- Department of Biological Sciences, Andong National University, Andong, Republic of Korea
Prion diseases are fatal infectious neurodegenerative diseases caused by the proteinase K-sensitive form of prion protein (PrPSc). The exact origin of prion seeding and the transition factor of PrPSc has not been elucidated. The main hosts of prion diseases are herbivores, so the feces and corpses of Amphibians can seed PrPSc through ecosystems. The frog is an excellent candidate for transmission studies for this reason, but genetic analyses of the prion protein gene (PRNP) in the context of prion-related characteristics of frog species are lacking. We amplified frog PRNP gene sequences in Dybowski’s frog and the American bullfrog by polymerase chain reaction (PCR) and amplicon sequencing. In addition, we carried out multiple sequencing alignments and annotated major PrP components including signal peptide, tandem repeat domain, and PrPC-PrPSc interaction region of frog PrPs by bioinformatics tools. We predicted secondary and tertiary structures and amyloid propensities of frog PrPs using AlphaFold2 and AMYCO, respectively. We obtained DNA sequences of the PRNP gene in Dybowski’s frog and the American bullfrog, as well as a partially conserved palindromic sequence (PrPC-PrPSc interaction region) and absence of tandem repeat region of PrP in seven frog species. We analyzed protein structure of among these frog species and found that the high Himalaya frog has high aggregation propensity and the western clawed frog does not have the N-terminal signal peptide. To the best of our knowledge, this was the first comparative genetic study regarding prion-related features of frog species.
1 Introduction
Prion diseases are amyloid-induced neurodegenerative diseases caused by beta sheet-rich and proteinaceous infectious particles (PrPSc) converted from normal prion protein (PrPC), leading to dysfunction of the central nervous system accompanied by accumulation of PrPSc, vacuolation, and gliosis in brain lesions (Prusiner and Dearmond, 1994; Johnson, 2005; Kim et al., 2021). Since the first prion disease, scrapie, was initially reported in 1732 (Nichols, 2014), various prion diseases have been presumed to be transmitted across species barriers and in a wide range of hosts including goats, cattle, deer, elk, raccoon, mink and humans over more than two centuries (Marsh and Hadlow, 1992; Kirkwood and Cunningham, 1994; Anderson et al., 1996; Bons et al., 1996; Lasmezas et al., 1996; Bruce et al., 1997; Baron et al., 1999; Bons et al., 1999; Lasmézas et al., 2001; Marsh et al., 2005). The central event of prion diseases is that seed prions convert templates of PrPSc, the prion protein (PrP), which is encoded by prion protein gene (PRNP) (Kupfer et al., 2009). PrP is mainly expressed on cell surfaces and have several major conserved components across species including the signal peptide, tandem repeat domain “AGAAAAGA” palindromic sequence (PrPC-PrPSc interaction region) and glycosylphosphatidylinositol (GPI) anchor (Gabriel et al., 1992; Norstrom and Mastrianni, 2005; Orge et al., 2021). To date, several pathogenic mutations of the PRNP gene associated with prion disease progression have been identified. In sheep, three-codon haplotypes A136V/T, R154H/L, and Q171R/H/K have been reported to affect susceptibility to scrapie (Baylis and Goldmann, 2004). The 12-bp insertion/deletion (in/del) polymorphism in the introns and the 23-bp in/del polymorphism in the promoter region are pivotal risk factors for bovine spongiform encephalopathy (BSE) (Mead et al., 2019). In deer, single nucleotide polymorphism (SNP), such as Q95H, G96S, and A116G in white-tailed deer, and S225F in mule deer, affect vulnerability to chronic wasting disease (CWD) (Moazami-Goudarzi et al., 2021). However, the proportion of genetic human prion diseases is low (<15%), no other animal prion disease-related casual mutations have been identified thus far (Appleby et al., 2022). Prion disease does not occur in brain organoids carrying a pathogenic mutation E200K. In addition, PrP synthesized by bioengineering does not show PrPSc seeding activity (Foliaki et al., 2020). Therefore, the exact origin of prion seeds or transition factors of spontaneously generated PrPSc has not been elucidated thus far.
The main hosts of prion disease are herbivores, so feces and corpses of animals that potentially spontaneously generate PrPSc, living around plants and water, can be the seed or transition factors of PrPSc through ecosystems (Pritzkow et al., 2015, Pritzkow et al., 2018). Among animals with these habitat characteristics, there are over 8,694 species of amphibians (AmphibiaWeb database; accessed on Nov 18 2023), of which 7,658 are frogs and toads with similar habitat characteristics to herbivores, the main hosts of prion diseases. A previous bioinformatic study reported that frog PrP has a modified binding sequence of PrPSc (AIGAAAGA) and does not have tandem repeat sequences that contribute copper-ion homeostasis, unlike other prion-related species (Calzolai et al., 2005; Kim et al., 2008). However, that study investigated only one species, the African clawed frog (Xenopus laevis) and did not evaluate potential for prion seeding activity, such as the amyloid propensity of PrP.
In Korea, Rana dybowskii (Dybowski’s frog) is one of most common indigenous frog species (Yang et al., 2000). Dybowski’s frog is raised in commercial farms and consumed as meat and as a health food. Another major food species is Lithobates catesbeianus (American bullfrog), which was imported from North America in 1958. The main infection route for prions is the gastrointestinal tract, and although frogs have been reported to have PrP, no research has been conducted on prions from these two frog species that are widely consumed in Korea (Davies et al., 2006; Harrison et al., 2010).
In the current study, we present the first report of the frog PRNP gene, in Dybowski’s frog and the American bullfrog, derived by polymerase chain reaction (PCR) and amplicon sequencing. In addition, we performed multiple sequencing alignments and analyzed major PrP components including signal peptide, tandem repeat domain, the PrPC-PrPSc interaction region, and the GPI-anchor of frog PrPs using bioinformatics tools. Furthermore, we analyzed secondary and tertiary structures and amyloid propensities of frog PrPs using AlphaFold2 and AMYCO, respectively.
