- 1Yunnan Provincial Key Laboratory of Animal Nutrition and Feed Science, Faculty of Animal Science and Technology, Yunnan Agricultural University, Kunming, China
- 2Changchun Nanguan District Changtong Community Health Service Center, Changchun, China
- 3WOD Poultry Research Institute, Beijing, China
- 4College of Veterinary Medicine, Yunnan Agricultural University, Kunming, China
Introduction: The aim of this study was to investigate effect of energy levels on liver oxidative state and gut microbiota of laying hens fed a low-protein diet.
Methods: A total of 216 laying hens (57-week-old with similar body weight) were randomly divided into 3 groups: low-energy and low-protein diet (LL: 10.73 MJ/kg), middle-energy and low-protein diet (ML: 11.15 MJ/kg), and high-energy and low-protein diet (HL: 11.57 MJ/kg) groups. The experiment lasted for 10 weeks.
Results: Results showed that the liver MDA level was higher, while the liver T-AOC level was lower in the HL group compared with LL and ML groups. The dominant phyla in LL group, ML group, and HL group were Bacteroidetes (38.08%, 39.69%, and 40.93%) and Firmicutes (16.78%, 18.37%, and 17.44%). At the genus level, Alistipes (9.45%) was abundant in the LL group. Bacteroides (14.46%), Phocaeicola (4.48%) and Precotella (2.95%) were highest in the ML group. Parabacteroides (1.78%) and Desulfovibrio (1.02%) were highest in the HL group. At the species level, Bacteroides fragilis (0.42%) is lowest in LL group, Desulfovibrio piger increased with the increase of energy. MDA was significantly and positively correlated with Methanobrevibacter woesei between ML group and HL groups (p < 0.05). T-AOC was highly significantly and positively correlated with Phocaeicola sp. Sa1YUN3 (p < 0.01) .
Discussion: This study found that high-energy and low-protein diets might cause liver oxidative stress by gut microbes in the laying hens.
1 Introduction
Feed is a major input cost in poultry production, accounting for up to 70% of the total production costs (Thirumalaisamy et al., 2016). Soybean meal and corn are the main ingredients in commercial poultry diets that provide crude protein and energy. However, the current feedstuffs supply cannot fulfill the needs of livestock production (Cao and Li, 2013). A low-protein diet is based on the theory of protein amino acid nutritional balance and reduces protein levels and nitrogen emissions by adding appropriate types and quantities of industrial amino acids, which could decrease production costs. However, the inappropriate energy levels with low protein diet can lead to lipid metabolism disorders and oxidative stress in the liver of laying hens (especially for late-laying hens), decreasing egg yield and quality (Ampong et al., 2020; Scappaticcio et al., 2022; Zutphen et al., 2016).
When laying hens enter the late-laying stage, large amounts of lipids accumulate in the liver. Oxidative stress is the key factor of liver lipid accumulation, resulting in liver function reduction, which may lead to hen tissue damage. This phenomenon leads to a decrease in the production performance and egg quality of laying hens in the late laying period (Liu et al., 2018). The gastrointestinal tract is the major site of food digestion and nutrient absorption (Yan et al., 2017). The gut microbiota profoundly affects many metabolic processes in the host, including energy homeostasis, lipid metabolism, and immune system development (Sonnenburg and Bäckhed, 2016; Sommer and Bäckhed, 2016). The cecum is the main site for intestinal microorganisms, and manipulation of the cecum microbiota of chickens might increase their productivity. Most chicken microbiota studies have focused on microbial communities in the cecum.
The gut microbiota is closely related to hepatic function via the gut-liver axis (Konturek et al., 2018). In the gut-liver axis, the liver and gut functions regulate each other, and they are both affected by diet (Ringseis et al., 2020; Albillos et al., 2020). Dietary composition is the most crucial factor for maintaining the growth of microbes, and changing the ingredients of the diet can directly affect the intestinal microbiota in the host (Abdollahi et al., 2018; Crisol-Martínez et al., 2017; Beam et al., 2021). Additionally, diet energy levels that do not fulfill the nutritional needs will lead to oxidative stress in the liver (Tan et al., 2018). At present, there are few reports on the effects of low-protein diets with different energy levels on liver oxidative stress and intestinal composition in the late laying period. In this study, we conducted a correlation analysis of liver antioxidant capacity and intestinal microbial composition in laying hens during the late laying period. To achieve this objective, first, we fed late laying hens different energy levels with the same low-protein diet content. Next, we detected the antioxidant capacity of the liver and performed a metagenomic gene sequencing analysis of the gut microbiomes of the three groups of laying hens. Finally, evaluated the differences in liver antioxidant capacity and gut microbiome composition of these three groups of laying hens to understand the optimal dietary energy density of laying hens.
2 Materials and methods
2.1 Animals and sample collection
Experiment was conducted on a sample of 216 Peking Pink laying hens (YunLingGuangDaYukou Poultry Co., Ltd., Yunnan, China) at 57 weeks of age, with similar body weights and consistent production performance. The sample was randomly divided into 3 groups of 6 replicates each and12 hens in each replicate. The diets were prepared by referring to the National Research Council (NRC 1994). The protein level was reduced by 2% to 14.29%. The dietary energy levels were low, 10.72 MJ/kg; middle, 11.14 MJ/kg; and high, 11.56 MJ/kg (Table 1). The other nutritional levels and feeding conditions were the same for a pre-test period of 1 week and a test period of 10 weeks, with feeding limited to 112 g daily; water was provided ad libitum throughout the trial period. At the end of experiment, six hens (one hens from every replicate) from every group were slaughter. The liver and cecum chyme samples were collected and immediately snap-frozen in liquid nitrogen. Samples were stored at -80 °C until use. All animal experiments were approved by the Institutional Animal Care and Use Committee of the Yunnan Agricultural University (No. 202202001).
2.2 Measurement of oxidative stress indices in liver
Colorimetric determination of liver malondialdehyde (MDA), total antioxidant capacity (T-AOC), total superoxide dismutase (T-SOD), glutathione (GSH), and glutathione peroxidase (GSH-Px). The determination steps were conducted per the manufacturer’s instructions (Nanjing Jiancheng Bioengineering Institute, Nanjing, China).
2.3 DNA extraction
Sample DNA was extracted by Guangdong Magigene Biotechnology Co. Ltd. (Guangzhou, China) using commercial kits per the manufacturer’s instructions. DNA integrity and purity were monitored using 1% agarose gel electrophoresis. The DNA concentration and purity were assessed using Qubit 3.0 (Thermo Fisher Scientific, Waltham, USA) and NanoDrop One (Thermo Fisher Scientific).
2.4 Database construction and metagenomic sequencing
Sequencing libraries were generated using the ALFA-SEQ DNA Library Prep Kit, and indexing codes were added. Library quality was evaluated using a Qubit 4.0 fluorometer (Life Technologies, Grand Island, NY, USA) and a QSEP400 high-throughput nucleic acid protein analysis system (Houze Biology Technology Co., China). Finally, the libraries were sequenced on the Illumina Novaseq 6000 platform, and 150 bp double-ended reads were obtained.
2.5 Statistical analysis
The statistical analysis of liver oxidative stress-related indicators was performed using SPSS with one-way ANOVA. Tukey test was used and p < 0.05 was regard as significance.
To obtain clean data, we used Trimmomatic (v.0.36) to process the original raw data. Based on the compositional spectra of the underlying functional units annotated at the phylum, genus, and species levels for each sample, the number of shared and unique species was calculated and visualized using Venn diagrams. Principal component analysis (PCA) is based on Euclidean distance and uses variance decomposition to find the principal components (eigenvalues) and their contributions that cause differences between samples. The linear discriminant analysis effect size (LEfSe) was used to determine the bacterial taxa that were statistically and biologically responsible for these differences. The Kyoto Encyclopedia of Genes and Genomes (KEGG, http://www.kegg.jp/kegg/) databases were used for the functional annotations. Statistical significance was set at p < 0.05.
