery Sciences of China 4, 356–360.
Maier I., Müller D. G., Katsaros C. (2002). Entry of the DNA virus, Ectocarpus fasciculatus virus type 1 (Phycodnaviridae), into host cell cytosol and nucleus. Phycological Res. 50, 227–231. doi: 10.1111/j.1440-1835.2002.tb00155.x
Maier I., Parodi E., Westermeier R., Müller D. G. (2000). Maullinia ectocarpii gen. et sp. nov. (Plasmodiophorea), an Intracellular Parasite in Ectocarpus siliculosus (Ectocarpales, Phaeophyceae) and other Filamentous Brown Algae. Protist 151, 225–238. doi: 10.1078/1434-4610-00021
Maier I., Wolf S., Delaroque N., Müller D. G., Kawai H. (1998). A DNA virus infecting the marine brown alga Pilayella littoralis (Ectocarpales, Phaeophyceae) in culture. Eur. J. Phycology 33, 213–220. doi: 10.1080/09670269810001736713
Mann K. H. (1973). Seaweeds: Their productivity and strategy for growth. Science 182, 975–981. doi: 10.1126/science.182.4116.975
Mann K. H. (2000). Ecology of coastal waters: with implications for management (Hoboken, New Jersey, U.S.: Blackwell Science). Available at: https://www.wiley.com/en-us/Ecology+of+Coastal+Waters%3A+With+Implications+For+Management%2C+2nd+Edition-p-9780865425507.
Marano A. V., Pires-Zottarelli C. L. A., de Souza J. I., Glockling S. L., Leaño E., Gachon C. M. M., et al. (2012). 11 hyphochytriomycota, oomycota and perkinsozoa (Super-group chromalveolata). Berlin, Germany: Walter de Gruyter, 167–214. doi: 10.1515/9783110264067.167
Marcogliese D. J. (2004). Parasites: small players with crucial roles in the ecological theater. EcoHealth 1, 151–164. doi: 10.1007/s10393-004-0028-3
Marcogliese D. J. (2008). The impact of climate change on the parasites and infectious diseases of aquatic animals. Rev Sci Tech (International Office of Epizootics) 27 (2), 467–484.
Marinho G. S., Holdt S. L., Birkeland M. J., Angelidaki I. (2015). Commercial cultivation and bioremediation potential of sugar kelp, Saccharina latissima, in Danish waters. J. Appl. Phycology 27, 1963–1973. doi: 10.1007/s10811-014-0519-8
Markel R. W., DeWreede R. E. (1998). Mechanisms underlying the effect of the chiton Katharina tunicata on the kelp Hedophyllum sessile: size escapes and indirect effects. Mar. Ecol. Prog. Ser. 166, 151–161. doi: 10.3354/meps166151
Marzinelli E. M., Campbell A. H., Zozaya Valdes E., Vergés A., Nielsen S., Wernberg T., et al. (2015). Continental-scale variation in seaweed host-associated bacterial communities is a function of host condition, not geography. Environ. Microbiol. 17, 4078–4088. doi: 10.1111/1462-2920.12972
Mazariegos-Villarreal A., Piñón-Gimate A., Aguilar F., Medina M. A., Serviere-Zaragoza E. (2013). Diet of the keyhole limpet megathura crenulata (Mollusca: gastropoda) in subtropical rocky reefs. J. Shellfish Res. 32, 297–303. doi: 10.2983/035.032.0208
Mckeown D. A., Schroeder J. L., Stevens K., Peters A. F., Claudio A. S., Park J., et al. (2018). Phaeoviral infections are present in Macrocystis, Ecklonia and Undaria (Laminariales) and are influenced by wave exposure in Ectocarpales. Viruses 10, 410. doi: 10.3390/v10080410
McKeown D., Schroeder J., Stevens K., Peters A., Sáez C., Park J., et al. (2018). Phaeoviral infections are present in Macrocystis, Ecklonia and Undaria (Laminariales) and are influenced by wave exposure in Ectocarpales. Viruses 10, 410. doi: 10.3390/v10080410
McKeown D. A., Stevens K., Peters A. F., Bond P., Harper G. M., Brownlee C., et al. (2017). Phaeoviruses discovered in kelp (Laminariales). ISME J. 11, 2869–2873. doi: 10.1038/ismej.2017.130
Meints R. H., Ivey R. G., Lee A. M., Choi T.-J. (2008). Identification of two virus integration sites in the brown alga Feldmannia chromosome. J. Virol. 82, 1407–1413. doi: 10.1128/JVI.01983-07
Monteiro P., Lomartire S., Cotas J., Pacheco D., Marques J. C., Pereira L., et al. (2021). Seaweeds as a fermentation substrate: A challenge for the food processing industry. Processes 9 (11), 1953. doi: 10.3390/pr9111953
Morris R. H., Abbott D. P., Haderlie E. C. (1980). Intertidal invertebrates of California (Stanford University Press, Stanford, Calif). Available at: https://worldcat.org/title/7043400.
