- 1Department of Animal Production and Preventive Veterinary Medicine, São Paulo State University "Júlio de Mesquita Filho" (UNESP), Botucatu, São Paulo, Brazil
- 2Faculty of Veterinary Medicine, Federal University of Uberlândia (UFU), Uberlândia, Minas Gerais, Brazil
- 3Department of Veterinary Medicine, Federal University of Viçosa (UFV), Viçosa, Brazil
- 4Department of Veterinary Sciences, Federal University of Paraná (UFPR), Palotina, Brazil
Introduction: The intensification of tilapia production has increased animal density in tanks, leading to more frequent exposure to pathogenic agents and compromising the quality of fish products. Antimicrobial resistance is a global concern that affects human treatment, and sentinel microorganisms like Escherichia coli are crucial for monitoring production chains, especially in aquaculture, where research is still limited. The aim of this study was to identify the presence of E. coli and investigate its antimicrobial resistance profiles throughout the entire tilapia production chain.
Methods: A total of 240 samples were collected from various points in the production process: carcasses before scaling (Ca), scaling wastewater (Sw), filleting wastewater (Fw), fillet washing wastewater (Tw), fillet handling surfaces (Su), and pre-packaged fillets (Pf). The samples were collected during 10 visits, each corresponding to animals from different farms. E. coli isolates were identified using MacConkey agar and biochemical tests. Phenotypic resistance profiles were determined using nine classes of antimicrobials. Extended- spectrum b-lactamase (ESBL) production was identified with ceftazidime and cefotaxime and confirmed by a double-disc synergy test. Isolates were classified as sensitive or resistant based on the inhibition zone. Multidrug-resistant (MDR) was defined as resistance to at least one agent in three or more antimicrobial categories, while extensively drug-resistant (XDR) was defined as resistance to at least one agent in all but two or fewer categories.
Results: Overall, 50.8% of the samples (122/240) tested positive for E. coli, with 403 isolates identified. Of these, 33% (133/403) were resistant to at least two antimicrobials, and 20% (48/240) of the samples had MDR isolates, with the highest frequency found at the filleting point (Fw), which also had the only XDR profile. Resistance was most commonly observed against amoxicillin (35.73%), tetracycline (30.77%), and ciprofloxacin (26.30%).
Discussion: These findings emphasize the importance of E. coli as an indicator of antimicrobial resistance throughout tilapia processing and highlight the need for good production practices and qualified technical support to mitigate risks to public health, animal health, and the environment.
Introduction
Brazil is recognized worldwide for its agro-export potential, moving the country’s economy and generating employment and income in the various work chains involved in agribusiness. Contributing to food security in several regions of the world, fish represents an important source of animal protein (Food and Agriculture Organization of the United Nations, 2020). The production of this food matrix is a growing market, with fish farming reaching 860,355 tons produced in 2022 in Brazil (Pedrini et al., 2023). This highlights the importance and growth of this chain, and its economic and social impacts since about 8% of the world population is dependent on this sector (Food and Agriculture Organization of the United Nations, 2020).
The production of tilapia (Oreochromis spp.) stands out worldwide. In Brazil, tilapia represents about 63.93% of the Brazilian fish production, keeping the country as the fourth largest producer in the world (Pedrini et al., 2023). The intensification of the system is a direct reflection of globalization, which has a growing demand for animal products (Dawood and Koshio, 2016). As a result, the adoption of biosecurity measures and good agricultural practices are essential for the prevention of disease outbreaks that compromise animal health and even consumer health due to their zoonotic nature (Van Boeckel et al., 2017).
Thus, the increased use of antimicrobials has been a common practice in intensive tilapia production systems, which are used as treatment, prophylaxis or metaphylaxis of batches (Smith, 2020). These practices have been pointed out as one of those responsible for the emergence of multidrug-resistant microorganisms, being a risk to animal, human and environmental health (Cabello, 2006). Considering that most of the Brazilian fish farms are in net cages in hydroelectric reservoir (Moura et al., 2016; Camargo and Amorim, 2020), the environmental impact becomes more relevant, as it increases the risk of multidrug-resistant bacteria dissemination. Therefore, these considerations highlight the importance of the topic within the concept of one health (Van Boeckel et al., 2017).
Among the measures that can be adopted, the investigation and monitoring of the antimicrobial resistance profile of bacteria is one of the first necessary actions (Preena et al., 2020; Caputo et al., 2023). This allows the elaboration of specific policies to combat resistance within aquaculture (Preena et al., 2020). In this sense, the use of sentinel microorganisms, as E. coli, has been shown to be effective and reliable (Mencía-Ares et al., 2022). In this context, E. coli is useful because it is characterized as an important disseminator of resistance genes (Poirel et al., 2018). This reinforces its importance as a target microorganism in monitoring programs (Lihan et al., 2021).
The rise in multidrug resistance globally poses a serious public health threat. Several recent studies have reported the emergence of MDR bacterial pathogens from diverse sources, emphasizing the necessity for the proper use of antibiotics. Furthermore, the routine implementation of antimicrobial susceptibility testing is crucial to identify effective antibiotics and detect emerging MDR strains (Algammal et al., 2022; Ibrahim et al., 2024; Mabrok et al., 2024).
Zoonotic pathogens such as E. coli, Salmonella spp., and Staphylococcus spp. can be present in fish production systems, compromising the safety of animal-derived products and affecting consumer health (Dhama et al., 2013). E. coli is known for its resistance to many antibiotics and its ability to spread resistance genes, highlighting the risk of contaminated water to biosafety and human health (Ng et al., 2018; Preena et al., 2021).
Although E. coli generally does not cause disease in fish, it can express virulence factors and cause infections in humans, making the implementation of good manufacturing practices and self-monitoring programs in production systems essential (Greenlees et al., 1998). As a gram-negative rod from the Enterobacteriaceae family, E. coli serves as an indicator of hygiene and can be transmitted through food, water, and soil (Croxen et al., 2013; Jang et al., 2017). Besides commensal strains, pathogenic strains that cause approximately 2 million deaths annually exist and can be classified into seven groups based on their virulence mechanisms (Croxen et al., 2013). E. coli is frequently associated with foodborne outbreaks, especially with raw products, emphasizing the need for an integrated approach to assess epidemiological impacts and risks (Walker et al., 2018).
Despite this importance, there are few studies that assess the antimicrobials resistance of bacteria in the fish production chain. Thus, the objective of this work was to evaluate the presence and resistance profile of E. coli from tilapia production chain.
Materials and methods
Sample collection
The project was carried out in a tilapia-processing industry that works under Official Brazilian Inspection System of Animal Products, located in southwestern Brazil. Ten visits to the industry were carried out, with a single origin of tilapia being slaughtered and collected at each visit. Fish-farming were identified from A to F (all under intensive production systems) and samples were collected at the following production points: carcasses before scaling (Ca); scaling wastewater (Sw); filleting wastewater (Fw); fillet toilet wastewater (Tw); fillet handling surface (Su); and pre-packaged fillets (Pf) (Table 1). For logistical reasons, this study considered Ca and Sw as representative of the Fish-farming microbiological conditions.