3 Results
3.1 Oxidative stress-related indicators of liver tissue
The activity of the antioxidative enzyme and MDA levels in the liver of the hens is shown in Figure 1. Compared with LL and ML groups, the liver MDA level was higher, while the liver T-AOC level was lower in the HL group (Figure 1).
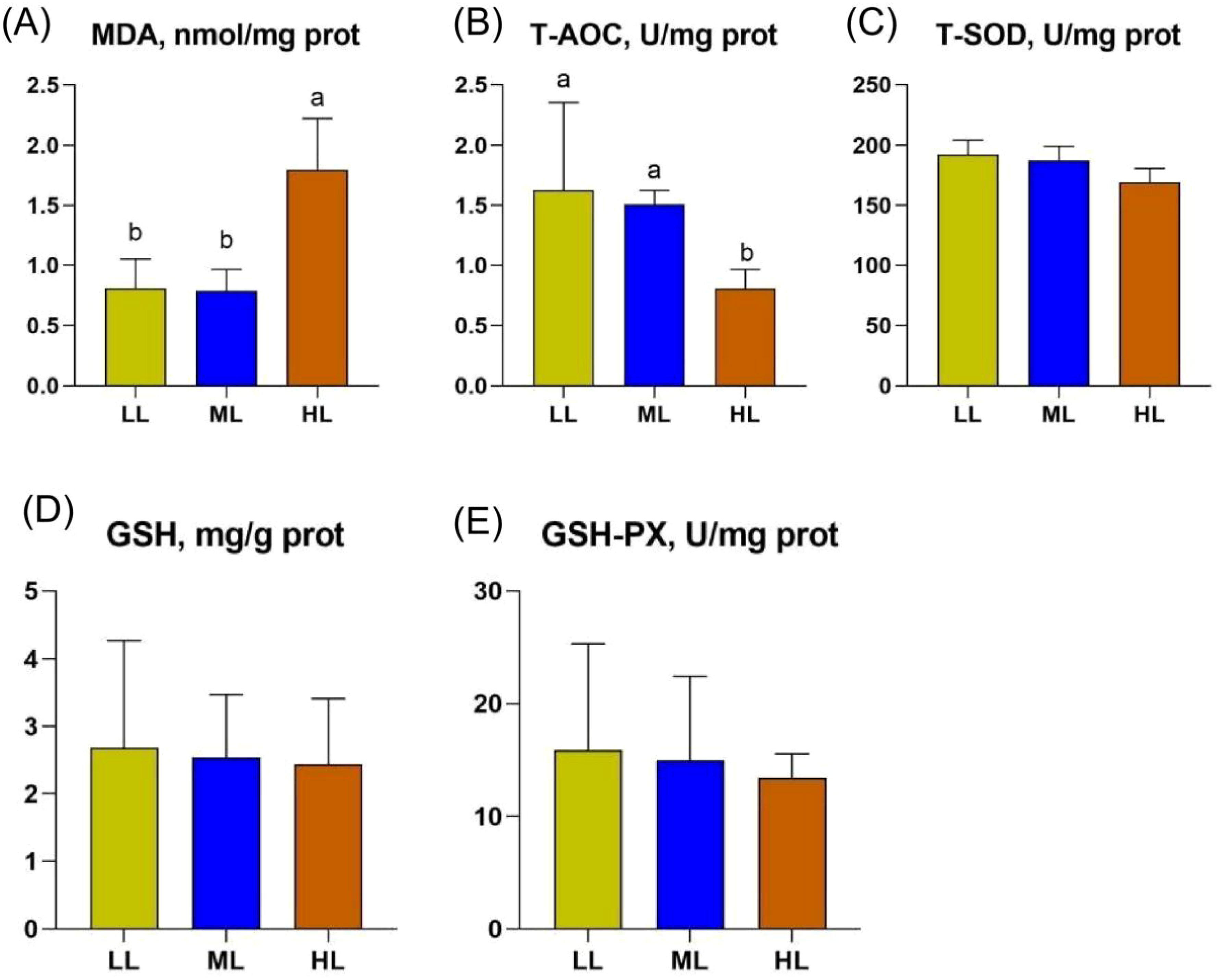
Figure 1. Illustrates the effect of three different low-protein dietary energy levels on liver MDA, T-AOC, T-SOD, GSH and GSH-PX in late-laying hens. (A) The content of MDA in liver of three groups. (B) The content of T-AOC in liver of three groups. (C) The content of T-SOD in liver of three groups. (D) The content of GSH in liver of three groups. (E) The content of GSH-PX in liver of three groups. Different letters in the superscripts (A, B) of the same row indicate significant differences (p < 0.05).
3.2 Sequencing data quality statistics
Eighteen cecal samples from the three groups generated 1,303,157,764 clean reads, with an average of 72,397,654 clean reads per sample. The proportion of bases with a sequencing error rate of less than 1% (Q30) in the valid data species reached more than 99%, indicating the high reliability of the sequencing data (Table 2).
3.3 Changes in diversity of microbial community in cecum
A Venn diagram revealed the three groups’ shared and unique microbial percentages. At the phylum level, 213 core OTUs were identified in the 3 groups, 4 core OTUs were identified in the LL and HL groups, and 1 unique OTU was identified in the HL group (Figure 2A). At the genus level, 3744 core OTUs were identified in the 3 groups; 93 core OTUs were identified in the LL and ML groups; 64 core OTUs were identified in the LL and HL groups; 71 core OTUs were identified in the ML and HL groups; and 70, 27, and 33 unique OTUs were identified in the LL, ML, and HL groups, respectively (Figure 2B). At the species level, 19309 core OTUs were identified in the 3 groups; 836 core OTUs were identified in the LL and ML groups; 731 core OTUs were identified in the LL and HL groups; 547 core OTUs were identified in the ML and HL groups; and 501,234, and 239 unique OTUs were identified in the LL, ML, and HL groups, respectively (Figure 2C).