Mouritsen O. G., Dawczynski C., Duelund L., Jahreis G., Vetter W., Schröder M. (2013). On the human consumption of the red seaweed dulse (Palmaria palmata (L.) Weber & Mohr). J. Appl. Phycology 25, 1777–1791. doi: 10.1007/s10811-013-0014-7
Moy F. E., Christie H. (2012). Large-scale shift from sugar kelp (Saccharina latissima) to ephemeral algae along the south and west coast of Norway. Mar. Biol. Res. 8, 309–321. doi: 10.1080/17451000.2011.637561
Msuya F. E., Bolton J., Pascal F., Narrain K., Nyonje B., Cottier-Cook E. J. (2022). Seaweed farming in Africa: current status and future potential. J. Appl. Phycology 34, 985–1005. doi: 10.1007/s10811-021-02676-w
Müller D. G., Brautigam M., Kntppers R., Muller D. G. (1996a). Virus infection and persistence of foreign DNA in the marine brown alga Feldmannia simplex (Ectocarpales, Phaeophyceae). Phycologia 35, 61–63. doi: 10.2216/i0031–8884-35–1-61.1
Müller D. G., Kapp M., Knippers R. (1998). Viruses in marine brown algae. Adv. Virus Res. 50, 49–67. doi: 10.1016/S0065–3527(08)60805–2
Müller D. G., Kawai H., Stache B., Lanka S. (1990). A virus infection in the marine brown alga Ectocarpus siliculosus (Phaeophyceae). Botanica Acta 103, 72–82. doi: 10.1111/j.1438-8677.1990.tb00129.x
Müller D. G., Küpper F. C., Küpper H. (1999). Infection experiments reveal broad host ranges of Eurychasma dicksonii (Oomycota) and Chytridium polysiphoniae (Chytridiomycota), two eukaryotic parasites in marine brown algae (Phaeophyceae). Phycological Res. 47, 217–223. doi: 10.1111/j.1440-1835.1999.tb00301.x
Müller D. G., Parodi E. (1993). Transfer of a marine DNA virus from Ectocarpus to Feldmannia (Ectocarpales, Phaeophyceae): aberrant symptoms and restitution of the host. Protoplasma 175, 121–125. doi: 10.1007/BF01385009
Müller D. G., Sengco M., Wolf S., Bräutigam M., Schmid C. E., Kapp M., et al. (1996b). Comparison of two DNA viruses infecting the marine brown algae Ectocarpus siliculosus and E. fasciculatus. J. Gen. Virol. 77, 2329–2333. doi: 10.1099/0022-1317-77-9-2329
Müller D. G., Stache B. (1992). Worldwide occurrence of virus-infections in filamentous marine brown algae. Helgoländer Meeresuntersuchungen 46, 1–8. doi: 10.1007/BF02366208
Müller D. G., Westermeier R., Morales J., Reina G. G., del Campo E., Correa J. A., et al. (2000). Massive prevalence of viral DNA in Ectocarpus (Phaeophyceae, Ectocarpales) from two habitats in the North Atlantic and South Pacific. Botanica Marina 43, 157–159. doi: 10.1515/BOT.2000.016
Murúa P., Goecke F., Westermeier R., van West P., Küpper F. C., Neuhauser S. (2017). Maullinia braseltonii sp. nov. (Rhizaria, Phytomyxea, Phagomyxida): A Cyst-forming Parasite of the Bull Kelp Durvillaea spp. (Stramenopila, Phaeophyceae, Fucales). Protist 168, 468–480. doi: 10.1016/j.protis.2017.07.001
Murúa P., Küpper F. C., Muñoz L. A., Bernard M., Peters A. F. (2018). Microspongium alariae in Alaria esculenta: a widely-distributed non-parasitic brown algal endophyte that shows cell modifications within its host. Botanica Marina 61 (4), 343–354. doi: 10.1515/bot-2017–0095
Myers A. A. (1974). “Amphitholina cuniculus (Strebing), a little-known marine amphipod crustacean new to Ireland,” in Proceedings of the Royal Irish Academy. Section B: Biological, Geological, and Chemical Science. 74B, 463–469.