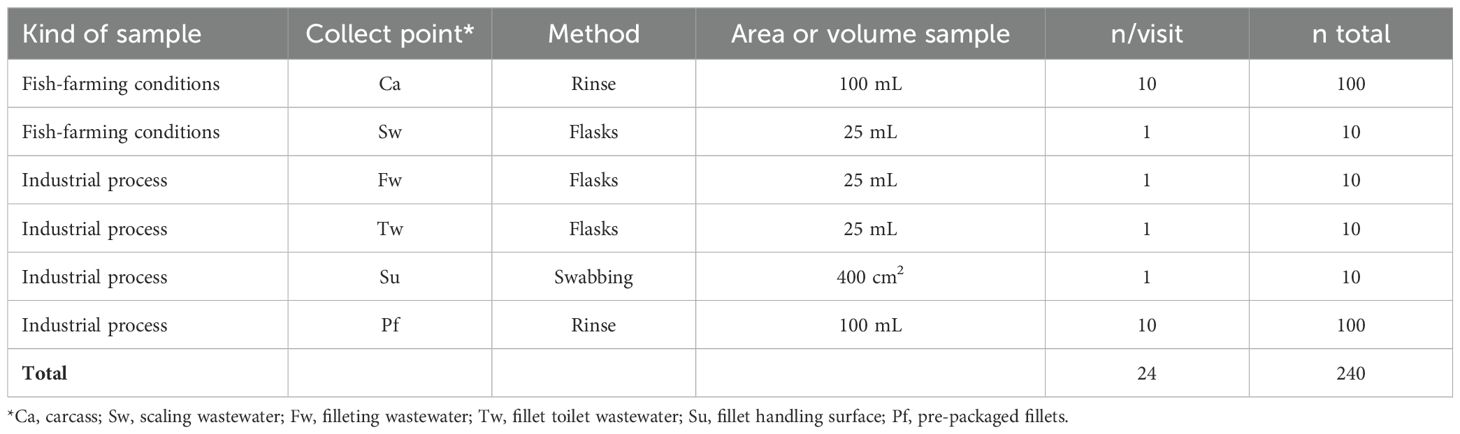
Table 1. Description of points, samples, and collection methods carried out in the tilapia-processing industry.
Ca and Pf points were sampled by superficial rinsing in sterile bags containing 100 mL of sterile saline solution (0.85% w/v). Su point was sample by swabbing two sterile sponges previously moistened with 20 mL of saline solution (0.85% w/v). For this procedure, sterile molds measuring 100 cm2 (10 cm x 10 cm) were used to delimit the area to be sampled, which was collected in 4 different places, totaling 400 cm2. At the points where the water was collected (Sw, Fw and Tw), sterile flasks containing sodium thiosulfate were used. After collection, the samples were placed in styrofoam box and kept at 4 °C until microbiological analysis.
Isolation and characterization of E. coli
Samples were subjected to E. coli isolation according to American Guidelines Public Health Association (APHA, 2001) Under sterile conditions, except for Su, 25 mL of each sample were added to 225 mL of Buffered Peptone Water (BPW CM0509 – Oxoid, Thermo Fisher Scientific, Waltham – EUA) (1:10). For Su, 20 mL were added to 180 mL of BPW (1:10). All samples were than homogenized in a stomacher for 60 seconds and incubated at 37°C for 18–24h. After this period, they were incubated in Escherichia coli broth (EC Broth CM0979 – Oxoid, Thermo Fisher Scientific, Waltham – EUA) at 45°C for 48h. Subsequently, aliquots of the broth were streaked onto MacConkey Agar (CM 0115, Oxoid, Thermo Fisher Scientific, Waltham – EUA) and incubated at 37°C for 18–24 h. If present, a total of four lactose fermenting colonies with typical E. coli morphology (on MacConkey agar, the colonies are pink, typically smooth, and may have a shiny appearance) and one non-lactose fermenting colony (colonies appear yellow or colorless) were selected.
All the suspected colonies selected were submitted to biochemical identification using EPM (Escola Paulista de Medicina), Mili (Motility, Indole, and Lysine) (De Toledo, 1982), and Simmons citrate (CM 0155, Oxoid, Thermo Fisher Scientific, Waltham – EUA). In typical biochemical tests for Escherichia coli, the following results are often observed: in the EPM test, gas production and glucose fermentation; and in the MiLLi test, positive results for lysine, indole, and motility, and negative for citrate. It is important to note that some Escherichia coli pathotypes may show negative results for lysine decarboxylation and motility.
Samples biochemically confirmed as E. coli were stored on Nutrient agar (CM 0003, Oxoid, Thermo Fisher Scientific, Waltham – EUA) and in Brain Heart Infusion (CM 1136, Oxoid, Thermo Fisher Scientific, Waltham – EUA) broth added with 10% glycerol and kept frozen.
Characterization of the phenotypic profile of antibiotic resistance in E. coli isolates
The sensitivity of E. coli isolates to antimicrobial agents was evaluated using the Kirby-Bauer disk diffusion methodology (Baurer et al., 1966), according to international recommendations (Clinical and Laboratory Standards Institute, 2018; Clinical and Laboratory Standards Institute, 2020). A total of nine classes of antimicrobials, commonly used in animal production and for human health, were tested: amoxicillin – AMO (10 μg); ceftiofur – CTF (30 µg); aztreonam – ATM (30 µg); imipenem – IPM (10 µg); ciprofloxacin – CIP (5 µg); tetracycline – TET (30 µg); gentamicin – GEN (10 μg), sulfamethoxazole + trimethoprim – SUT (23.75/1.25 μg), chloramphenicol – CLO (30 μg) and azithromycin – AZI (15 μg) (Antimicrobial disks, Interlab, São Paulo, Brazil). Extended-spectrum β-lactamase production (ESBL) two antimicrobials were used as screening, ceftazidime – CAZ (10 μg) and cefotaxime-CTX (5 μg) (Antimicrobial disks, Interlab, São Paulo, Brazil), both third generation cephalosporins (W. Therapeutics Guideline Group, 2020). In addition, the isolates were characterized by their resistance to extended-spectrum b-lactamases (ESBL) by a double-disc synergy test (EUCAST, 2013). The results were classified according to the Clinical and Laboratory Standards Institute (2020), as sensitive (S), intermediate (I), or resistant (R) (Clinical and Laboratory Standards Institute, 2020).
The E. coli isolates were categorized as sensitive or resistant based on the inhibition zone. Isolates resistant to three or more classes of antimicrobials were classified as multidrug-resistant (MDR), which is defined as resistance to at least one agent in three or more antimicrobial categories. Extensively drug-resistant (XDR) was defined as resistance to at least one agent in all but two or fewer categories, while pandrug-resistant (PDR) was defined as resistance to all agents in all categories (Magiorakos et al., 2012) The positive reference standard strain used to ensure the accuracy and reliability of the tests was Escherichia coli ATCC 25922 (Biomedh, Minas Gerais, Brazil).
Statistical analysis
Descriptive statistics were used to characterize the frequency of E. coli and resistant isolates at each point. E. coli frequency results between the initial (Ca) and final point of process (Pf) were compared by chi-square. The chi-square test was also used to compare the MDR E. coli frequencies between fish-farms, for that, samples that presented at least one positive MDR isolate were considered positive. For all analysis the GraphPad Prisma 9.2.0 software was used (P<0.05). The figures were constructed using the RStudio (RStudio: Integrated Development Environment for R, 2024) packages ggplot2 (Chang et al., 2016) and UpSetR (Conway et al., 2017).
Results
A total of 50.8% of samples (122/240) were positive for E. coli. Despite the 31-percentage-point reduction in the frequency of E. coli between the initial (Ca) and final (Pf) stages of tilapia processing industry, all analyzed points had positive samples. Among the positive samples, 403 isolates were identified as E. coli by using biochemical tests (Table 2).