2 Materials and methods
2.1 Animal samples
Four frogs of two species (two Rana dybowskii, two Lithobates catesbeianus) were obtained from animal farms in the Republic of Korea. The experimental procedures were reviewed and accredited by the Institutional Animal Care and Use Committee of Andong National University (IACUC No. 2023-2-0420-02-01).
2.2 Genomic DNA extraction
Genomic DNA was extracted from muscle tissue using QIAGEN DNA Purification Kits (QIAGEN, Hilden, Germany) following the manufacturer’s guidelines.
2.3 Polymerase chain reaction (PCR)
To identify the PRNP DNA sequences of these species, we designed forward and reverse primers using Primer3Plus (https://www.bioinformatics.nl/cgi-bin/primer3plus/primer3plus.cgi) based on the PRNP DNA sequence of Rana temporaria (common frog) registered in GenBank at the National Center for Biotechnology Information (NCBI) (Gene ID: 120932994) (PRNP-F: 5’- ttgcaaaatgagaccctgtg-3’; PRNP-R: 5’-gcagtggagcaggatatctgtat-3’). The designed primers were used to amplify the coding region of the frog PRNP gene by PCR using BioFACT™ Taq DNA Polymerase (BioFACT, Daejeon, Korea) following the manufacturer’s guidelines.
PCR was conducted using peripheral tissue-extracted genomic DNA from Rana dybowskii and Lithobates catesbeianus. PCR was performed in an S-1000 Thermal Cycler (Bio-Rad, Hercules, CA, USA), and the PCR conditions were as follows: 95°C for 3 minutes, followed by 36 cycles of 95°C for 30 seconds, 65°C for 30 seconds, and 72°C for 1 minute, and then 1 cycle of 72°C for 10 minutes for the final extension. The length of the PCR products was verified using a BioFACT™ DNA Ladder (Biofact, Daejeon, Korea). PCR products were eluted using the AccuPrep® PCR/Gel Purification Kit (Bioneer, Daejeon, Korea) and analyzed with an ABI PRISM 3730XL Analyzer (ABI, Foster City, CA, USA). The sequencing result was analyzed using Finch TV software (Geospiza Inc., Seattle, WA, USA). The 899 bp amplicon was verified by agarose gel electrophoresis (Supplementary Figure 1).
2.4 Multiple sequence alignments and phylogenetic analysis of frog PrPs
The frog PrP sequences were collected from GenBank or obtained in the present study. Detailed information on amino acid sequences is as follows: Xenopus tropicalis (western clawed frog, XP_012821570.1), Xenopus laevis (African clawed frog, XP_018106305.1), Hyla sarda (Sardinian tree frog, XP_056427363.1), Nanorana parkeri (high Himalaya frog, XP_018424224.1), Rana temporaria (common frog, XP_040201852.1), Lithobates catesbeianus (American bullfrog, In this study), Rana dybowskii (Dybowski’s frog, In this study). The PrP sequences were compared using ClustalW2 (https://www.ebi.ac.uk/Tools/msa/clustalo/).
The amino acid sequences of frog PrPs were used for phylogenetic analysis by MEGA X program using the neighbor-joining method with 3,000 bootstrap replicates. The tree was drawn based on evolutionary distances estimated using the Poisson correction method.
2.5 Secondary and tertiary structure analysis of frog PrPs
Secondary and tertiary structures of frog PrPs were predicted by Alphfold2 based on machine learning (https://colab.research.google.com/github/sokrypton/ColabFold/blob/main/AlphaFold2.ipynb). The confidence of predicted structures was assessed by the predicted local distance difference test (pLDDT) value. Electrostatic potential and hydrogen bonds were predicted and visualized by the DeepView program (https://spdbv.unil.ch). Hydrogen bonds were analyzed according to amino acid properties and interatomic distance and predicted whether hydrogen was in the range from 1.2 to 2.76 Å of a potential donor atom. Electrostatic potential was predicted by the Poisson-Boltzmann equation based on the surface charges of surface amino acids.
2.6 Amyloid propensity
AMYCO (http://bioinf.uab.es/amycov04) was used to predict the aggregation propensity of frog PrPs. AMYCO calculates the impact of amyloidogenic sequences based on pWALTZ and PAPA algorithms with the PSEP score. The detailed PSEP score indicated weak aggregation propensity (<0.45).
2.7 N-terminal signal peptide prediction
The N-terminal signal peptide was predicted using SignalP 5.0 (https://services.healthtech.dtu.dk/service.php?SignalP-5.0) using a neural network-based internal algorithm.
3 Results
3.1 Identification of the PRNP gene sequences in Dybowski’s frog and American bullfrog
There were no previous reports of the DNA sequences of the PRNP gene in Rana dybowskii and Lithobates catesbeianus. The morphological differences between the two frog species are presented inSupplementary Figure 2. To identify the PRNP DNA sequences of these species, we designed forward and reverse primers based on the PRNP DNA sequence of Rana temporaria (common frog) registered in GenBank. In this study, we carried out amplicon sequencing and identified the frog PRNP DNA sequence of Rana dybowskii and Lithobates catesbeianus for the first time.
To investigate differences of the open reading frame (ORF) in the PRNP gene among seven frog species, we compared the DNA sequences using ClustalW2 (Figures 1A, B). Among seven frog species, Dybowski’s frog showed the highest DNA sequence similarity with Rana temporaria (common frog, 98.9%), followed by American bullfrog (95.8%) and Nanorana parkeri (high Himalaya frog, 82.3%). Of note, some frog species, including Xenopus tropicalis (Western clawed frog 53.4%), Xenopus laevis (African clawed frog, 53.6%) and Hyla sarda (Sardinian tree frog, 65.6%) showed low DNA sequence similarity (50-60%) with Dybowski’s frog.