Neushul M., Haxo F. T. (1963). Studies on the giant kelp, macrocystis. I. Growth of young plants. Am. J. Bot. 50, 349–353. doi: 10.2307/2440151
Nielsen R. (1979). Culture studies on the type species of Acrochaete, Bolbocoleon and Entocladia (Chaetophoraceae, Chlorophyceae). Bot. Notiser 132, 441–449.
North W. (1971). The biology of giant kelp beds (Macrocystis) in California, Vol. 32. Stuttgart, Germany: Schweizerbart and Borntraeger science publishers.
Notoya M., Miyashita A. (1999). Life history, in culture, of the obligate epiphyte Porphyra moriensis (Bangiales, Rhodophyta). Hydrobiologia 398, 121–125. doi: 10.1023/A:1017023210883
Ohmi H. (1954). New species of Porphyra, epiphytic on Chorda filum from Hokkaido. Bull. Faculty Fisheries Hokkaido Univ. 5, 231–239.
O’Kelly C. J. (1983). Observations on marine Chaetophoraceae (Chlorophyta). IV. The structure, reproduction, and life history of Acrochaete geniculata (Gardner) comb. nov. Phycologia 22, 13–21. doi: 10.2216/i0031–8884-22–1-13.1
Okida J. (1960). Marine algae epiphytes on Laminariales plants. Bull. Fac. Fish. Hokkaido Univ. 11, 72–105.
Pang S. J., Zhang Z. H., Zhao H. J., Sun J. Z. (2007). Cultivation of the brown alga Hizikia fusiformis (Harvey) Okamura: stress resistance of artificially raised young seedlings revealed by chlorophyll fluorescence measurement. J. Appl. Phycology 19, 557–565. doi: 10.1007/s10811-007-9170-y
Paul V. J., Hay M. E. (1986). Seaweed susceptibility to herbivory: chemical and morphological correlates. Mar. Ecol. Prog. Ser. 33, 255–264. doi: 10.3354/meps033255
Pereira R. C., Bianco É.M., Bueno L. B., de Oliveira M. A. L., Pamplona O. S., da Gama B. A. P. (2010). Associational defense against herbivory between brown seaweeds. Phycologia 49, 424–428. doi: 10.2216/09-84.1
Perris S. (2018). What does Hine-nui-te-po look like?: A case study of oral tradition, myth and literature in Aotearoa New Zealand. J. Polynesian Soc. 127, 365–388. doi: 10.15286/jps.127.4.365-388
Pessarrodona A., Moore P. J., Sayer M. D. J., Smale D. A. (2018). Carbon assimilation and transfer through kelp forests in the NE Atlantic is diminished under a warmer ocean climate. Global Change Biol. 24, 4386–4398. doi: 10.1111/gcb.14303
Pessoa M. F. (2012). Harmful effects of UV radiation in algae and aquatic macrophytes - A Review. Emirates J. Food Agric. 24, 510–526. doi: 10.9755/ejfa.v24i6.14671
Peteiro C., Sánchez N., Martínez B. (2016). Mariculture of the Asian kelp Undaria pinnatifida and the native kelp Saccharina latissima along the Atlantic coast of Southern Europe: An overview. Algal Res. 15, 9–23. doi: 10.1016/j.algal.2016.01.012
Peters A. F., Chapman A. R. O., Anderson R. J., Vreeland V., Davison I. R. (2003) Molecular identification, distribution and taxonomy of brown algal endophytes, with emphasis on species from Antarctica. Available online at: https://api.semanticscholar.org/CorpusID:82542967.