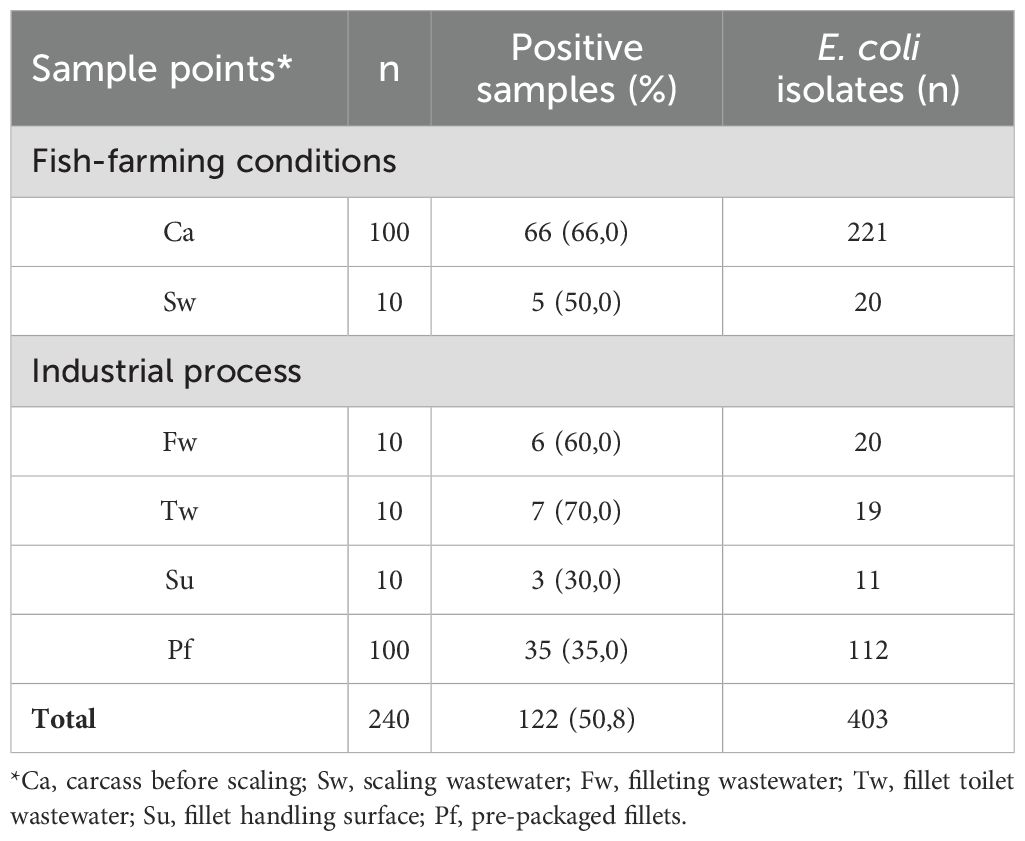
Table 2. Frequency of Escherichia coli and number of isolates from a tilapia processing unit in southwestern Brazil.
The antimicrobial susceptibility test showed that 36% (145/403) of the E. coli strains were susceptible to all tested antimicrobials and 33% (133/403) were resistant to at least two antimicrobials (Supplementary Table S1). The highest frequencies of resistance presented by the isolates were against amoxicillin (35.73%), tetracycline (30.77%) and ciprofloxacin (26.30%), respectively. It was observed that the E. coli was associated with lower resistance to gentamicin (1.99%), azithromycin (2.73%), and ceftiofur (2.98%), aztreonam (4.,71%) and imipenem (6.95%) (Table 3).
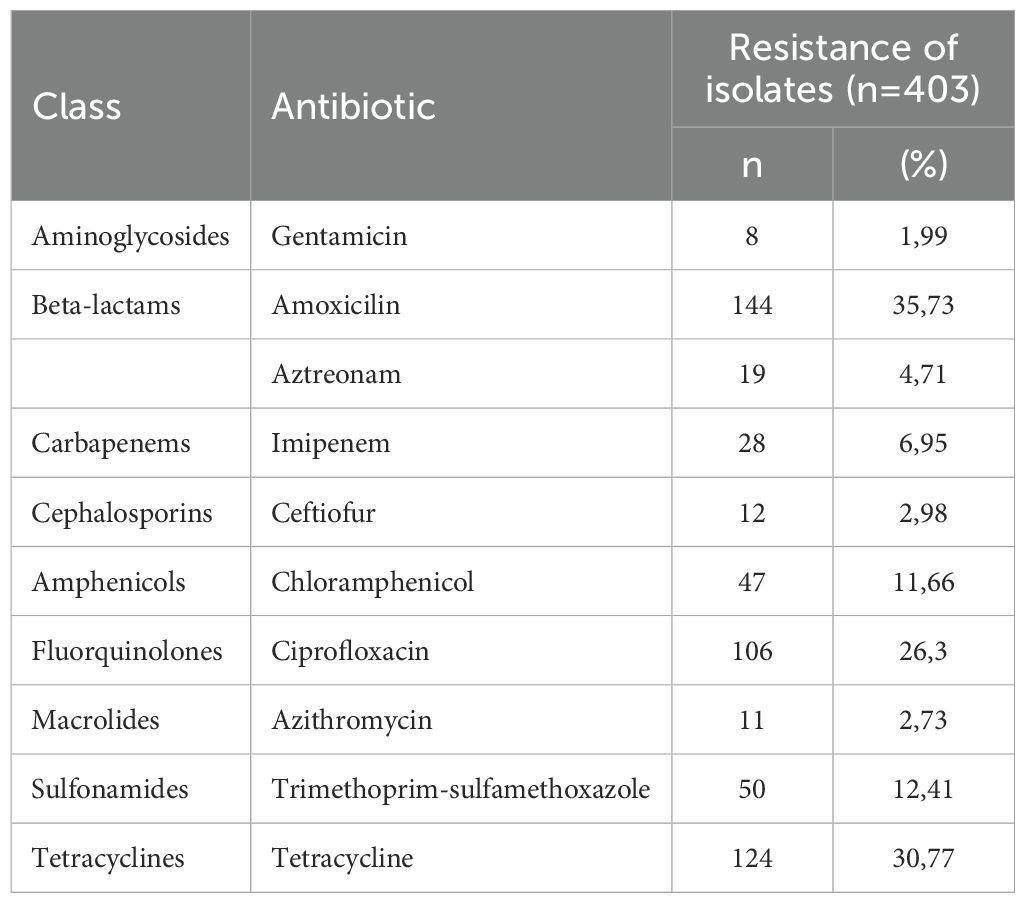
Table 3. Antimicrobial resistance of Escherichia coli obtained at different stages of a tilapia-processing industry located in southwestern Brazil.
Considering the results of resistance to the antimicrobials tested, it was possible to identify 20.0% (48/240) of samples with at least one MDR E. coli isolate (Figure 1A). The highest frequency of positive samples was obtained from representative points of the Fish-farming microbiological conditions (Ca and Sw) (chi-square test, p=0.0038, Figure 1A). However, this difference was only identified by the results of fish-farming C, which had 41.66% (15/36) of the MDR positive samples obtained from Ca and Sw points (Figure 1B). It is worth mentioning that these MDR positive samples were identified in two different visits to the slaughterhouse, five samples from a first batch and nine from a second one, showing a pattern of results from this fish-farming.