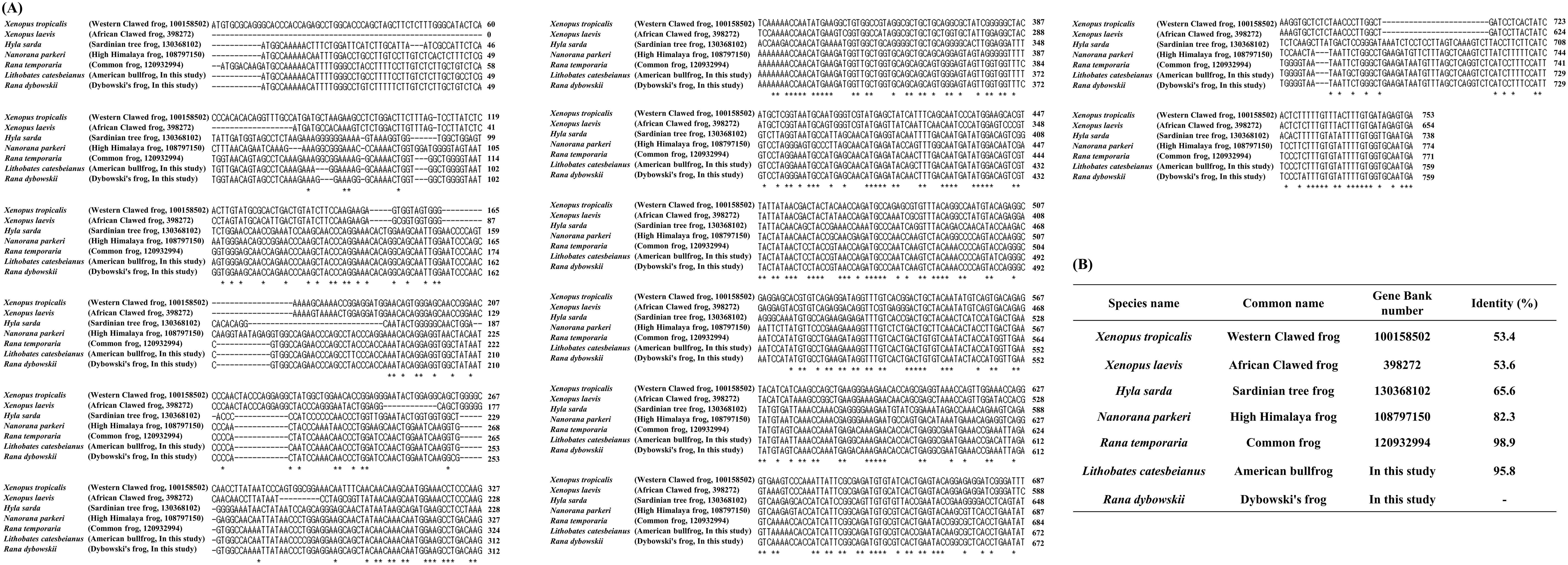
Figure 1. The comparison of the open reading frame (ORF) of the prion protein gene (PRNP) was compared in 7 frog species. (A) Multiple sequence alignment of ORF of the PRNP gene sequences in frog species using ClustalW2. (B) Detailed information on the sequence similarity of ORF of the PRNP gene sequences in seven frog species. The sequence alignment includes Xenopus tropicalis (western clawed frog, Gene ID: 100158502), Xenopus laevis (African clawed frog, Gene ID: 398272), Hyla sarda (Sardinian tree frog, Gene ID: 130368102), Nanorana parkeri (high Himalaya frog, Gene ID: 108797150), Rana temporaria (common frog, Gene ID: 120932994), Lithobates catesbeianus (American bullfrog, Gene ID: In this study) and Rana dybowskii (Dybowski’s frog, Gene ID: In this study). Asterisks indicate identical positions among frog species.
3.2 Comparison amino acid sequences of frog PrPs
We performed multiple sequence alignment to find prion-related features of the amino acid sequences of frog PrPs (Figure 2). Similar to our DNA comparison results, Dybowski’s frog showed the highest similarity of amino acid sequence with the common frog (99.6%), followed by American bullfrog (93.6%) and high Himalaya frog (77.4%). Of note, some frog species, including western clawed frog (48.8%), African clawed frog (50%) and Sardinian tree frog (62.7%) showed low similarity (40-60%) of amino acid sequences with Dybowski’s frog.