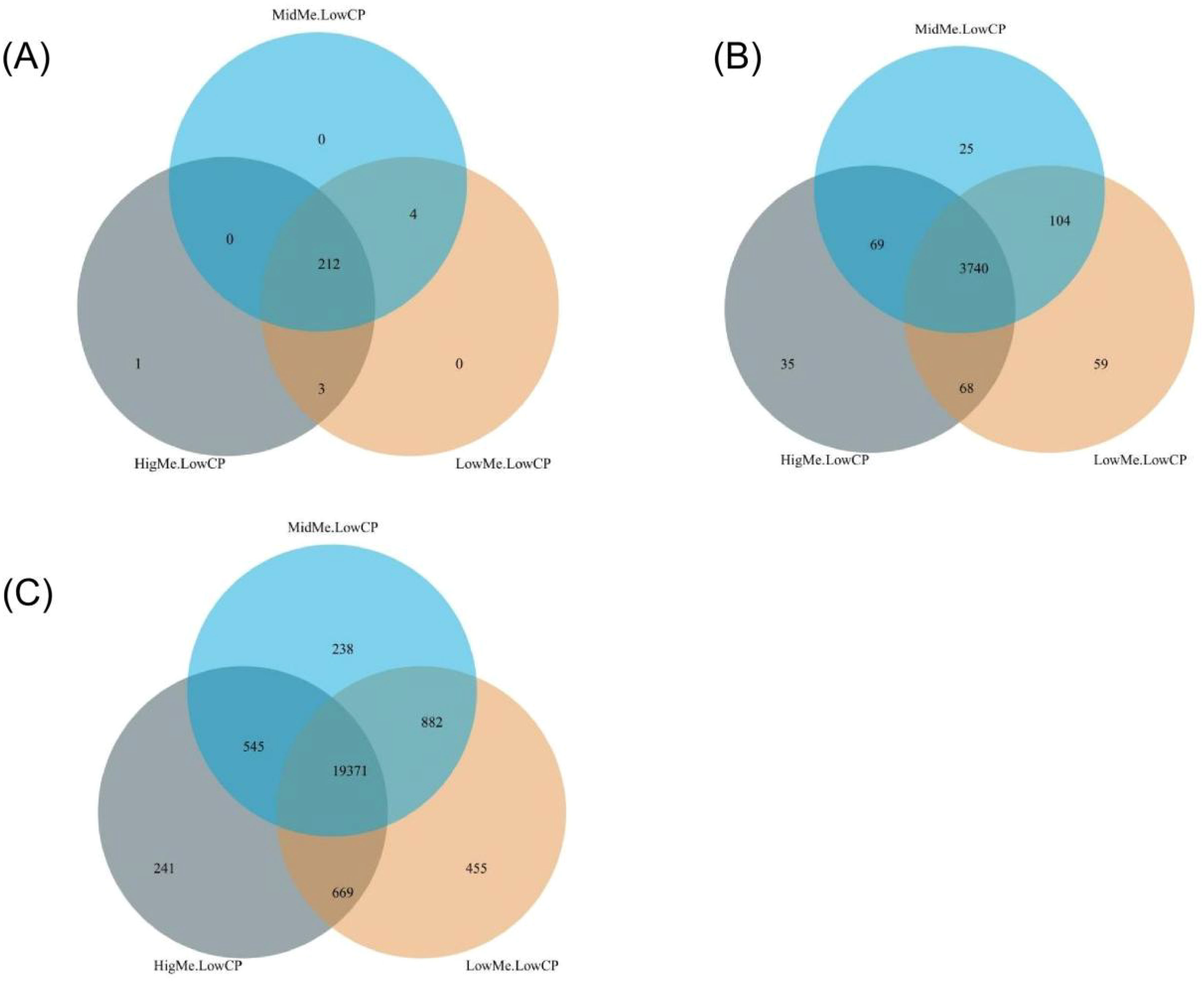
Figure 2. Venn diagram of microbial OTUs clustered at identity in LL, ML and HL groups, the overlapping area represent shared OTUs numbers between different groups; (A) Venn diagram at the phylum level; (B) Venn diagram at genus level; (C) Venn diagram at species level.
3.4 Microbiota diversity analysis
PCA was used to estimate beta diversity among the three groups. The PCA plot of unweighted UniFrac distances showed no distinct differences among the three groups (p > 0.05; Figures 3A–C).
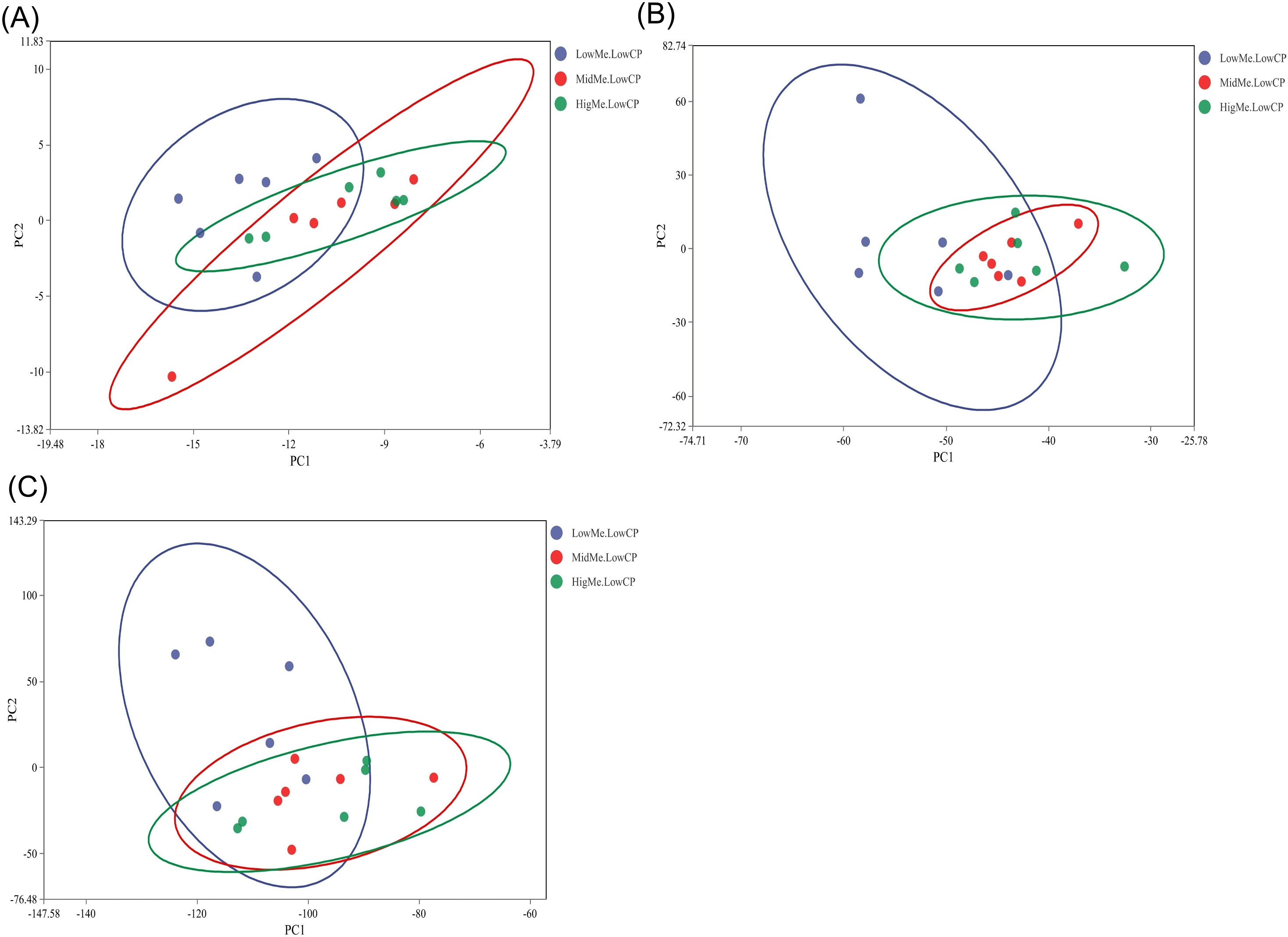
Figure 3. Principal component analysis (PCA) of cecal microbial beta-diversity in three groups (black denotes the LL group, yellow denotes the ML group and blue denotes the HL group). The percentage of the variation explained by the plotted principal coordinates is indicated on the axes. (A) PCA plot at the phylum level; (B) PCA plot at the genus level; (C) PCA plot at the species level.
3.5 Microbial composition in the cecum of the three groups
We assessed the relative abundances among all treatment groups at the phylum, genus, and species levels. At the phylum level, Bacteroidetes and Firmicutes were the two types of bacteria that accounted for the largest proportion in the cecum of the laying hens, exceeding 50% of the total cecum bacteria (Figure 4A). Bacteroidetes was the most abundant in the HL group. At the genus level, Bacteroides, Alistipes, Phocaeicola, and Prevotella accounted for greater than 30% of the total cecal bacteria. Alistipes was abundant in the LL group. Desulfovibrio was the most abundant in the HL group (Figure 4B). At the species level, Methanobrevibacter woesei increased with increasing energy levels. Bacteroides togonis and Mediterranea sp. An20 were the most abundant in the ML group. Phocaeicola plebeius and Alistipes sp. CAG:831 were most abundant in the LL group (Figure 4C).