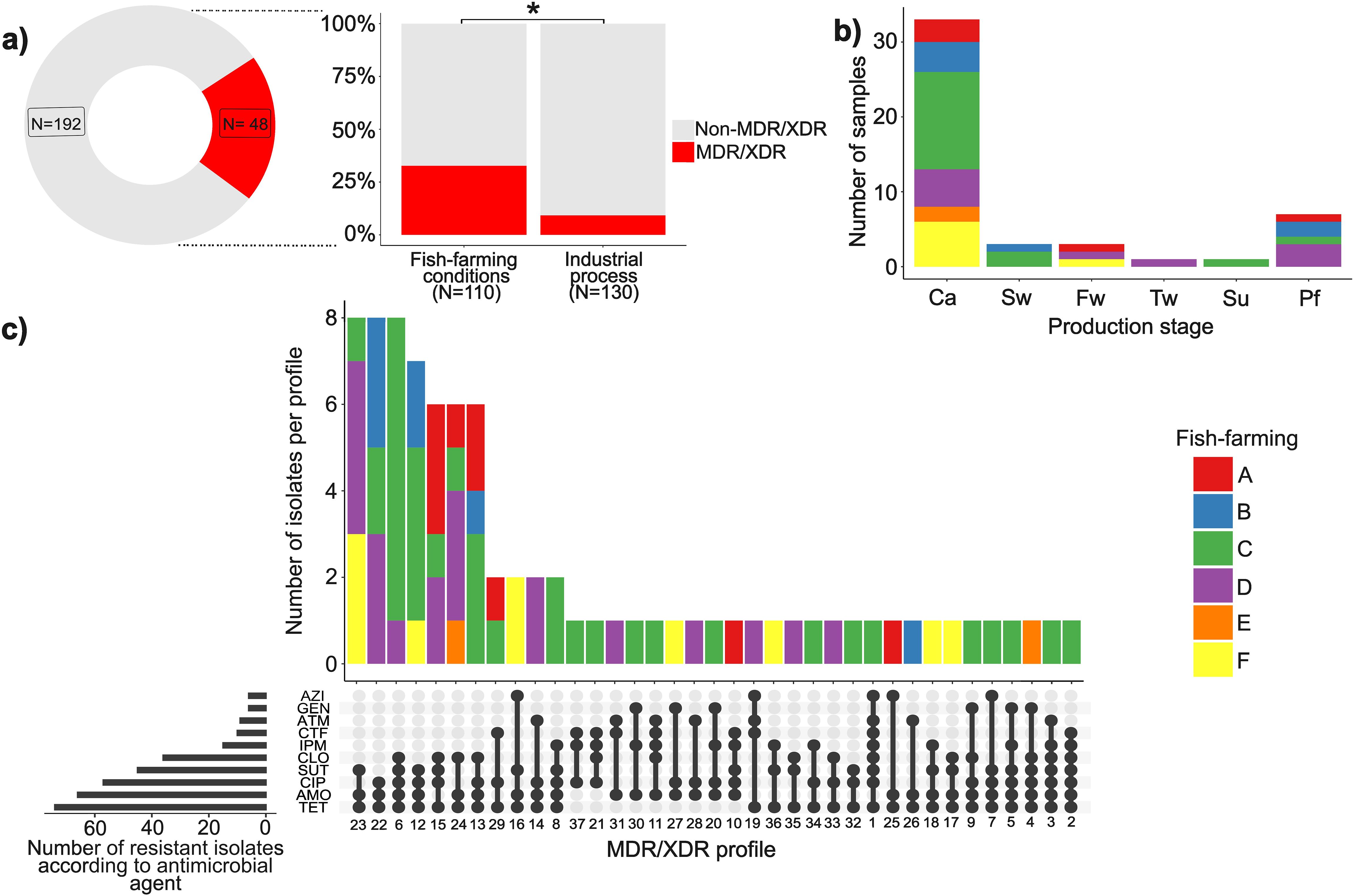
Figure 1. Multidrug resistance (MDR) and Extensively drug-resistant (XDR) of Escherichia coli obtained at different stages of a tilapia-processing industry located in southwesternBrazil. (A) Number of MDR/XDR samples by production stage. *Comparison between fish-farming conditions and industrial process using chi-square test (p<0.05). (B) Distribution of MDR/XDR samples according to the different production stages. Ca: carcass. Sw: scaling wastewater. Fw, filleting wastewater; Tw, fillet toilet wastewater; Su, fillet handling surface; Pf, pre-packaged fillets. (C) Distribution of isolates according to the 36 MDR and 1 XDR profiles identified. TETTetracycline; CIP-Ciprofloxacin; SUT-Sulfamethoxazole and trimethoprim; CLOChloramphenicol; AZI-Azithromycin; CTF-Ceftiofur; IPM-Imipenem; ATM-Aztreonam; AMOAmoxicillin; GEN-Gentamycin.
Analyzing the resistance results presented by each isolate, 62 profiles were identified (Supplementary Table S1). Of these, 58,06% (36/62) were considered MDR isolate profiles, ranging from 3 to 8 antimicrobial classes and 1.61% (1/62) was classified as an XDR isolate profile, exhibiting resistance to antimicrobials from 8 different classes (Figure 1C) and no samples were identified as PDR. Eleven resistance profiles were formed by five or more classes of antimicrobials, nine of which were exclusive or contained isolates from fish-farming C. Eleven profiles brought together more than one isolate, and eight had isolates from more than one fish-farming. Among the MDR resistance profiles, 36.14% (30/83) isolates showed simultaneous resistance to TET-CIP-SUT, 46.99% (39/83) showed resistance to AMO-TET-CIP, and 40.97% (34/83) showed resistance to TET-AMO-SUT.
Discussion
Considering all the collection points, E. coli was identified in a prevalence of 50.8%. A similar result was obtained by Dewi et al. (2022) in Malaysia, where 44.5% of tilapia samples were positive. Some studies indicate that E. coli does not occur naturally in the microbiota of fish, which is influenced by the habitat (Guzmán et al., 2004), and thus this pathogen is transferred to these animals by the environment and during handling. The reduction of positivity samples for E. coli between the initial (Ca) and the final collection point (Pf) shows that, despite the presence of the agent, the industry’s self-control programs have been efficient in reducing biological hazards. In this context, it is important to emphasize the role of the government as a supervisory agent and the industry as responsible for seeking a safety product for the consumer (Lupien, 2007; De Filippis et al., 2018; Preena et al., 2020). On the other hand, the presence of E. coli at all analysis points reinforces its important role as a sentinel microorganism for monitoring the resistance of microorganisms to antimicrobials (Mencía-Ares et al., 2022).
It is important to emphasize that antimicrobial resistance (AMR) refers to the ability of microorganisms to remain alive and active even in the presence of antimicrobial agents. These agents include antibiotics, disinfectants, and food preservatives, which are used to control microbial growth, inhibit their multiplication, or even eliminate them. Antimicrobials can be classified as natural, semi-synthetic, or synthetic and operate through various mechanisms, causing significant impacts on the microorganisms’ metabolic and physiological processes. For instance, β-lactams and glycopeptides affect cell wall synthesis, while macrolides and tetracyclines block protein production. Other antimicrobials, such as sulfonamides, interfere with metabolic pathways, and fluoroquinolones affect DNA replication and translation (Tenover, 2006; Varela et al., 2021).
In this context, the present study observed that while more than a third of the E. coli isolates were susceptible to all the antimicrobials tested, another third exhibited resistance to at least two drugs, thus being classified as multidrug-resistant (Jeong et al., 2007). Similar studies have demonstrated antimicrobial resistance in E. coli from fish. In Brazil, Rocha et al. (2014) analyzed 44 E. coli strains isolated from tilapias collected in markets, which showed low resistance to sulfamethoxazole-trimethoprim (4.54%) and tetracyclines (15.9%), with no isolates resistant to gentamicin, imipenem, or ciprofloxacin. In a study conducted in Malaysia, E. coli strains were isolated from tilapias within the production chain, with the authors finding 42.7% of isolates to be multidrug-resistant (MDR) (Dewi et al., 2022).
Resistance to tetracyclines (31.2%), chloramphenicol (12.7%), gentamicin (5.1%), and ceftiofur (0.0%) was similar to our findings, whereas resistance to ciprofloxacin (15.3%) was lower. In Bangladesh, Amin et al. (2024) conducted a study on 500 fish samples (tilapias and pangas) collected from the market, where the levels of resistance to chloramphenicol (7.0%) were similar to our findings, although resistance to ciprofloxacin (15.0%) was lower, and resistance to tetracyclines (40.0%), aztreonam (38.0%), gentamicin (9.0%), and sulfamethoxazole (38.0%) was higher (Amin et al., 2024). The levels of resistance in E. coli within fish production in African countries were analyzed in a meta-analysis by Moffo et al. (2024), which observed a high prevalence of MDR strains (43.1%) on the continent. Resistance to tetracyclines (66.4%), gentamicin (18.0%), and chloramphenicol (44.4%) was greater than what was observed in our study, while resistance to ciprofloxacin (15.1%) was lower (Moffo et al., 2024).