Peters A. F., Schaffelke B. (1996). Streblonema (Ectocarpales, Phaeophyceae) infection in the kelp Laminaria saccharina (Laminariales, Phaeophyceae) in the western Baltic. Hydrobiologia 326, 111–116. doi: 10.1007/BF00047795
Petersen H. E. (1905). Contributions à la connaissance des phycomycètes marins (Chytridinae Fischer). Kgl. Danske Videnskabernes Selskabs Forhandlinger 5, 439–488.
Philippsen A., Wild P., Rowe A. (2014). Energy input, carbon intensity and cost for ethanol produced from farmed seaweed. Renewable Sustain. Energy Rev. 38, 609–623. doi: 10.1016/j.rser.2014.06.010
Phillips J. A. (2001). Marine macroalgal biodiversity hotspots: why is there high species richness and endemism in southern Australian marine benthic flora? Biodiversity Conserv. 10, 1555–1577. doi: 10.1023/A:1011813627613
Pinnegar J. K., Polunin N. V. C., Francour P., Badalamenti F., Chemello R., Harmelin-Vivien M.-L., et al. (2000). Trophic cascades in benthic marine ecosystems: lessons for fisheries and protected-area management. Environ. Conserv. 27, 179–200. doi: 10.1017/S0376892900000205
Pörtner H.-O., Roberts D., Tignor M., Poloczanska E., Mintenbeck K., Alegría A., et al. (2022). Climate Change 2022: Impacts, Adaptation and Vulnerability Working Group II Contribution to the Sixth Assessment Report of the Intergovernmental Panel on Climate Change. Cambridge University Press. Cambridge University Press, Cambridge, UK and New York, NY, USA, 3056 pp. doi: 10.1017/9781009325844
Purcell-Meyerink D., Packer M. A., Wheeler T. T., Hayes M., Franco Ruiz D., López-Pedrouso M., et al. (2021). Aquaculture Production of the Brown Seaweeds Laminaria digitata and Macrocystis pyrifera: Applications in Food and Pharmaceuticals. Molecules 26, 1306. doi: 10.3390/molecules26051306
Quigley D. T. G., Fenwick D. (2017). Epibiont attachment of the brown alga Cladostephus spongiosum (Hudson) C. Agardh 1817 (Phaeophyceae: sphacelariaceae) on the auger shell turritella communis risso 1826 (Gastropoda: cerithiodea: turritellidae). PMNHS Bull. 8, 58–60.
Raybaud V., Beaugrand G., Goberville E., Delebecq G., Destombe C., Valero M., et al. (2013). Decline in kelp in West Europe and climate. PloS One 8, 1–10. doi: 10.1371/journal.pone.0066044
Reboleira J., Silva S., Chatzifragkou A., Niranjan K., Lemos M. F. L. (2021). Seaweed fermentation within the fields of food and natural products. Trends Food Sci. Technol. 116, 1056–1073. doi: 10.1016/j.tifs.2021.08.018
Reed R. H., Davison I. R., Chudek J. A., Foster R. (1985). The osmotic role of mannitol in the Phaeophyta: an appraisal. Phycologia 24, 35–47. doi: 10.2216/i0031-8884-24-1-35.1
Ricketts E. F., Calvin J. (1968). Between Pacific Tides. California: Stanford University Press Stanford.