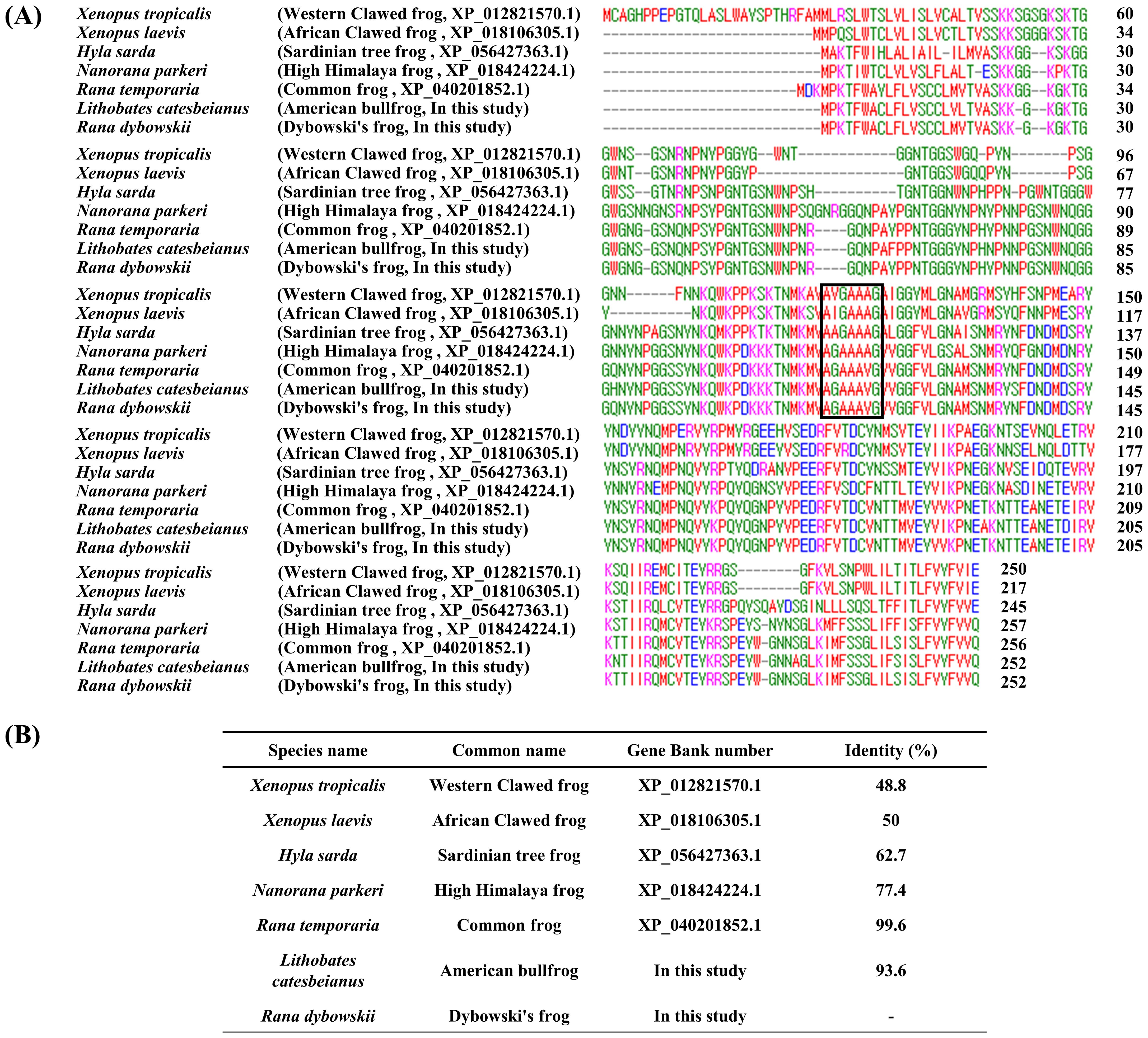
Figure 2. The comparison of the amino acid sequences of the prion protein (PrP) in 7 frog species. (A) Multiple sequence alignment of the amino acid sequences of the PrP in 7 frog species. (B) Detailed information on the sequence similarity of amino acid sequences of the PrP in 7 frog species. Protein sequences were obtained from GenBank at the National Center for Biotechnology Information (NCBI), including those of Xenopus tropicalis (Western clawed frog, XP_012821570.1), Xenopus laevis (African clawed frog, XP_018106305.1), Hyla sarda (Sardinian tree frog, XP_056427363.1), Nanorana parkeri (high Himalaya frog, XP_018424224.1), Rana temporaria (common frog, XP_040201852.1), Lithobates catesbeianus (American bullfrog, In this study) and Rana dybowskii (Dybowski’s frog, In this study). Protein sequence alignments were performed among frog species using ClustalW2. Colors indicate chemical properties: green, hydroxyl, sulphhydryl, amine and glycine; blue: acid; red, small and hydrophobic; magenta, basic. Black box indicates palindromic sequence (normal prion protein (PrPC)-abnormal prion protein (PrPSc) interaction region).
Except for Xenopus tropicalis (western clawed frog), all frog species showed high levels of similarity in the N-terminal region (1-30aa). Similar to a previous study of the African clawed frog (Calzolai et al., 2005; Kim et al., 2008), we did not find tandem repeat domains (octapeptide repeat region) in all seven frog species. However, the “AGAAAAGA” palindromic sequence (PrPC-PrPSc interaction region) was partially conserved across all seven frog species (western clawed frog: AVGAAAGA; African clawed frog: AIGAAAGA; Sardinian tree frog: AAGAAAGA; high Himalaya frog: AGAAAAGV; common frog: AGAAAVGV; American bullfrog: AGAAAVGV; Dybowski’s frog: AGAAAVGV).
To investigate the evolutionary relationships of frog species, the amino acid sequences of the PrPs were analyzed by MEGA X using the neighbor-joining method (Figure 3). Similar to the multiple sequence alignment results, Dybowski’s frog showed the closest evolutionary relationship with the common frog, followed by American bullfrog and high Himalaya frog. The two clawed frog species, western and African clawed frogs, showed the most distant evolutionary relationships from Dybowski’s frog.