In addition to these data, studies are also concerned about the resistance against amoxicillin and tetracycline, which is a risk for the environment and future generations due to its widespread misuse (Weir et al., 2012). Resistance to tetracycline is considered frequent in most aquatic productions due to its wide use and corroborates the findings in the present study (Tuševljak et al., 2013). Conversely, the sensitivity of E. coli isolates to gentamicin and aztreonam, observed in our study, can be explained by the less common use of these drugs in aquaculture (Rocha et al., 2014).
Another important result was the E. coli isolates resistance to Chloraphenicol. This pharmacological base has been banned in animal production since 2003, because its residues constitute a risk to public health (MAPA, 2003). Despite this, an antimicrobial from the same class, florfenicol, is used to treat fish diseases, as it is an effective antimicrobial against a broad spectrum of pathogens (Botelho et al., 2015; Preena et al., 2020). The role of horizontal gene transfer in the dissemination of resistance should be considered as a possible explanation for our finding (Richardson et al., 2018) since some mechanisms of resistance to florfenicol also provide resistance to chloramphenicol (Schwarz et al., 2004; Pacheco-Silva et al., 2014). However, the literature suggests that this drug is used even after its legal ban (Pacheco-Silva et al., 2014). Therefore, the illegal use of this drug inducing resistance in the analyzed microbiome should be considered (Smith and Lewin, 1993; Miller and Harbottle, 2018).
Antimicrobial-resistant bacteria are currently one of the greatest challenges in human and veterinary medicine. In aquaculture, they are associated with the presence of residues in the aquatic environment and alterations in the local microbiome, contaminating fish and increasing the risk of resistant pathogens reaching humans. A major issue is that the limitations of therapies and the efficacy of pharmacological treatments are insufficient to combat resistance acquired by pathogenic microorganisms (Heuer et al., 2009).
Throughout their evolution, microorganisms have developed various mechanisms to resist the effects of antimicrobial agents, involving complex molecular and cellular systems. It is important to note that resistance to a single agent often leads to the development of resistance to multiple drugs in new variants. Consequently, multidrug-resistant bacteria can significantly compromise the effectiveness of treatments (Wright, 2011; Christaki et al., 2020).
Antibiotic resistance in bacteria can be classified into three types: intrinsic, acquired, and adaptive. Intrinsic resistance is linked to the natural characteristics of bacteria that make them inherently resistant to certain antibiotics. Acquired resistance, on the other hand, occurs when previously sensitive bacteria develop resistance due to genetic mutations or the incorporation of external genetic material via horizontal gene transfer (Holmes et al., 2016).
This process can occur through three main mechanisms: in transformation, bacteria absorb free DNA from the environment or under laboratory conditions and integrate it into their genome; in transduction, bacteriophages transfer DNA, including resistance genes, between bacteria during replication, spreading resistance; and in conjugation, resistance genes are transferred directly between bacteria through physical contact, aided by transferosomes and coupling proteins (Holmes et al., 2016; Munita and Arias, 2016).
Adaptive resistance, in contrast, arises in response to specific environmental signals such as stress or nutrient conditions. Unlike intrinsic and acquired resistances, which are permanent, adaptive resistance is temporary and reverts to the original state once the stimulus is removed. This type of resistance results from changes in gene expression, mediated by epigenetic modifications like DNA methylation, and involves the regulation of efflux pumps and porins (Salimiyan Rizi et al., 2018; Lee, 2019). Moreover, multidrug-resistant bacteria can transfer their resistance genes to other species in various environments, such as hospitals, the food industry, the human intestinal tract, and agriculture (Varela et al., 2021).
As occur in other parts of the world (Machowska and Stålsby Lundborg, 2018; Garcia et al., 2020), in Brazil it is relatively easy to purchase antimicrobials for use in animals, often without a veterinarian’s prescription (Garcia et al., 2020). Despite the existence of laws that state which drugs can be used, improvements still need to be done to curb this practice. Legislations that regulate the commercialization of drugs for veterinary use need to be created and improved, and adequate oversight needs to be implemented.
The higher frequency of MDR isolates observed at sample points related to fish-farming microbiological conditions reinforces this concern about the misuse of antimicrobials. This corroborates the fact that the indiscriminate use of antimicrobials in animal production is a concern and requires immediate changes, reinforcing international recommendations on surveillance and monitoring programs (Smith et al., 2013; Preena et al., 2020).
The higher consumption of antimicrobials is normally associated with intensive production systems, a condition that brings economic benefits but also increases the possibility of disease in tilapia (Jackson et al., 2020; Wencewicz, 2019). This occurs because the intensification of these systems exposes the animal to more stressors, weakening its immunological barriers. Knowing this reality, many producers carry out prophylaxis or metaphylaxis of their batch of fish, increasing the chance of developing multidrug-resistant bacteria (Wencewicz, 2019; Rigos et al., 2021). Despite this, it should be known that good practices in production and qualified technical support are ways to achieve an intensive production system with low consumption of antimicrobials (Rigos et al., 2021). These variations in the practices of each production system may explain the high frequency of MDR E. coli observed in fish-farming C, compared to other farms.
In addition to animal health problems, other challenges related to MDR bacteria are the risks of reaching humans through the food chain, and the impacts they may have on the environment (Islam et al., 2019). This requires a broad approach that directs the antibiotic use, with adequate and assisted indication in all links of the animal production chain (Manyi-Loh et al., 2018; de Alcântara Rodrigues et al., 2020).
The wide variation in resistance profiles found in our study reveals the variety of antimicrobials that may be used in the tilapia production. Furthermore, when animals are submitted to a challenge, their microbiome and the aquatic environment microbiome can cause the differences observed between isolates (Ho et al., 2000). The highest resistance profiles were found in E. coli isolated at sample points related to animal production, showing that the primary production is the bottleneck in tilapia production chain in relation to bacterial resistance.
The present study did not identify any extended-spectrum beta-lactamase-producing strain, unlike previous studies such as the one by Sivaraman et al. (2020). One possibility for this difference is the characteristic of local production exerting less selective pressure on the microbiome. Furthermore, the absence of the enzyme production phenotype does not rule out the possibility of strains presenting the gene and transmitting it to other bacteria present in the medium and, consequently, the risk to public health (Kazemian et al., 2019). However, a molecular analysis to answer this gap was not performed in this research.
The damage done by years of indiscriminate use of antibiotics cannot be undone, but alternatives already exist that can minimize their use. The use of herbal medicines appears as a natural alternative, non-aggressive to the environment and with antimicrobial properties (Valladão et al., 2015). Another strategy is the use of essential oils in the prevention and treatment of diseases in fish, contributing to the reduction of the use of antibiotics (Cunha et al., 2018). The use of vaccines reduces the use of antimicrobials and contributes to animal health in intensive production (Håstein et al., 2005). All these alternatives must also include good agricultural practices, water management, proper cleaning, proper disease diagnosis and improvement in infrastructure. Finally, probiotics are also an alternative to the use of antimicrobials, as they influence water quality, increase the immune response and antiviral effects (Balcazar et al., 2006).
Conclusion
The presence of E. coli in all stages of tilapia processing reinforces its importance as a sentinel microorganism for resistance surveillance. Furthermore, the high frequency of multidrug resistance isolates, especially in samples related to the microbiota of the fish-farming. The study warns about the risk to public health, animal health and the environment, reinforcing the importance of good practices in animal production and qualified technical support.
Data availability statement
The raw data supporting the conclusions of this article will be made available by the authors, without undue reservation.
Ethics statement
The manuscript presents research on animals that do not require ethical approval for their study.