Roleda M. Y., Lüder U. H., Wiencke C. (2010). UV-susceptibility of zoospores of the brown macroalga Laminaria digitata from Spitsbergen. Polar Biol. 33, 577–588. doi: 10.1007/s00300-009-0733-z
Rugiu L., Panova M., Pereyra R. T., Jormalainen V. (2020). Gene regulatory response to hyposalinity in the brown seaweed Fucus vesiculosus. BMC Genomics 21, 42. doi: 10.1186/s12864-020-6470-y
Ruiz Martínez E., Mckeown D. A., Schroeder D. C., Thuestad G., Sjøtun K., Sandaa R.-A., et al. (2023). Phaeoviruses Present in Cultured and Natural Kelp Species, Saccharina latissima and Laminaria hyperborea (Phaeophyceae, Laminariales), in Norway. Viruses 15 (12), 2331. doi: 10.3390/v15122331
Russell G. (1964). Laminariocolax tomentosoides on the Isle of Man. J. Mar. Biol. Assoc. United Kingdom 44, 601–612. doi: 10.1017/S0025315400027806
Russell G. (1983). Formation of an ectocarpoid epiflora on blades of Laminaria digitata. Mar. Ecol. Prog. Ser. 11, 181–187. doi: 10.3354/meps011181
Saderne V., Wahl M. (2013). Differential responses of calcifying and non-calcifying epibionts of a brown macroalga to present-day and future upwelling pCO2. PloS One 8, e70455. doi: 10.1371/journal.pone.0070455
Santelices B., Ojeda F. P. (1984). Effects of canopy removal on the understory algal community structure of coastal forests of Macrocystispyrifera from southern South America. Mar. Ecol. Prog. Ser. 14, 165–173. doi: 10.3354/meps014165
Saunders M. I., Metaxas A., Filgueira R. (2010). Implications of warming temperatures for population outbreaks of a nonindigenous species (Membranipora membranacea, Bryozoa) in rocky subtidal ecosystems. Limnology Oceanography 55, 1627–1642. doi: 10.4319/lo.2010.55.4.1627
Schatz S. (1983). The developmental morphology and life history of phycomelaina laminariae. Mycologia 75, 762–772. doi: 10.1080/00275514.1983.12023752
Schiener P., Black K. D., Stanley M. S., Green D. H. (2014). The seasonal variation in the chemical composition of the kelp species Laminaria digitata, Laminaria hyperborea, Saccharina latissima and Alaria esculenta. J. Appl. Phycology 27, 363–373. doi: 10.1007/s10811-014-0327-1
Schroeder D. C. (2011). “Viruses of Seaweeds,” in Studies in viral ecology. Ed. Hurst C. J. (Hoboken, NJ, USA: John Wiley & Sons, Inc), 205–215. doi: 10.1002/9781118025666.ch8
Schroeder D. C., C. D. (2015). More to Phaeovirus infections than first meets the eye. Perspect. Phycology 2, 105–109. doi: 10.1127/pip/2015/0034
Schroeder D. C., Park Y., Yoon H.-M., Lee Y. S., Kang S. W., Meints R. H., et al. (2009). Genomic analysis of the smallest giant virus — Feldmannia sp. virus 158. Virology 384, 223–232. doi: 10.1016/j.virol.2008.10.040
Schroeder D. C., Schoenrock K. M., McHugh T. A., Ray J., Krueger-Hadfield S. A. (2023). Phaeoviruses found in recovering Nereocystis luetkeana kelp forest community. J. Phycology 59 (5), 818–821. doi: 10.1111/jpy.13378
Selivanova O. N., Zhigadlova G. G. (2013). Marine benthic algae of the commander islands (Pacific coast of Russia) with checklist revised in 2012. ISRN Oceanography 2013, 470185. doi: 10.5402/2013/470185
Sengco M. R., Bräutigam M., Kapp M., Müller D. G. (1996). Detection of virus DNA in Ectocarpus siliculosus and E. fasciculatus (Phaeophyceae) from various geographic areas. Eur. J. Phycology 31, 73–78. doi: 10.1080/09670269600651221
Seymour R. J., Tegner M. J., Dayton P. K., Parnell P. E. (1989). Storm wave induced mortality of giant kelp, Macrocystis pyrifera, in Southern California. Estuarine Coast. Shelf Sci. 28, 277–292. doi: 10.1016/0272-7714(89)90018-8
Skjermo J., Aasen I. M., Arff J., Broch O. J., Carvajal A. K., Christie H. C., et al. (2014). A new Norwegian bioeconomy based on cultivation and processing of seaweeds: Opportunities and R&D needs SINTEF Fisheries and Aquaculture SINTEF Fisheries and Aquaculture (Trondheim, Norway: SINTEF Fisheries and Aquaculture). Available at: https://brage.bibsys.no/xmlui/handle/11250/2447671.