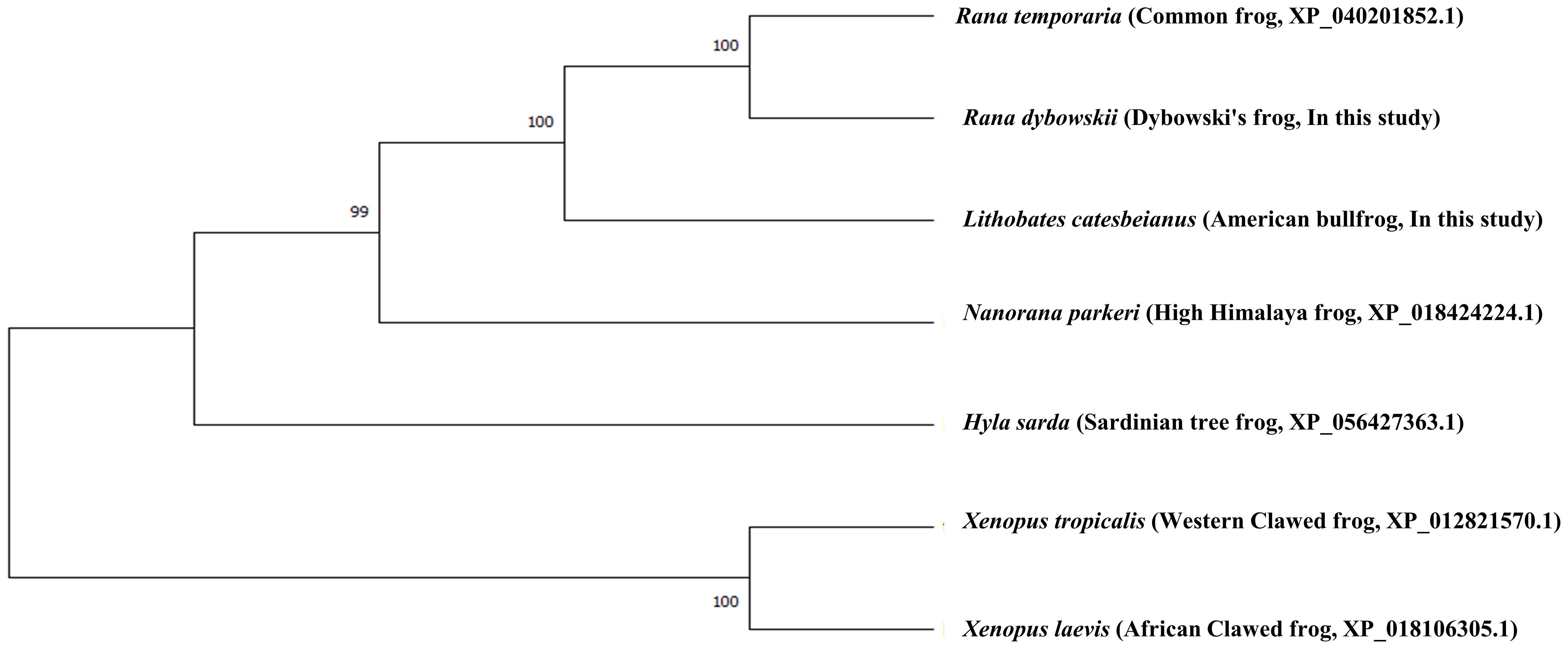
Figure 3. Phylogenetic analyses of the PrP in 7 frog species. The phylogenetic tree was obtained using the Molecular Evolutionary Genetics Analysis (MEGA) X software and includes amino acid sequences of Xenopus tropicalis (Western clawed frog, XP_012821570.1), Xenopus laevis (African clawed frog, XP_018106305.1), Hyla sarda (Sardinian tree frog, XP_056427363.1), Nanorana parkeri (high Himalaya frog, XP_018424224.1), Rana temporaria (common frog, XP_040201852.1), Lithobates catesbeianus (American bullfrog, In this study) and Rana dybowskii (Dybowski’s frog, In this study). The phylogenetic tree was analyzed by the neighbor-joining method and branch nodes indicate bootstrap confidence intervals (3,000 replicates). The branch lengths represent the number of amino acid substitutions per site.
3.3 Secondary and tertiary structures of frog PrPs
We analyzed secondary and tertiary structures of frog PrPs by AlphaFold2 (Figure 4). All frog PrPs showed two beta-sheet structures. However, the number of alpha-helixes was variable among frog species. In brief, the Sardinian tree and high Himalaya frogs both had the greatest number of alpha-helixes, 8. Dybowski’s frog showed the fewest, 5.
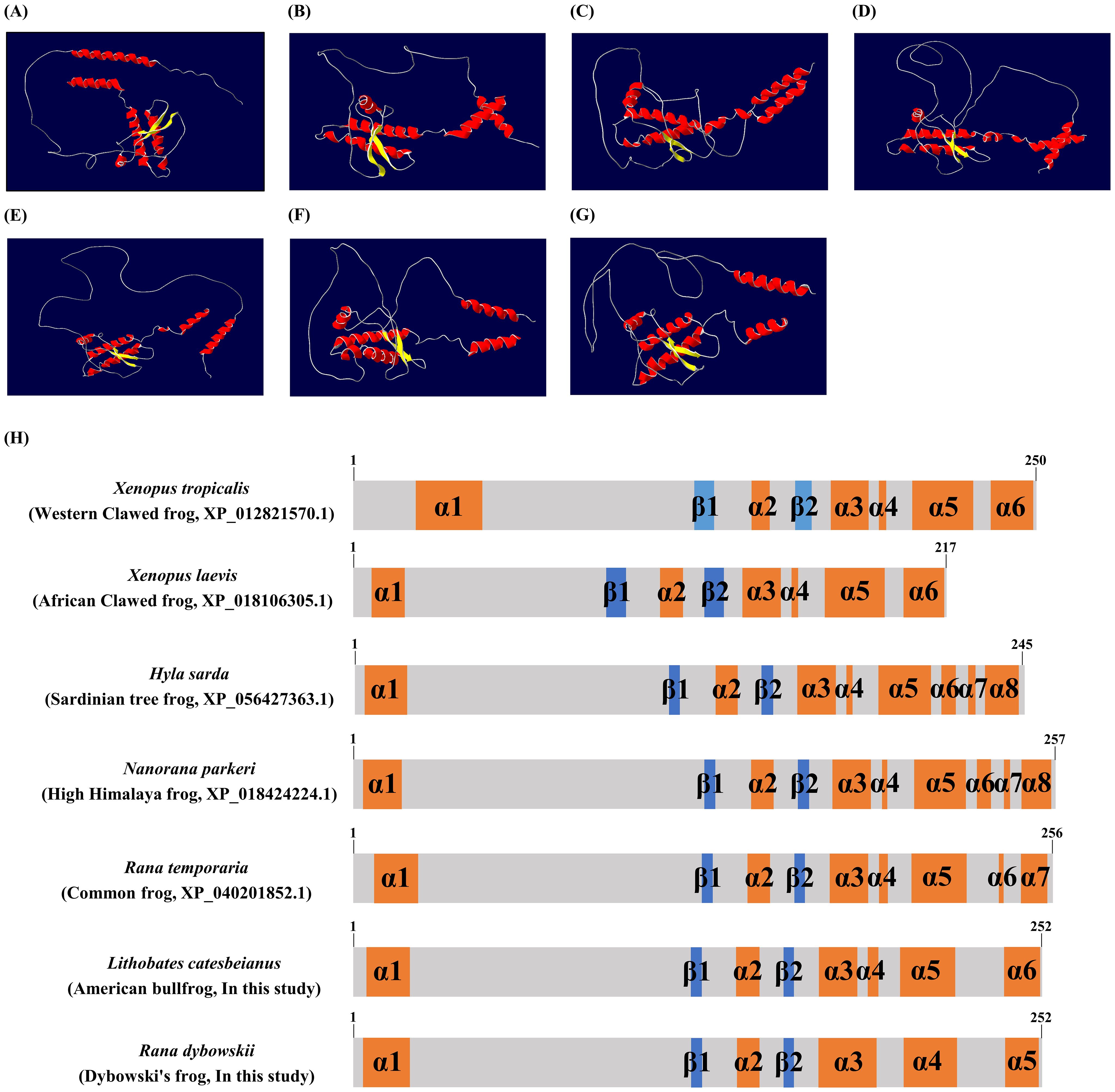
Figure 4. The tertiary and secondary structures of frog PrPs. The 3D modeling of the tertiary structure model and schematic representation of the secondary structure of frog PrPs was predicted by AlphaFold2. (A) The tertiary structure of the Xenopus tropicalis (Western clawed