Author contributions
SD: Writing – original draft, Project administration, Methodology, Investigation, Conceptualization. LC: Writing – review & editing, Visualization, Methodology, Investigation, Conceptualization. AB: Writing – review & editing, Visualization, Software, Formal analysis, Data curation. MC: Writing – review & editing, Visualization, Validation, Supervision, Project administration, Methodology, Investigation, Formal analysis, Data curation, Conceptualization. LN: Writing – review & editing, Visualization, Supervision, Project administration, Funding acquisition. RY: Writing – review & editing, Visualization, Methodology, Funding acquisition. LB: Writing – review & editing, Visualization, Validation, Resources. JP: Writing – review & editing, Visualization, Validation, Supervision, Resources, Project administration, Investigation, Funding acquisition, Formal analysis.
Funding
The author(s) declare financial support was received for the research, authorship, and/or publication of this article. The authors received financial support in part from the Coordination for the Improvement of Higher Education Personnel – Brazil (CAPES) – Finance Code 001 and the National Council for Scientific and Technological Development (CNPq) in Universal Call MCTIC/CNPq n° 28/2018.
Conflict of interest
The authors declare that the research was conducted in the absence of any commercial or financial relationships that could be construed as a potential conflict of interest.
Publisher’s note
All claims expressed in this article are solely those of the authors and do not necessarily represent those of their affiliated organizations, or those of the publisher, the editors and the reviewers. Any product that may be evaluated in this article, or claim that may be made by its manufacturer, is not guaranteed or endorsed by the publisher.
Supplementary material
The Supplementary Material for this article can be found online at: https://www.frontiersin.org/articles/10.3389/frabi.2024.1461662/full#supplementary-material
References
Algammal A. M., Alfifi K. J., Mabrok M., Alatawy M., Abdel-moneam D. A., Alghamdi S., et al. (2022). Newly Emerging MDR B. cereus in Mugil seheli as the First Report Commonly Harbor nhe, hbl, cytK, and pc-plc Virulence Genes and bla1, bla2, tetA, and ermA Resistance Genes. Infect. Drug Resist. 15, 2167–2185. doi: 10.2147/IDR.S365254
Amin M. B., Talukdar P. K., Sraboni A. S., Islam R., Mahmud Z. H., Berendes D., et al. (2024). Prevalence and antimicrobial resistance of major foodborne pathogens isolated from pangas and tilapia fish sold in retail markets of Dhaka city, Bangladesh. Int. J. Food Microbiol. 418, 110717. doi: 10.1016/j.ijfoodmicro.2024.110717
APHA. (2001). Compendium of methods for the microbiological examination of foods (Washington, DC: American Public Health Association), 676.
Balcazar J., Blas I., Ruizzarzuela I., Cunningham D., Vendrell D. (2006). The role of probiotics in aquaculture. Vet. Microbiol. 114, 173–186. doi: 10.1016/j.vetmic.2006.01.009
Baurer A. W., Kirby W. M., Sherris J. C. (1966). Antibiotic susceptibility testing by a standardized single disk method. Am. J. Clin. Pathol. 45, 493–496. doi: 10.1093/ajcp/45.4.493
Botelho R. G., Christofoletti C. A., Correia J. E., Ansoar Y., Olinda R. A., Tornisielo V. L. (2015). Genotoxic responses of juvenile tilapia (Oreochromis niloticus) exposed to florfenicol and oxytetracycline. Chemosphere 132, 206–212. doi: 10.1016/j.chemosphere.2015.02.053
Cabello F. C. (2006). Heavy use of prophylactic antibiotics in aquaculture: a growing problem for human and animal health and for the environment. Environ. Microbiol. vol, 1137–1144. doi: 10.1111/j.1462-2920.2006.01054.x
Camargo A. F. M., Amorim R. V. (2020). Resistance to antimicrobials in Escherichia coli isolated from fish in Brazilian aquaculture. Environmental Microbiology Reports 12, 67–72. doi: 10.1590/s2179-975x5519
Caputo A., Bondad-Reantaso M. G., Karunasagar I., Ahmed S. K. N. N. S., de Souza L. M., Goddard L. S., et al. (2023). Antimicrobial resistance in aquaculture: A global analysis of literature and national action plans. Rev. Aquac 15, 568–578. doi: 10.1111/raq.12741
Chang W., Henry L., Pedersen T. L., Takahashi K., Wilke C., Woo K., et al. (2016). ggplot2: elegant graphics for data analysis (Springer-Verlag New York).
Christaki E., Marcou M., Tofarides A. (2020). Antimicrobial resistance in bacteria: mechanisms, evolution, and persistence. J. Mol. Evol. 88, 26–40. doi: 10.1007/s00239-019-09914-3
Clinical and Laboratory Standards Institute (2018). CLSI M23-ED5:2018 development of in vitro susceptibility testing criteria and quality control parameters. (Wayne, PA: Clinical and Laboratory Standards Institute (CLSI)).
Clinical and Laboratory Standards Institute (2020). CLSI M100-ED30:2020 performance standards for antimicrobial susceptibility testing. (Wayne, PA: Clinical and Laboratory Standards Institute (CLSI)).
Conway J. R., Lex A., Gehlenborg N. (2017). UpSetR: an R package for the visualization of intersecting sets and their properties. Bioinformatics 33, 2938–2940. doi: 10.1093/bioinformatics/btx364
Croxen M. A., Law R. J., Scholz R., Keeney K. M., Wlodarska M., Finlay B. B. (2013). Recent advances in understanding enteric pathogenic escherichia coli. Clin. Microbiol. Rev. 26, 822–880. doi: 10.1128/CMR.00022-13
Cunha J. A., Heinzmann B. M., Baldisserotto B. (2018). The effects of essential oils and their major compounds on fish bacterial pathogens – a review. J. Appl. Microbiol. 125, 328–344. doi: 10.1111/jam.13911
Dawood M. A. O., Koshio S. (2016). Recent advances in the role of probiotics and prebiotics in carp aquaculture: A review. Aquaculture 454, 243–251. doi: 10.1016/j.aquaculture.2015.12.033
de Alcântara Rodrigues I., Ferrari R. G., Panzenhagen P. H. N., Mano S. B., Conte-Junior C. A. (2020). Antimicrobial resistance genes in bacteria from animal-based foods. Adv. App. Microbiol. 143, 183. doi: 10.1016/bs.aambs.2020.03.001
De Filippis F., Parente E., Ercolini D. (2018). Recent past, present, and future of the food microbiome. Annu. Rev. Food Sci. Technol. 9, 589–608. doi: 10.1146/annurev-food-030117-012312
De Toledo M. R. F. F. C. F. T. L. R. (1982). Mili - um meio para a realizacao dos testes de motilidade, indol e lisina descarboxilase. Rev. Microbiologia 13, 230–235. doi: 10.1590/S0034-75181982000400007
Dewi R. R., Rahman A. A., Abdul Rahman M. A., Mohd Noor A. S., Zainuddin M. S., Abdullah N. H. (2022). Prevalence and Antimicrobial Resistance of Escherichia coli, Salmonella and Vibrio Derived from Farm-Raised Red Hybrid Tilapia (Oreochromis spp.) and Asian Sea Bass (Lates calcarifer, Bloch 1970) on the West Coast of Peninsular Malaysia. Antibiotics 11, 136. doi: 10.3390/antibiotics11020136
Dhama K., Rajagunalan S., Chakraborty S., Verma A. K., Kumar A., Tiwari R., et al. (2013). Food-borne pathogens of animal origin-diagnosis, prevention, control and their zoonotic significance: A review. Pakistan J. Biol. Sci. 16, 1076–1085. doi: 10.3923/pjbs.2013.1076.1085
Food and Agriculture Organization of the United Nations (2020). The state of world fisheries and aquaculture 2020. (Rome, Italy: Food and Agriculture Organization of the United Nations (FAO)).