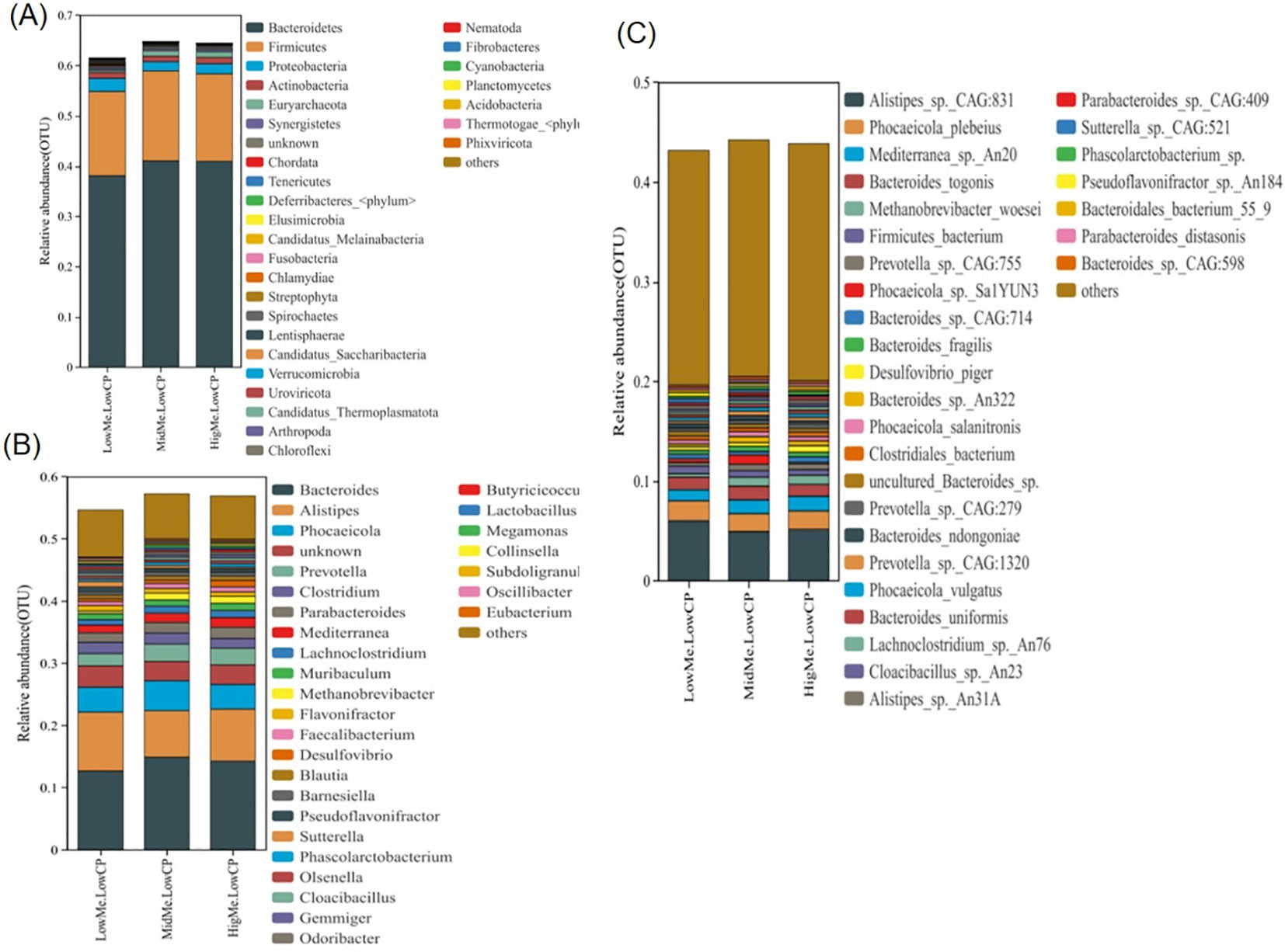
Figure 4. The effects of different energy level in low protein diets on the relative abundance in the cecal microbiota of laying hens. (A) Relative abundance of gut microbiota at the phylum level; (B) Relative abundance of gut microbiota at the genus level; (C) Relative abundance of gut microbiota at the species level.
3.6 Analysis of species differences among the three groups
The LEfSe analysis is an analytical tool for discovering and interpreting high dimensional data biomarkers. Using the LEfSe, we compared the cecal microbiotas of these groups.
Compared with those of the ML group, in the HL group, Limosilactobacillus mucosae, Alistipes sp. CAG 268, Alistipes sp. 58 9 plus, Bacteroidales bacterium 55 9, and Alistipes dispar were enriched in HL group (Figure 5A); Clostridia bacterium, Alistipes timonensis, Clostridium sp. CAG 306, Candidatus Gastranaerophilales bacterium, Clostridium sp. CAG 768, Flavonifractor plautii, Fusobacterium sp. CAG 439, Acetobacter sp. CAG 267, Brachyspira sp. CAG 484, Mailhella massiliensis, Alistipes dispar, and Alistipes sp. An66 were enriched in the LL group (Figure 5B). Compared with those of the HL group, Sutterella sp. CAG 521, Sutterella sp. AM11 39, Mailhella massiliensis, Acetobacter sp. CAG 267, Brachyspira sp. CAG 484, Fusobacterium sp. CAG 439, Flavonifractor plautii, Candidatus Gastranaerophilales bacterium, Clostridium sp. CAG 768, Clostridium sp. CAG 306, Flavonifractor sp. An100, and Intestinimonas massiliensis were enriched in the LL group (Figure 5C).
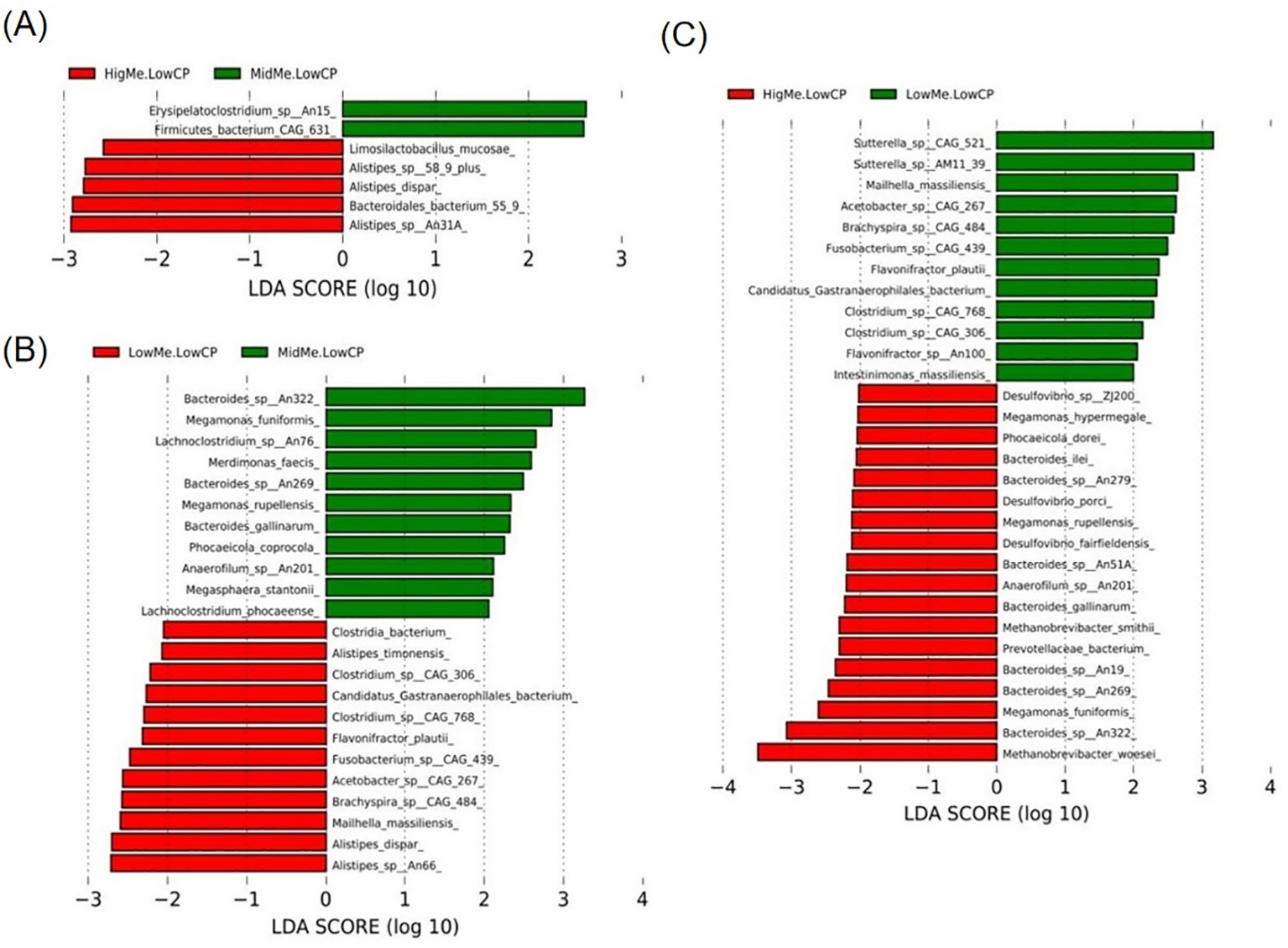
Figure 5. Linear discriminant analysis effect size (LEfSe) analysis based on OTUs characterizes microbiota between three groups. Histogram of the linear discriminant analysis (LDA) scores computed for features differentially abundant (significant threshold > 2 fold and p < 0.05) among hens was shown. (A) HL group * ML group; (B) LL group * ML group; (C) HL group * LL group.