Smale D. A., Burrows M. T., Moore P., O’Connor N., Hawkins S. J. (2013). Threats and knowledge gaps for ecosystem services provided by kelp forests: a northeast Atlantic perspective. Ecol. Evol. 3, 4016–4038. doi: 10.1002/ece3.774
Smale D. A., Moore P. J. (2017). Variability in kelp forest structure along a latitudinal gradient in ocean temperature. J. Exp. Mar. Biol. Ecol. 486, 255–264. doi: 10.1016/j.jembe.2016.10.023
Smit A. J. (2004). Medicinal and pharmaceutical uses of seaweed natural products: A review. J. Appl. Phycology 16, 245–262. doi: 10.1023/B:JAPH.0000047783.36600.ef
Song M., Duc Pham H., Seon J., Chul Woo H. (2015). Marine brown algae: A conundrum answer for sustainable biofuels production. Renewable Sustain. Energy Rev. 50, 782–792. doi: 10.1016/j.rser.2015.05.021
South G. R. (1968). Aspects of the development and reproduction of Acrochaete repens and Bolbocoleon piliferum. Can. J. Bot. 46, 101–113. doi: 10.1139/b68-020
South G. R. (1974). Herpodiscus gen.nov. and Herpodiscus durvilleae (Lindauer) comb.nov., a parasite of Durvillea Antarctica (Chamisso) hariot endemic to New Zealand. J. R. Soc. New Z. 4, 455–461. doi: 10.1080/03036758.1974.10419388
Stekoll M. S., Peeples T. N., Raymond A. E. T. (2021). Mariculture research of Macrocystis pyrifera and Saccharina latissima in Southeast Alaska. J. World Aquaculture Soc. 52, 1031–1046. doi: 10.1111/jwas.12765
Steneck R. S., Graham M. H., Bourque B. J., Corbett D., Erlandson J. M., Estes J. A., et al. (2002). Kelp forest ecosystems: biodiversity, stability, resilience and future. Environ. Conserv. 29, 436–459. doi: 10.1017/S0376892902000322
Stévant P., Rebours C., Chapman A. (2017). Seaweed aquaculture in Norway: recent industrial developments and future perspectives. Aquaculture Int. 25, 1373–1390. doi: 10.1007/s10499-017-0120-7
Stockenreiter M., Haupt F., Graber A.-K., Seppälä J., Spilling K., Tamminen T., et al. (2013). Functional group richness: implications of biodiversity for light use and lipid yield in microalgae. J. Phycology 49, 838–847. doi: 10.1111/jpy.12092
Strittmatter M., Grenville-Briggs L. J., Breithut L., Van West P., Gachon C. M. M., Küpper F. C. (2016). Infection of the brown alga Ectocarpus siliculosus by the oomycete Eurychasma dicksonii induces oxidative stress and halogen metabolism. Plant Cell Environ. 39, 259–271. doi: 10.1111/pce.12533
Sugumaran R., Padam B. S., Yong W. T. L., Saallah S., Ahmed K., Yusof N. A. (2022). Retrospective review of global commercial seaweed production-current challenges, biosecurity and mitigation measures and prospects. Int. J. Environ. Res. Public Health 19 (12), 7087. doi: 10.3390/ijerph19127087
Sutherland G. K. (1915a). Additional notes on marine Pyrenomycetes. New Phytol. 14, 183–193. doi: 10.1111/j.1469-8137.1915.tb07185.x
Sutherland G. K. (1915b). New marine phycomycetes. Trans. Br. Mycological Soc. 5, 147–155. doi: 10.1111/j.1469-8137.1915.tb07171.x
Troell M., Robertson-Andersson D., Anderson R. J., Bolton J. J., Maneveldt G., Halling C., et al. (2006). Abalone farming in South Africa: An overview with perspectives on kelp resources, abalone feed, potential for on-farm seaweed production and socio-economic importance. Aquaculture 257, 266–281. doi: 10.1016/j.aquaculture.2006.02.066
Tropin I. V., Radzinskaya N. V., Voskoboinikov G. M. (2003). The influence of salinity on the rate of dark respiration and structure of the cells of brown algae thalli from the Barents sea littoral. Biol. Bull. Russian Acad. Sci. 30, 40–47. doi: 10.1023/A:1022063426675
Tseng C. K. (1962). Manual of Cultivation of Haidai (Laminaria Japonica) (Beijing: Science Press), 99–122.