Garcia J. F., Jose Diez M., Sahagun A. M., Diez R., Sierra M., Garcia J. J., et al. (2020). The online sale of antibiotics for veterinary use. Animals 10, 503. doi: 10.3390/ani10030503
Greenlees K. J., MaChado J., Bell T., Sundlof S. F. (1998). Food borne microbial pathogens of cultured aquatic species. Veterinary Clinics North America: Food Anim. Pract. 14, 101–112. doi: 10.1016/S0749-0720(15)30282-6
Guzmán M. C., de los Angeles Bistoni M., Tamagnini L. M., González R. D. (2004). Recovery of Escherichia coli in fresh water fish, Jenynsia multidentata and Bryconamericus iheringi. Water Res. 38, 2368–2374. doi: 10.1016/j.watres.2004.02.016
Håstein T., Gudding R., Evensen O. (2005). Bacterial vaccines for fish–an update of the current situation worldwide. Dev. Biol. (Basel) 121, 55–74. doi: 10.1159/000086162
Heuer O. E., Kruse H., Grave K., Collignon P., Karunasagar I., Angulo F. J. (2009). Human health consequences of use of antimicrobial agents in aquaculture. Clin. Infect. Dis. 49, 1248–1253. doi: 10.1086/605667
Ho S.-P., Hsu T.-Y., Chen M.-H., Wang W.-S. (2000). Antibacterial effect of chloramphenicol, thiamphenicol and florfenicol against aquatic animal bacteria. J. Veterinary Med. Sci. 62, 479–485. doi: 10.1292/jvms.62.479
Holmes A. H., Moore L. S.P., Sundsfjord A., Steinbakk M., Regmi S., Karkey A., et al. (2016). Understanding the mechanisms and drivers of antimicrobial resistance. Lancet vol, 176–187. doi: 10.1016/S0140-6736(15)00473-0
Ibrahim G. A., Mabrok M., Alfifi K., Algammal A., Alatawy M., Alotaibi A., et al. (2024). Pathogenicity, resistance patterns, virulence traits, and resistance genes of re-emerging extensively drug-resistant (XDR) Aeromonas veronii in Oreochromis niloticus. Aquaculture Int. 32, 6987–7006. doi: 10.1007/s10499-024-01498-0
Islam M. A., Amin M. B., Roy S., Asaduzzaman M., Islam R., Navab-Daneshmand T., et al. (2019). Fecal colonization with multidrug-resistant E. coli among healthy infants in rural Bangladesh. Front. Microbiol. 10. doi: 10.3389/fmicb.2019.00640
Jackson J. A., Friberg I. M., Hablützel P. I., Masud N., Stewart A., Synnott R., et al. (2020). Partitioning the environmental drivers of immunocompetence. Sci. Total Environ. vol, 141152. doi: 10.1016/j.scitotenv.2020.141152
Jang J., Hur H.-G., Sadowsky M. J., Byappanahalli M. N., Yan T., Ishii S. (2017). Environmental Escherichia coli : ecology and public health implications-a review. J. Appl. Microbiol. 123, 570–581. doi: 10.1111/jam.13468
Jeong H.-Y., Lee J.-E., Choi B.-K., Seo K.-W., Park S.-H., Kim Y.-L., et al. (2007). Molecular Epidemiology of Community-Associated Antimicrobial-Resistant Staphylococcus aureus in Seoul, Korea (2003): Pervasiveness of Multidrug-Resistant SCC mec Type II Methicillin-Resistant S. aureus. Microbial Drug Resistance 13, 178–185. doi: 10.1089/mdr.2007.709
Kazemian H., Heidari H., Ghanavati R., Ghafourian S., Yazdani F., Sadeghifard N., et al. (2019). Phenotypic and Genotypic Characterization of ESBL-, AmpC-, and Carbapenemase-Producing Klebsiella pneumoniae and Escherichia coli Isolates. Med. Principles Pract. 28, 547–551. doi: 10.1159/000500311
Lee J.-H. (2019). Perspectives towards antibiotic resistance: from molecules to population. J. Microbiol. 57, 181–184. doi: 10.1007/s12275-019-0718-8
Lihan S., Lee S. Y., Toh S. C., Leong S. S. (2021). Plasmid-mediated antibiotic resistant escherichia coli in sarawak rivers and aquaculture farms, northwest of borneo. Antibiotics vol, 776. doi: 10.3390/antibiotics10070776
Lupien J. R. (2007). Prevention and control of food safety risks: the role of governments, food producers, marketers, and academia. Food Control 18, 1101–1106. doi: 10.1016/j.foodcont.2006.09.001
Mabrok M., Algammal A., El-Tarabili R. M., Rodkhum C. (2024). Enterobacter cloacae as a re-emerging pathogen affecting mullets (Mugil spp.): Pathogenicity testing, LD50, antibiogram, and encoded antimicrobial resistance genes. Aquaculture 583, 740619. doi: 10.1016/j.aquaculture.2024.740619
Machowska A., Stålsby Lundborg C. (2018). Drivers of irrational use of antibiotics in europe. Int. J. Environ. Res. Public Health 16, 27. doi: 10.3390/ijerph16010027
Magiorakos A.-P., Srinivasan A., Carey R. B., Carmeli Y., Falagas M. E., Giske C. G., et al. (2012). Multidrug-resistant, extensively drug-resistant and pandrug-resistant bacteria: an international expert proposal for interim standard definitions for acquired resistance. Clin. Microbiol. Infection 18, 268–281. doi: 10.1111/j.1469-0691.2011.03570.x
Manyi-Loh C., Mamphweli S., Meyer E., Okoh A. (2018). Antibiotic use in agriculture and its consequential resistance in environmental sources: potential public health implications. Molecules 23, 795. doi: 10.3390/molecules23040795
MAPA. (2003). instrucao-normativa-no-9-de-27-de-junho-de-2003. (Brasília, Brazil: Ministério da Agricultura, Pecuária Abastecimento (MAPA))
Mencía-Ares O., Borowiak M., Argüello H., Cobo-Díaz J. F., Malorny B., Álvarez-Ordóñez A., et al. (2022). Genomic insights into the mobilome and resistome of sentinel microorganisms originating from farms of two different swine production systems. Microbiol. Spectr. 10, 159–164. doi: 10.1128/spectrum.02896-22
Miller R. A., Harbottle H. (2018). Antimicrobial drug resistance in fish pathogens. Microbiol. Spectr. 6, 497–509. doi: 10.1128/microbiolspec.ARBA-0017-2017
Moffo F., Ndebé M. M. F., Tangu M. N., Noumedem R. N. G., Awah-Ndukum J., Mouiche M. M. M. (2024). Antimicrobial use, residues and resistance in fish production in Africa: systematic review and meta-analysis. BMC Vet. Res. 20, 307. doi: 10.1186/s12917-024-04158-w
Moura R. S. T., Valenti W. C., Henry-Silva G. G. (2016). Sustainability of Nile tilapia net-cage culture in a reservoir in a semi-arid region. Ecol. Indic 66, 574–582. doi: 10.1016/j.ecolind.2016.01.052
Munita J. M., Arias C. A. (2016). “Mechanisms of antibiotic resistance,” in Virulence mechanisms of bacterial pathogens (ASM Press, Washington, DC, USA), 481–511. doi: 10.1128/9781555819286.ch17
Ng C., Chen H., Goh S. G., Haller L., Wu Z., Charles F. R., et al. (2018). Microbial water quality and the detection of multidrug resistant E. coli and antibiotic resistance genes in aquaculture sites of Singapore. Mar. pollut. Bull. 135, 475–480. doi: 10.1016/j.marpolbul.2018.07.055
Pacheco-Silva É., de Souza J. R., Caldas E. D. (2014). Resíduos de medicamentos veterinários em leite e ovos. Quim Nova 37, 111–122. doi: 10.1590/S0100-40422014000100020
Pedrini B., Sperche C., Dellova D., Medeiros F., Souza F., Oliveira G., et al. (2023). ANUÁRIO PEIXE BR DA PISCICULTURA 2023 ANUÁRIO 2023 peixe BR da piscicultura (Brasília, Brazil: Associação Brasileira de Piscicultura (ABP)).