3.7 Analysis of functional differences among the three groups
Of the three groups, in the HL group, the Infectious disease viral pathway was significantly enriched at the KEGG L2 level (Figure 6A), and the Porphyrin metabolism pathway was significantly enriched at the KEGG L3 level (Figure 6B). The Bray–Curtis s dissimilarity and analysis of molecular variance demonstrated that no structural differences among the three groups significantly differed at the L1 and family levels.
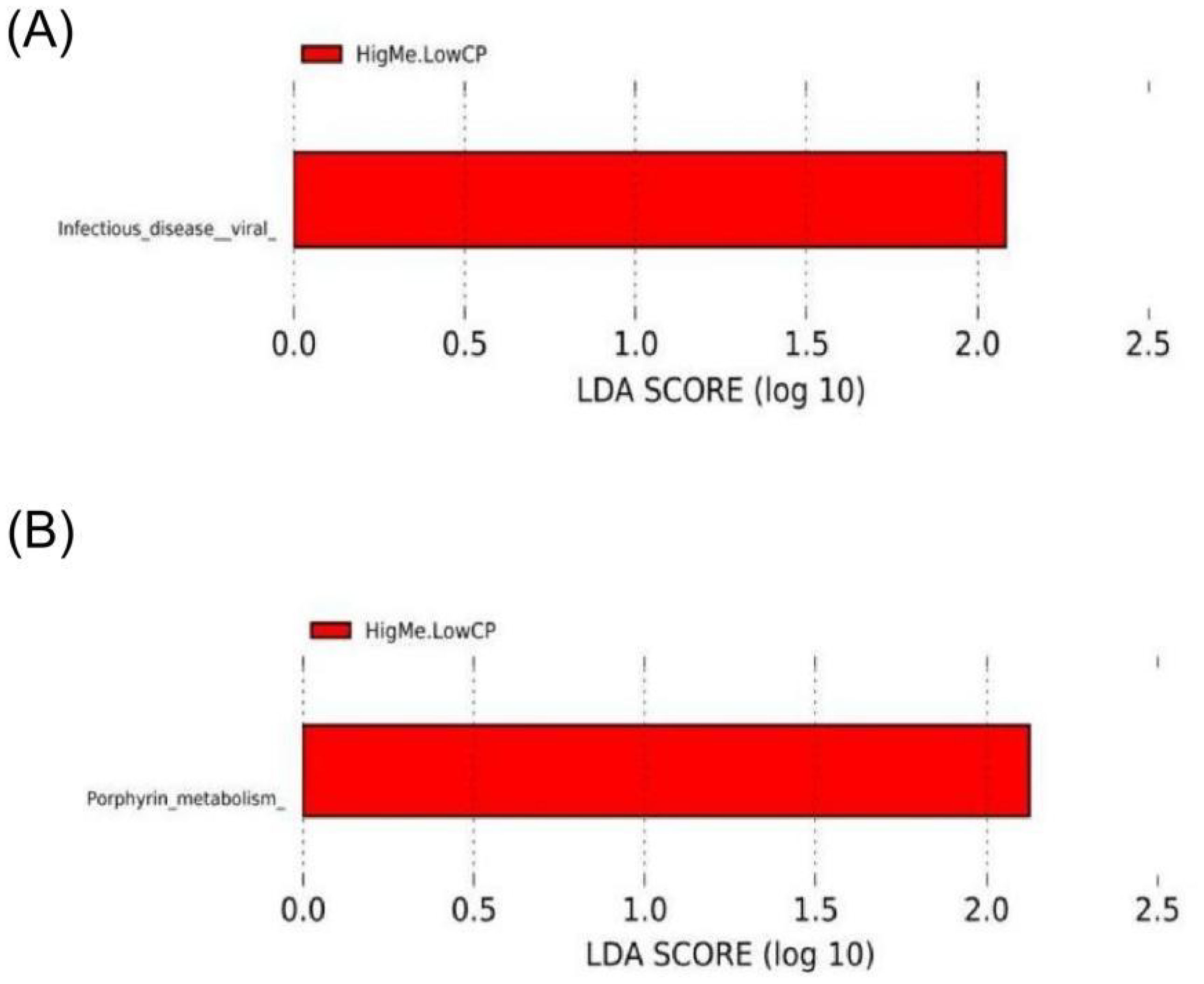
Figure 6. LEfSe analysis based on OTUs characterizes function between three groups. Histogram of the linear discriminant analysis (LDA) scores computed for features differentially abundant (significant threshold > 2 fold and p < 0.05) among hens was shown. (A) Three groups compared at KEGG L2 level; (B) Three groups compared at KEGG L3 level.
3.8 Analysis between liver oxidative stress and cecum microbiota
Correlation analysis was performed to obtain a visualized understanding of the relationship of the gut–liver axis in aged laying hens fed different energy low-protein diets. The correlation between liver oxidation indexes (MDA, T-AOC) and cecal flora was analyzed. We compared the HH group and HL groups, and MDA was significantly and positively correlated with M. woesei (p < 0.05; Figure 7A). T-AOC was highly significantly and positively correlated with Phocaeicola sp. Sa1YUN3 (p < 0.01; Figure 7A). There were non-significant difference between HH group and ML group (p > 0.05; Figure 7B).