Tsukidate J. (1991). Seaweed disease. Fish health management in Asia-Pacific. Report on a regional study and Workshop on Fish Disease and Fish Health Management (ADB/NACA: Bangkok, Thailand), 397–408.
Uchida M., Miyoshi T. (2013). Algal fermentation - the seed for a new fermentation industry of foods and related products. Japan Agric. Res. Quarterly: JARQ 47, 53–63. doi: 10.6090/jarq.47.53
Vairappan C. S., Suzuki M., Motomura T., Ichimura T. (2001). Pathogenic bacteria associated with lesions and thallus bleaching symptoms in the Japanese kelp Laminaria religiosa Miyabe (Laminariales, Phaeophyceae). Hydrobiologia 445, 183–191. doi: 10.1023/A:1017517832302
Valero M., Guillemin M.-L., Destombe C., Jacquemin B., Gachon C. M. M., Badis Y., et al. (2017). Perspectives on domestication research for sustainable seaweed aquaculture. Perspect. Phycology 4, 33–46. doi: 10.1127/pip/2017/0066
Vallet M., Strittmatter M., Murúa P., Lacoste S., Dupont J., Hubas C., et al. (2018). Chemically-mediated interactions between macroalgae, their fungal endophytes, and protistan pathogens. Front. Microbiol. 9. doi: 10.3389/fmicb.2018.03161
Van Alstyne K. L. (1989). Adventitious branching as a herbivore-induced defense in the intertidal brown alga Fucus distichus. Mar. Ecol. Prog. Ser. 56, 169–176. doi: 10.3354/meps056169
Vranken S., Wernberg T., Scheben A., Severn-Ellis A. A., Batley J., Bayer P. E., et al. (2021). Genotype–Environment mismatch of kelp forests under climate change. Mol. Ecol. 30, 3730–3746. doi: 10.1111/mec.15993
Wada S., MN A., Mikami A., Komatsu T., Tsuchiya Y., Sato T., et al. (2008). Bioavailability of macroalgal dissolved organic matter in seawater. Mar. Ecol. Prog. Ser. 370, 33–44. doi: 10.3354/meps07645
Wang W., Li X., Liang G., Zhao N., Shi L., Yang G. (2021). Description of a white spot disease of field cultivated kelp (Saccharina japonica) and evaluation of its influences on the growth and phytochemical contents. Aquat. Bot. 173, 103414. doi: 10.1016/j.aquabot.2021.103414
Wang G., Lu B., Shuai L., Li D., Zhang R. (2014). Microbial diseases of nursery and field-cultivated Saccharina japonica (Phaeophyta) in China. Algological Stud., 145–146, 39–51. doi: 10.1127/1864-1318/2014/0167
Wang Q. K., Shi C. L., Ma J. C. (1983). Isolation and cultivation of MLO associated with coiling- stunt disease of sea tangle. Acta Microbiologica Sin. 23, 73–74.