Poirel L., Madec J.-Y., Lupo A., Schink A.-K., Kieffer N., Nordmann P., et al. (2018). Antimicrobial resistance in escherichia coli. Microbiol. Spectr. 6, 268–273. doi: 10.1128/microbiolspec.ARBA-0026-2017
Preena P. G., Dharmaratnam A., Raj N. S., Raja S. A., Nair R. R., Swaminathan T. R. (2021). Antibiotic-resistant Enterobacteriaceae from diseased freshwater goldfish. Arch. Microbiol. 203, 219–231. doi: 10.1007/s00203-020-02021-8
Preena P. G., Swaminathan T. R., Kumar V. J. R., Singh I. S. B. (2020). Antimicrobial resistance in aquaculture: a crisis for concern. Biol. (Bratisl) 75, 1497–1517. doi: 10.2478/s11756-020-00456-4
Richardson E. J., Bacigalupe R., Harrison E. M., Weinert L. A., Lycett S., Vrieling M. (2018). Gene exchange drives the ecological success of a multi-host bacterial pathogen. Nat. Ecol. Evol. 2, 1468–1478. doi: 10.1038/s41559-018-0617-0
Rigos G., Kogiannou D., Padrós F., Cristòfol C., Florio D., Fioravanti M., et al. (2021). Best therapeutic practices for the use of antibacterial agents in finfish aquaculture: a particular view on European seabass (Dicentrarchus labrax) and gilthead seabream (Sparus aurata) in Mediterranean aquaculture. Rev. Aquac 13, 1285–1323. doi: 10.1111/raq.12523
Rocha R.d. S., Leite L. O., de Sousa O.V., Vieira R.H.S.d. (2014). Antimicrobial Susceptibility of Escherichia coli Isolated from Fresh-Marketed Nile Tilapia (Oreochromis niloticus). J. Pathog. 2014, 1–5. doi: 10.1155/2014/756539
Salimiyan Rizi K., Ghazvini K., Noghondar M.K. (2018). Adaptive antibiotic resistance: overview and perspectives. J. Infect. Dis. Ther. 06, 86–90. doi: 10.4172/2332-0877.1000363
Schwarz S., Kehrenberg C., Doublet B., Cloeckaert A. (2004). Molecular basis of bacterial resistance to chloramphenicol and florfenicol. FEMS Microbiol. Rev. vol, 519–542. doi: 10.1016/j.femsre.2004.04.001
Sivaraman G. K., Sudha S., Muneeb K. H., Shome B., Holmes M., Cole J. (2020). Molecular assessment of antimicrobial resistance and virulence in multi drug resistant ESBL-producing Escherichia coli and Klebsiella pneumoniae from food fishes, Assam, India. Microb. Pathog. vol, 104581. doi: 10.1016/j.micpath.2020.104581
Smith P. G. (2020). La resistencia a los antimicrobianos en acuicultura. Rev. Scientifique Technique l’OIE 27, 243–264. doi: 10.20506/rst.27.1.1799
Smith P. G., Alday-Sanz V., Matysczak J., Moulin G., Lavilla-Pitogo C. R., Prater D. A. (2013). [amp]]ldquo;Monitoring and surveillance of antimicrobial resistance in microorganisms associated with aquatic animals. Rev. Scientifique Technique l’OIE 32, 583–593. doi: 10.20506/rst.32.2.2237
Smith J. T., Lewin C. S. (1993). Mechanisms of antimicrobial resistance and implications for epidemiology. Vet. Microbiol. 35, 233–242. doi: 10.1016/0378-1135(93)90148-Z
Tenover F. C. (2006). Mechanisms of antimicrobial resistance in bacteria. Am. J. Infect. Control 34, S3–S10. doi: 10.1016/j.ajic.2006.05.219
Tuševljak N., Dutil L., Rajić A., Uhland F. C., McClure C., St-Hilaire S., et al. (2013). Antimicrobial use and resistance in aquaculture: findings of a globally administered survey of aquaculture-allied professionals. Zoonoses Public Health 60, 426–436. doi: 10.1111/zph.12017
Valladão G. M. R., Gallani S. U., Pilarski F. (2015). Phytotherapy as an alternative for treating fish disease. J. Vet. Pharmacol. Ther. 38, 417–428. doi: 10.1111/jvp.12202
Van Boeckel T. P., Glennon E. E., Chen D., Gilbert M., Robinson T. P., Grenfell B. T., et al. (2017). Reducing antimicrobial use in food animals. Sci. (1979) 357, 1350–1352. doi: 10.1126/science.aao1495
Varela M. F., Stephen J., Lekshmi M., Ojha M., Wenzel N., Sanford L. M., et al. (2021). Bacterial resistance to antimicrobial agents. Antibiotics 10, 593. doi: 10.3390/antibiotics10050593
Walker D. I., Younger A., Stockley L., Baker-Austin C. (2018). Escherichia coli testing and enumeration in live bivalve shellfish – Present methods and future directions. Food Microbiol. 73, 29–38. doi: 10.1016/j.fm.2017.12.006
Weir M., Rajić A., Dutil L., Cernicchiaro N., Uhland F. C., Mercier B., et al. (2012). Zoonotic bacteria, antimicrobial use and antimicrobial resistance in ornamental fish: a systematic review of the existing research and survey of aquaculture-allied professionals. Epidemiol. Infect. 140, 192–206. doi: 10.1017/S0950268811001798
Wencewicz T. A. (2019). Crossroads of antibiotic resistance and biosynthesis. J. Mol. Biol. 431, 3370–3399. doi: 10.1016/j.jmb.2019.06.033
Wright G. D. (2011). Molecular mechanisms of antibiotic resistance. Chem. Commun. 47, 4055. doi: 10.1039/c0cc05111j
W. Therapeutics Guideline Group (2020). List of essential medicines for cats and dogs members of the WSAVA therapeutic guidelines group (TGG). Available online at: https://www.who.int/medicines/publications/essentialmedicines/en/ (accessed April 2024).
Keywords: antibiotics, E. coli, microbial sensitivity, tilapia, resistance
Citation: Dias SDC, Costa LRM, Buiatte ABG, Cossi MVC, Nero LA, Yamatogi RS, Bersot LdS and Pereira JG (2024) Escherichia coli as a sentinel in the assessment of antimicrobial resistance in the tilapia production chain: from production environment to the final product. Front. Antibiot. 3:1461662. doi: 10.3389/frabi.2024.1461662
Received: 08 July 2024; Accepted: 30 September 2024;
Published: 22 October 2024.
Edited by:
Md Asadulghani, International Centre for Diarrhoeal Disease Research (ICDDR), BangladeshReviewed by:
Santosh Dulal, Management Sciences for Health, United StatesMahmoud Mabrok, Suez Canal University, Egypt
Copyright © 2024 Dias, Costa, Buiatte, Cossi, Nero, Yamatogi, Bersot and Pereira. This is an open-access article distributed under the terms of the Creative Commons Attribution License (CC BY). The use, distribution or reproduction in other forums is permitted, provided the original author(s) and the copyright owner(s) are credited and that the original publication in this journal is cited, in accordance with accepted academic practice. No use, distribution or reproduction is permitted which does not comply with these terms.
*Correspondence: Letícia Roberta Martins Costa, bGV0aWNpYW1hcnRpbnN2ZXRAZ21haWwuY29t