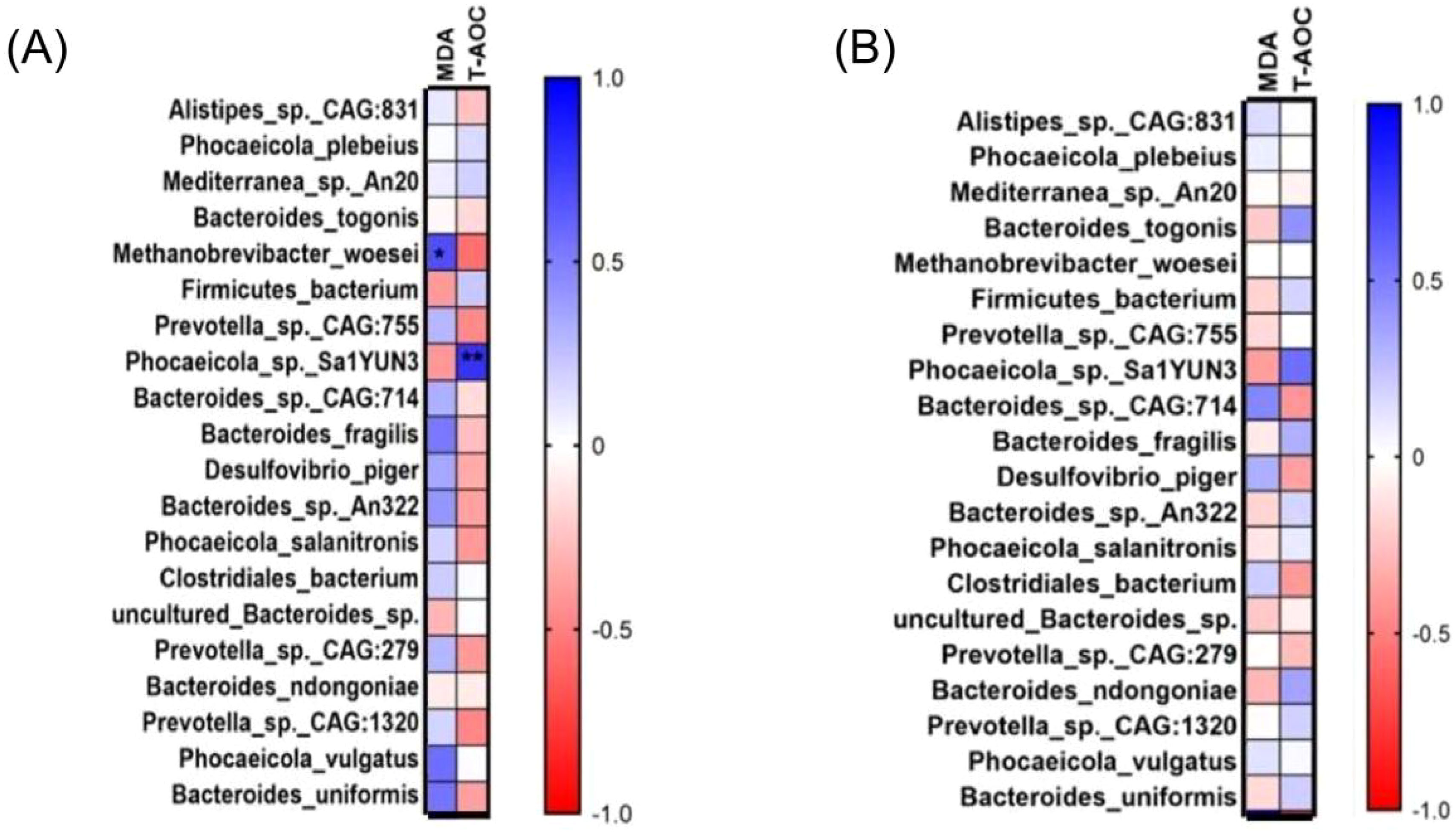
Figure 7. The correlations analysis between liver oxidation indexes and cecal flora parameters. (A) The correlations analysis between HH group and LL group. (B) The correlations analysis between HH group and ML group. *Representative significant difference between two groups (p < 0.05); **Representative extremely significant difference between two groups (p < 0.01).
4 Discussion
HFD is one of the important causes of obesity and liver oxidative stress (Milagro et al., 2006; Chu et al., 2024). MDA is a soluble degradation product of lipids, and indirectly reflects the degree of cell damage. Oxidative stress occurs in tissues, and the cell membrane of tissue cells will produce MDA through an oxidation reaction. MDA is the final product of lipid peroxidation, and the MDA level increased significantly with the increase in energy (Chen et al., 2018). According to Lasker et al. (2019), oxidative stress induced by a high-energy diet significantly increased MDA content in rats. Hosseinian and Hasanzadeh (2021) increased dietary energy to 3,450 kcal/kg, increased serum MDA concentration and decreased T-AOC level in pigeons. Our results support this view. We also detected the contents of T-AOC, T-SOD, GSH, and GSH-Px, which play an important role in maintaining the internal redox balance; they are important indicators for evaluating the body ‘s antioxidant capacity (Li et al., 2022). T-AOC is an integrative index used to reflected the functional status of the body ‘s antioxidant system (Miao et al., 2017). In the liver, according to our results, the HL diet significantly increased the liver MDA level and decreased the T-AOC level. This result prove that the high-energy diet has an adversely affected on the liver oxidation ability of aged laying hens.
Microbes that colonize the intestine are called the gut microbiota, regarded as an organ that contributes to the regulation of host metabolism. The gut microbiota might also affect production efficiency by altering the shape of intestinal tissue in poultry. According to our results, at the genus level, Bacteroides and Parabacteroides play key roles in the host immune system. Bacteroides can protect the intestinal tract from pathogens and reduce the production of lipopolysaccharides in intestinal microorganisms (Yoshida et al., 2018). Parabacteroides can help the body regulate metabolism, relieve inflammation, and regulate the immune system (Fu et al., 2019). Some studies have found that Parabacteroides can protect against inflammation and obesity (Cui et al., 2022; Wang et al., 2022). Alistipes have protective effects against liver fibrosis but are pathogenic in colorectal cancer and cardiovascular diseases (Rau et al., 2018; Moschen et al., 2016; Zuo et al., 2019). Polansky et al. Polansky et al. (2015) reported that in the cecal microbiota of chickens, Alistipes is a propionate producer. In research conducted by Oliphant and Allen-Vercoe (2019) also found that Alistipes can benefit the host gut and produce acetate in the cecum. Alistipes has a protective role, and a decrease in its abundance correlates with an increase in hepatic encephalopathy recurrence (Sung et al., 2019). Alistipes grows particularly well in the gut microbiota of patients who are obese, suggesting that Alistipes is associated with obesity. Therefore, the LL group had the lowest risk of liver cirrhosis. Desulfovibrio can act as opportunistic pathogens or induce asymptomatic infections in the host gastrointestinal (GI) tract (Urata et al., 2008). Desulfovibrio infections are often associated with inflammation (Ma et al., 2018). Gibson et al. (2010) postulated a role for Desulfovibrio in ulcerative colitis (UC). Our results showed Desulfovibrio abundance in the HL group; thus, a high energy diet was not conducive to host gut health. At the species level, Bacteroides fragilis was the lowest in the LL group. Bacteroides fragilis is a Gram-negative anaerobic bacterium that colonizes the host’s lower gastrointestinal tract. Although Bacteroides fragilis occupies a eminently low proportion of the gut microbiome, it benefits the immune system. Decreases in Bacteroides fragilis can lead to ulcerative colitis and Crohn’s disease (Zamani et al., 2017), which are associated with an imbalance in the intestinal flora. Furthermore, Desulfovibrio piger increased with an increase in energy. Loubinoux et al. (2002) found that the number of Desulfovibrio piger significantly increased in patients with inflammatory bowel disease. Doumatey et al. (2020) have reported that Desulfovibrio piger improves hyperglycemia and reduces insulin resistance.