Wang G., Shuai L., Li Y., Lin W., Zhao X., Duan D. (2008). Phylogenetic analysis of epiphytic marine bacteria on Hole-Rotten diseased sporophytes of Laminaria japonica. J. Appl. Phycology 20, 403–409. doi: 10.1007/s10811-007-9274-4
Ward G. M., Faisan J. P., Cottier-Cook E. J., Gachon C., Hurtado A. Q., Lim P. E., et al. (2019). A review of reported seaweed diseases and pests in aquaculture in Asia. J. World Aquaculture Soc. 51, 815–828. doi: 10.1111/jwas.12649
Wargacki A. J., Leonard E., Win M. N., Regitsky D. D., Santos C. N. S., Kim P. B., et al. (2012). An engineered microbial platform for direct biofuel production from brown macroalgae. Science 335, 308–313. doi: 10.1126/science.1214547
Weinberger F. (2007). Pathogen-induced defense and innate immunity in macroalgae. Biol. Bull. 213, 290–302. doi: 10.2307/25066646
Wernberg T., Bennett S., Babcock R. C., de Bettignies T., Cure K., Depczynski M., et al. (2016). Climate-driven regime shift of a temperate marine ecosystem. Science 353, 169–172. doi: 10.1126/science.aad8745
Wernberg T., Krumhansl K., Filbee-Dexter K., Pedersen M. F. (2019). Status and trends for the world’s kelp forests,” in World Seas: An Environmental Evaluation Volume III: Ecological Issues and Environmental Impacts. Amsterdam, The Netherlands: Elsevier Academic Press, 57–78. doi: 10.1016/B978–0-12–805052–1.00003–6
Wernberg T., Thomsen M. S., Tuya F., Kendrick G. A., Staehr P. A., Toohey B. D. (2010). Decreasing resilience of kelp beds along a latitudinal temperature gradient: potential implications for a warmer future. Ecol. Lett. 13, 685–694. doi: 10.1111/j.1461-0248.2010.01466.x
Wheeler W. N. (1980). Effect of boundary layer transport on the fixation of carbon by the giant kelp Macrocystis pyrifera. Mar. Biol. 56, 103–110. doi: 10.1007/BF00397128
Wilson W. H., Allen M. J. (2011). “Viruses of eukaryotic algae,” in Studies in Viral Ecology. Ed. Hurst C. J. (Hoboken, NJ, USA: John Wiley & Sons, Inc), 189–203. doi: 10.1002/9781118025666.ch7
Wu C. Y., Dou C., Jiajun L. (1983). “On the diseases of cultivated Laminaria japonica,” in Proceedings of the Joint China-U.S. Phycology symposium. Ed. Tseng C. K. (Science Press, Beijing, China), 211–220.
Yan Y., Wang S., Liu K., Mo Z., Yang H., Rong X., et al. (2023). Divergence of epibacterial community assemblage correlates with malformation disease severity in Saccharina japonica seedlings. Front. Mar. Sci. 10. doi: 10.3389/fmars.2023.1089349
Young E. B., Berges J. A., Dring M. J. (2009). Physiological responses of intertidal marine brown algae to nitrogen deprivation and resupply of nitrate and ammonium. Physiologia Plantarum 135, 400–411. doi: 10.1111/j.1399-3054.2008.01199.x
Zhang X., Chen Y., Saha M., Zhuang Y., Chang L., Xiao L., et al. (2022). Pseudoalteromonas piscicida X-8 causes bleaching disease in farmed Saccharina japonica. Aquaculture 546, 737354. doi: 10.1016/j.aquaculture.2021.737354
Keywords: macroalgae, stressors, illnesses, seaweed, aquaculture, industry, Phaeophyceae, climate change
Citation: Ruiz Martínez E, Schroeder DC, Thuestad G and Hoell IA (2024) Brown algae (Phaeophyceae) stressors and illnesses: a review for a sustainable aquaculture under climate change. Front. Aquac. 3:1390415. doi: 10.3389/faquc.2024.1390415
Received: 23 February 2024; Accepted: 09 May 2024;
Published: 03 June 2024.
Edited by:
Felipe Ascencio, Centro de Investigación Biológica del Noroeste (CIBNOR), MexicoReviewed by:
Juan Manuel Pacheco-Vega, Autonomous University of Nayarit, MexicoAlvaro Anibal Israel, University of Haifa, Israel
Copyright © 2024 Ruiz Martínez, Schroeder, Thuestad and Hoell. This is an open-access article distributed under the terms of the Creative Commons Attribution License (CC BY). The use, distribution or reproduction in other forums is permitted, provided the original author(s) and the copyright owner(s) are credited and that the original publication in this journal is cited, in accordance with accepted academic practice. No use, distribution or reproduction is permitted which does not comply with these terms.
*Correspondence: Eliana Ruiz Martínez, ZWxybUBodmwubm8=