frog) PrP. (B) The tertiary structure of the Xenopus laevis (African clawed frog) PrP. (C) The tertiary structure of the Hyla sarda (Sardinian tree frog) PrP. (D) The tertiary structure of the Nanorana parkeri (high Himalaya frog) PrP. (E) The tertiary structure of the Rana temporaria (common frog) PrP. (F) The tertiary structure of the Lithobates catesbeianus (American bullfrog) PrP. (G) The tertiary structure of the Rana dybowskii (Dybowski’s frog) PrP. Colors indicate β-sheets (yellow), α-helices (red) and coils (white). (H) The secondary structure of 7 frog species including Xenopus tropicalis (Western clawed frog), Xenopus laevis (African clawed frog), Hyla sarda (Sardinian tree frog), Nanorana parkeri (high Himalaya frog), Rana temporaria (common frog), Lithobates catesbeianus (American bullfrog, In this study) and Rana dybowskii (Dybowski’s frog, In this study). The colors indicate α-helices (orange), β-sheets (blue) and coils (gray).
3.4 Identification of high amyloid propensity in high Himalaya frog PrP
To evaluate the amyloid propensity of frog PrPs, we performed in silico analysis using AMYCO (Figure 5). The high Himalaya frog PrP showed the greatest amyloid propensity (0.61), followed by the African clawed frog (0.45), American bullfrog (0.29), and common frog (0.29). The lowest amyloid propensity was observed in the Sardinian tree frog PrP (0).
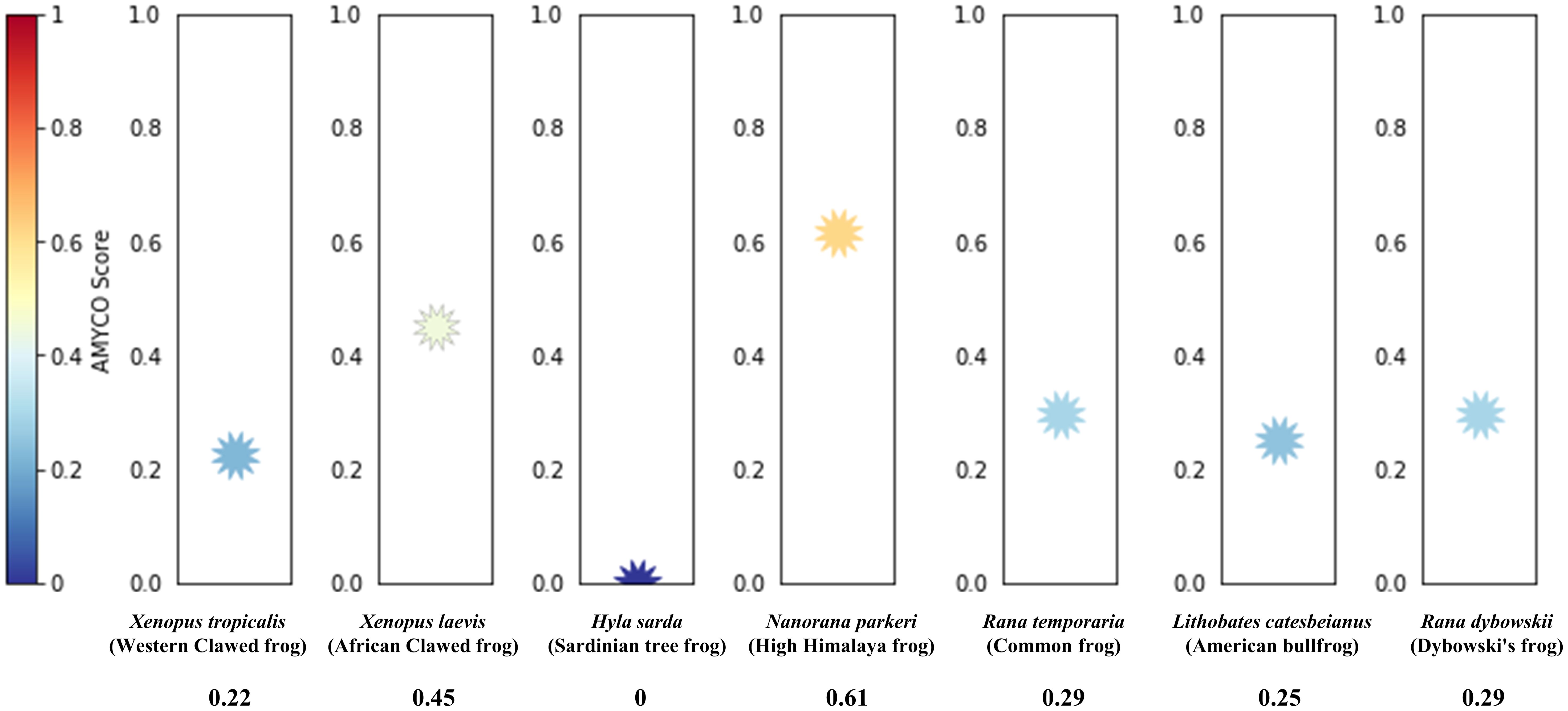