As the core of the liver-gut axis, intestinal flora is involved in the regulation of liver diseases. We found that liver oxidation indexes were closely related to some microorganisms in the cecum. In our research, M.woesei was significantly correlated with the MDA level; Phocaeicola sp. Sa1YUN3 was significantly correlated with the T-AOC level. In this study, M.woesei increased with an increase in energy. Notably, M. woesei participates in the regulation of gut metabolism, such as methane metabolism. Additionally, in this study, M.woesei had higher intestinal content in chickens with higher abdominal fat content (Xiang et al., 2021). Our research also found that as the MDA level increased, M.woesei content increased. According to our results, Phocaeicola sp. Sa1YUN3 was highly abundant in the HL group. Sun et al. found Phocaeicola sp. could improved colitis in mice. Choi et al. (2023) found that the HF diet increased the relative abundance of Phocaeicola, supported by our findings.
As an important metabolic organ of the body, the liver can maintain oxidative balance by scavenging excessive reactive oxygen species in the body. Intestinal flora imbalance can cause oxidative stress (Vajro et al., 2013). Intestinal microorganisms are closely related to the liver through the gut–liver axis. This result has been further confirmed in our experiments. As an important component of the diet, energy affects not only affect intestinal composition, but also affect liver function. We found that high-energy–low-protein diets can cause oxidative stress in the liver.
5 Conclusions
In this study, a high-energy-low-protein diet caused oxidative stress in laying hens. According to our results, the HL group was enriched in the infectious disease viral pathway at the KEGG L2 level, indicating that feeding a high-energy-low-protein diet affected the intestinal health of chickens adversely. Our experiment further demonstrated that high-energy diets cause oxidative stress in laying hens through the gut-liver axis. This research might indicate that high energy adversely affects the production of laying hens.
Data availability statement
The datasets presented in this study can be found in online repositories. The names of the repository/repositories and accession number(s) can be found in the article/supplementary material.
Ethics statement
The animal study was approved by Institutional Animal Care and Use Committee of Yunnan Agricultural University (Approval Number: 202202001). The study was conducted in accordance with the local legislation and institutional requirements.
Author contributions
QM: Formal analysis, Writing – original draft. XD: Data curation, Writing – original draft. PW: Investigation, Methodology, Writing – original draft. HP: Writing – review & editing. YH: Formal analysis, Writing – review & editing. HH: Validation, Visualization, Writing – review & editing. AL: Software, Writing – review & editing. QZ: Conceptualization, Supervision, Writing – review & editing. SW: Conceptualization, Funding acquisition, Project administration, Resources, Writing – review & editing.
Funding
The author(s) declare financial support was received for the research, authorship, and/or publication of this article. This work was supported by China Agriculture Research System (No. CARS□40-S25), National Key R&D Program of China (No. 2022YFD1601905), theIndustrial Innovation Talent Project of the “Xing Dian Talent Support Program” of Yunnan Province in 2022 (XDYC-CYCX-2022–0029) and the Young Talent Project of the “Xing Dian Talent Support Program” of Yunnan Province in 2023.
Conflict of interest
The authors declare that the research was conducted in the absence of any commercial or financial relationships that could be construed as a potential conflict of interest.
Publisher’s note
All claims expressed in this article are solely those of the authors and do not necessarily represent those of their affiliated organizations, or those of the publisher, the editors and the reviewers. Any product that may be evaluated in this article, or claim that may be made by its manufacturer, is not guaranteed or endorsed by the publisher.
References
Abdollahi M. R., Zaefarian F., Ravindran V., Selle P. H. (2018). The interactive influence of dietary nutrient density and feed form on the performance of broiler chickens. Anim. Feed Sci. Tech. 239, 33–43. doi: 10.1016/j
Albillos A., De Gottardi A., Rescigno M. (2020). The gut-liver axis in liver disease: Pathophysiological basis for therapy. J. Hepatol. 72, 558–577. doi: 10.1016/j.jhep.2019.10.003
Ampong I., Watkins A., Gutierrez-Merino J., Ikwuobe J., Griffiths H. R. (2020). Dietary protein insufficiency: an important consideration in fatty liver disease? Brit J. Nutr. 123, 601–609. doi: 10.1017/S0007114519003064
Beam A., Clinger E., Hao L. (2021). Effect of diet and dietary components on the composition of the gut microbiota. Nutrients 13, 2795. doi: 10.3390/nu13082795
Cao Y., Li D. (2013). Impact of increased demand for animal protein products in Asian countries: Implications on global food security. Anim. Front. 3, 48–55, 3. doi: 10.2527/af.2013-0024
Chen H., Fu Y., Jiang X., Li D., Qin W., Zhang Q., et al. (2018). Arabinoxylan activates lipid catabolism and alleviates liver damage in rats induced by high-fat diet. J. Sci. Food Agric. 98, 253–260. doi: 10.1002/jsfa.8463
Choi K. J., Yoon M. Y., Kim J., Yoon S. S. (2023). Gut commensal Kineothrix alysoides mitigates liver dysfunction by restoring lipid metabolism and gut microbial balance. Sci. Rep-UK. 13, 14668. doi: 10.1038/s41598-023-41160-y
Chu Y., Zheng Y., Li Y., Gui S., Zhao J., Zhao Y., et al. (2024). Dietary supplementation of magnolol alleviates fatty liver hemorrhage syndrome in postpeak Xinhua laying hens via regulation of liver lipid metabolism. Poult Sci. 103, 103378. doi: 10.1016/j.psj.2023.103378
Crisol-Martínez E., Stanley D., Geier M. S., Hughes R. J., Moore R. J. (2017). Sorghum and wheat differentially affect caecal microbiota and associated performance characteristics of meat chickens. PeerJ. 5, e3071. doi: 10.7717/peerj.3071
Cui Y., Zhang L., Wang X., Yi Y., Shan Y., Liu B., et al. (2022). Roles of intestinal Parabacteroides in human health and diseases. FEMS Microbiol. Lett. 369, fnac072. doi: 10.1093/femsle/fnac072
Doumatey A. P., Adeyemo A., Zhou J., Lei L., Adebamowo S. N., Adebamowo C., et al. (2020) Gut microbiome profiles are associated with type 2 diabetes in urban Africans. Front. Cell Infect. Mi. 10. doi: 10.3389/fcimb.2020.00063
Fu X., Liu Z., Zhu C., Mou H., Kong Q. (2019). Nondigestible carbohydrates, butyrate, and butyrate-producing bacteria. Crit. Rev. Food Sci. 59:sup1, S130–S152. doi: 10.1080/10408398.2018.1542587
Gibson G. R., Cummings J. H., Macfarlane G. T. (2010). Competition for hydrogen between sulphate-reducing bacteria and methanogenic bacteria from the human large intestine. J. Appl. Bacteriology. 65, 241–247. doi: 10.1111/j.1365-2672.1988.tb01891.x
Hosseinian S. A., Hasanzadeh F. (2021). Impact of high dietary energy on obesity and oxidative stress in domestic pigeons. Vet. Med. Sci. 7, 1391–1399. doi: 10.1002/vms3.478
Konturek P. C., Harsch I. A., Konturek K., Schink M., Konturek T., Neurath M. F., et al. (2018). Gut–liver axis: how do gut bacteria influence the liver? Med. Sci. 6, 79. doi: 10.3390/medsci6030079
Lasker S., Rahman M. M., Parvez F., Zamila M., Miah P., Nahar K., et al. (2019). High-fat diet-induced metabolic syndrome and oxidative stress in obese rats are ameliorated by yogurt supplementation. Sci. Rep. 9, 20026. doi: 10.1038/s41598-019-56538-0
Li Q., Yang S., Chen F., Guan W., Zhang S. (2022). Nutritional strategies to alleviate oxidative stress in sows. Anim. Nutr. 9, 60–73. doi: 10.1016/j.aninu.2021.10.006
Liu X., Lin X., Zhang S., Guo C., Li J., Mi Y., et al. (2018). Lycopene ameliorates oxidative stress in the aging chicken ovary via activation of Nrf2/HO-1 pathway. Aging. 10, 2016–2036. doi: 10.18632/aging.101526
Loubinoux J., Bronowicki J. P., Pereira I. A., Mougenel J. L., Le Faou A. E. (2002). Sulfate-reducing bacteria in human feces and their association with inflammatory bowel diseases. FEMS Microbiol. Ecol. 40, 107–112. doi: 10.1111/j.1574-6941.2002.tb00942.x
Ma D., Wang A. C., Parikh I., Green S. J., Hoffman J. D., Chlipala G., et al. (2018). Ketogenic diet enhances neurovascular function with altered gut microbiome in young healthy mice. Sci. Rep. 8, 1–10. doi: 10.1038/s41598-018-25190-5
Miao L. P., Zhou M. Y., Zhang X. Y., Yuan C., Dong X. Y., Zou X. T. (2017). Effect of excess dietary fluoride on laying performance and antioxidant capacity of laying hens. Poult Sci. 96, 2200–2205. doi: 10.3382/ps/pex002
Milagro F. I., Campión J., Martínez J. A. (2006). Weight gain induced by high-fat feeding involves increased liver oxidative stress. Obes. (Silver Spring). 14, 1118–1123. doi: 10.1038/oby.2006.128
Moschen A. R., Gerner R. R., Wang J., Klepsch V., Adolph T. E., Reider S. J., et al. (2016). Lipocalin 2 protects from inflammation and tumorigenesis associated with gut microbiota alterations. Cell Host. Microbe 19, 455–469. doi: 10.1016/j.chom.2016.03.007
Oliphant K., Allen-Vercoe E. (2019). Macronutrient metabolism by the human gut microbiome: major fermentation by-products and their impact on host health. Microbiome 7, 1–15. doi: 10.1186/s40168-019-0704-8
Polansky O., Sekelova Z., Faldynova M., Sebkova A., Sisak F., Rychlik I. (2015). Important metabolic pathways and biological processes expressed by chicken cecal microbiota. Appl. Environ. Microb. 82, 1569–1576. doi: 10.1128/AEM.03473-15
Rau M., Rehman A., Dittrich M., Groen A. K., Hermanns H. M., Seyfried F., et al. (2018). Fecal SCFAs and SCFA-producing bacteria in gut microbiome of human NAFLD as a putative link to systemic T-cell activation and advanced disease. United Eur. Gastroent. 6, 1496–1507. doi: 10.1177/2050640618804444
Ringseis R., Gessner D. K., Eder K. (2020). The gut–liver axis in the control of energy metabolism and food intake in animals. Annu. Rev. Anim. Biosci. 8, 295–319. doi: 10.1146/annurev-animal-021419-083852
Scappaticcio R., Cámara L., Herrera J., Mateos G. G., de Juan A. F., Fondevila G. (2022). Influence of the energy concentration and the standardized ileal digestible lysine content of the diet on performance and egg quality of brown-egg laying hens from 18 to 41 weeks of age. Poultry Sci. 101, 102197. 7. doi: 10.1016/j
Sommer F., Bäckhed F. (2016). Know your neighbor: Microbiota and host epithelial cells interact locally to control intestinal function and physiology. BioEssays 38, 455–464. doi: 10.1002/bies.201500151
Sonnenburg J. L., Bäckhed F. (2016). Diet–microbiota interactions as moderators of human metabolism. Nature 535, 56–64. doi: 10.1038/nature18846
Sung C. M., Lin Y. F., Chen K. F., Ke H. M., Huang H. Y., Gong Y. N., et al. (2019). Predicting clinical outcomes of cirrhosis patients with hepatic encephalopathy from the fecal microbiome. Cell Mol. Gastroenter. 8, 301–318. doi: 10.1016/j.jcmgh.2019.04.008
Tan B. L., Norhaizan M. E., Liew W. P. (2018). Nutrients and oxidative stress: friend or foe? Oxid. Med. Cell Longev. 1, 9719584.2018. doi: 10.1155/2018/9719584
Thirumalaisamy G., Muralidharan J., Senthilkumar S., Hema Sayee R., Priyadharsini M. (2016). Cost-effective feeding of poultry. Int. J. Environ. Sci. Te. 5, 3997–4005.
Urata T., Kikuchi M., Hino T., Yoda Y., Tamai K., Kodaira Y., et al. (2008). Bacteremia caused by Desulfovibrio fairfieldensis. J. Infect. Chemother. 14, 368–370. doi: 10.1007/s10156-008-0629-9
Vajro P., Paolella G., Fasano A. (2013). Microbiota and gut-liver axis: their influences on obesity and obesity-related liver disease. J. Pediatr. Gastr Nutr. 56, 461–468. doi: 10.1097/MPG.0b013e318284abb5
Wang P., Ma Y., Wang D., Zhao W., Hu X., Chen F., et al. (2022). Protective Effects of Dietary Resveratrol against Chronic Low-Grade Inflammation Mediated through the Gut Microbiota in High-Fat Diet Mice. Nutrients 10, 14(10):1994. doi: 10.3390/nu14101994
Xiang H., Gan J., Zeng D., Li J., Yu H., Zhao H., et al. (2021). Specific microbial taxa and functional capacity contribute to chicken abdominal fat deposition. Front. Microbiol. 12. doi: 10.3389/fmicb.2021.643025
Yan W., Sun C., Yuan J., Yang N. (2017). Gut metagenomic analysis reveals prominent roles of Lactobacillus and cecal microbiota in chicken feed efficiency. Sci. Rep-UK. 7, 45308. doi: 10.1038/srep45308
Yoshida N., Emoto T., Yamashita T., Watanabe H., Hayashi T., Tabata T., et al. (2018). Bacteroides vulgatus and Bacteroides dorei reduce gut microbial lipopolysaccharide production and inhibit atherosclerosis. Circulation 138, 2486–2498. doi: 10.1161/CIRCULATIONAHA.118.033714
Zamani S., Hesam Shariati S., Zali M. R., Asadzadeh Aghdaei H., Sarabi Asiabar A., Bokaie S., et al. (2017). Detection of enterotoxigenic Bacteroides fragilis in patients with ulcerative colitis. Gut Pathog. 9, 1–7. doi: 10.1186/s13099-017-0202-0
Zuo K., Li J., Li K., Hu C., Gao Y., Chen M., et al. (2019). Disordered gut microbiota and alterations in metabolic patterns are associated with atrial fibrillation. Gigascience 8, giz058. doi: 10.1093/gigascience/giz058
Keywords: energy, low protein diet, laying hen, liver, oxidative stress, cecum microbiota, metagenomics
Citation: Mi Q, Deng X, Wang P, Pan H, Huang Y, Hu H, Li A, Zhang Q and Wang S (2024) Effect of energy levels on liver oxidative state and gut microbiota of laying hens fed a low-protein diet. Front. Anim. Sci. 5:1465305. doi: 10.3389/fanim.2024.1465305
Received: 16 July 2024; Accepted: 11 October 2024;
Published: 04 November 2024.
Edited by:
Demin Cai, Yangzhou University, ChinaReviewed by:
Ravikanth Reddy Poonooru, University of Missouri, United StatesGuofeng Han, Jiangsu Academy of Agricultural Sciences (JAAS), China
Copyright © 2024 Mi, Deng, Wang, Pan, Huang, Hu, Li, Zhang and Wang. This is an open-access article distributed under the terms of the Creative Commons Attribution License (CC BY). The use, distribution or reproduction in other forums is permitted, provided the original author(s) and the copyright owner(s) are credited and that the original publication in this journal is cited, in accordance with accepted academic practice. No use, distribution or reproduction is permitted which does not comply with these terms.
*Correspondence: Qiang Zhang, MTU5NjMwNDk3MzFAMTYzLmNvbQ==; Shaoqing Wang, d3NxMjMxQDEyNi5jb20=
†These authors share first authorship