Figure 5. Prediction of the aggregation propensities in frog PrPs species including 7 frog species including Xenopus tropicalis (Western clawed frog), Xenopus leavis (African clawed frog), Hyla sarda (Sardinian tree frog), Nanorana parkeri (high Himalaya frog), Rana temporaria (common frog), Lithobates catesbeianus (American bullfrog, In this study) and Rana dybowskii (Dybowski’s frog, In this study). The aggregation propensities of the PrPs were analyzed by AMYCO.
3.5 Signal peptide prediction
Since N-terminal amino acid sequences are significant determinants of signal peptides, we predicted N-terminal signal peptide sequences using SignalP 5.0. Except for the western clawed frog, all frog PrPs have signal peptide sequences and cutting sites in the N-terminal region (Figure 6). We did not predict the signal peptide sequence or cutting site for the western clawed frog.
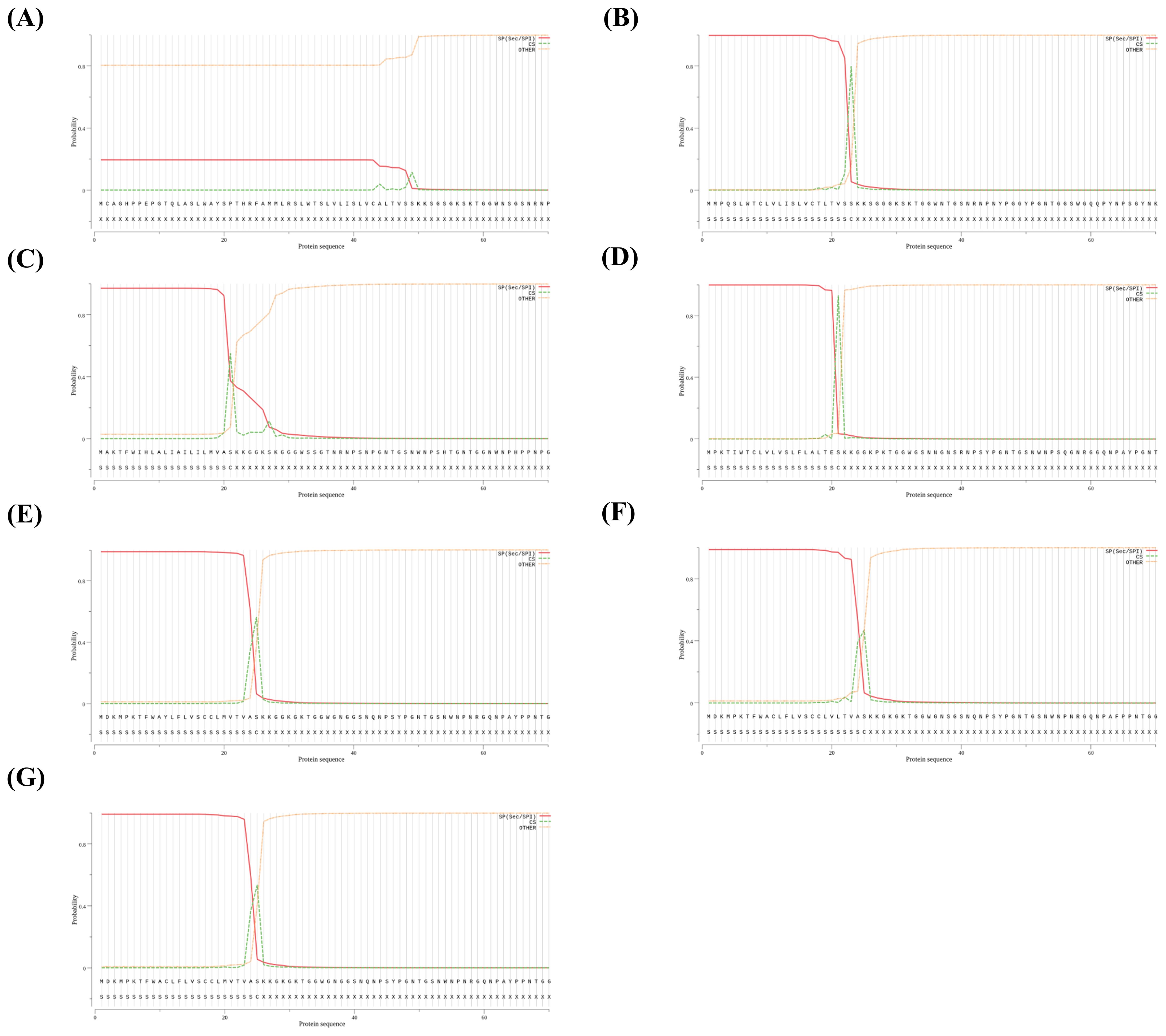
Figure 6. Signal peptide prediction using the SignalIP-5.0 server in 7 frogs. (A) The signal peptide prediction of Xenopus tropicalis (Western clawed frog). (B) The signal peptide prediction of Xenopus laevis (African clawed frog). (C) The signal peptide prediction of Hyla sarda (Sardinian tree frog). (D) The signal peptide prediction of Nanorana parkeri (high Himalaya frog). (E) The signal peptide prediction of Rana temporaria (common frog). (F) The signal peptide prediction of Lithobates catesbeianus (American bullfrog). (G) The signal peptide prediction of Rana dybowskii (Dybowski’s frog). Each lane indicates probability of signal peptide-related structure (signal peptide: red lane; cleavage site: green lane; yellow lane: no signal peptide).
4 Discussion
Dybowski’s frog is native to East Asia, including Korea, Japan, Russia, and China, and is classified as a species of “Near Threatened” on the international union for conservation of nature (IUCN) red list (https://www.iucn.org/). Dybowski’s frog is farmed for meat and as a health food in Korea, and is considered as climate-sensitive biological indicator species by the Korean National Institute of Biological Resources (NIBR, Incheon, Korea) (https://www.nibr.go.kr/cmn/main/enMain.do). The American bullfrog is a large North American species that is native to the United States and Eastern Canada and was first brought to Korea in the 1970s for food purposes, but became an invasive species that disrupts the ecosystem and has rapidly proliferated through escapes from breeding facilities and unauthorized release (Koo and Choe, 2021). Since both frog species are widely used as food in Korea, and pathogenesis of prion diseases are mainly mediated by the gastrointestinal tract, further research on the characteristics of the PrP in these species is necessary.
In the present study, we present the first report of frog PRNP gene sequences in Dybowski’s frog and the American bullfrog (Figure 1). None of the frog PrPs have a tandem repeat domain (Figure 2). In mammals, there are conserved tandem repeat domains (octapeptide repeat, PHGGGWGQ) related to copper binding that play pivotal roles in copper homeostasis (Brown et al., 1997; Garnett and Viles, 2003). Although bird species have a significantly different form of the tandem repeat domain (hexapeptide repeat, NPGYPH), chicken has twice the number of amino acids (histidine, major copper binding amino acid) in the tandem repeat region (Kim et al., 2018; Jeong et al., 2021; Kim et al., 2022). Since tandem repeat regions of mammalian and bird PrPs are pivotal in copper-related physiology, frog species may use other proteins for copper-related physiology. Further research is needed to identify the proteins responsible for the copper-related physiology of frogs in the context of the absence of the tandem repeat region of frog PrP (Strumbo et al., 2001).
In the present study, we observed abnormally high aggregation propensity of frog PrP in the high Himalaya frog (Figure 5). Currently, there are no confirmed reports specifically identifying frogs as candidate organisms for the transmission of prion diseases. Most research has focused on mammals, particularly livestock such as cattle and deer, which are known to be susceptible to prion infections (Orge et al., 2021). However, given the consumption of amphibians like frogs in some regions, and the wide range of species that may be affected by prions, it is important to investigate their potential role in prion transmission. This study identified potential candidates carrying a predicted high aggregation propensity of frog PrP. Therefore, future studies are necessary to validate the prion-forming ability of frog PrP using in vivo and in vitro systems to explore the possibility of prion seeding in frogs.
Even though PrPs show low aggregation in some frog species (Sardinian tree frog, American bullfrog), they can show high PrP aggregation formation ability depending on genetic polymorphisms of the PRNP gene. Previous studies have reported that genetic polymorphisms and germline mutations of the PRNP gene are significantly related to the pathomechanisms of several types of prion disease. In humans, over 30 mutations (V180I, E200K, V203I, etc.) are widely reported in genetic Creutzfeldt–Jakob disease (CJD). In addition, M129V and E219K confer resistance to variant and sporadic CJD (Jackson and Clarke, 2000; Takada et al., 2017). Furthermore, the G127V genotype of the PRNP gene confers complete resistance to kuru and in prion-inoculated transgenic mice (Asante et al., 2015). In artiodactyls including sheep, goats, deer and elk, genetic polymorphisms of the PRNP gene are significantly associated with vulnerability to prion diseases. Those mutations and genetic polymorphisms impact the tertiary structure of PrP and regulate the amyloid propensity of PrPSc (Sanchez-Garcia and Fernandez-Funez, 2018; Myers et al., 2020). Since several studies have suggested that genetic variations of PrPs serve as seeds to spread prion diseases, additional research is needed to evaluate the possibility that frog PrPs will become prion seeds in the future. In addition, conducting further studies involving additional laboratory experiments to determine the potential susceptibility of amphibians to mammalian prions would provide valuable information for academia and the public.
A previous study reported that frogs do not have the “AGAAAAGA” palindromic sequence of PrP. The palindromic sequence is the PrPC-PrPSc interaction region, which plays a crucial role in the conversion of PrPC to PrPSc (Norstrom and Mastrianni., 2005). Although the exact palindromic sequence has not been identified in frog species, we observed palindromic sequences the were partially conserved in all frog species (Figure 2). The palindromic sequence plays a pivotal role in the conversion of PrPC to PrPSc, and experimental disruption of the palindromic sequence inhibits the prion conversion process. Since the partially conserved palindromic sequence may be important in the prion pathology of frogs, research is needed to determine whether this partially conserved palindromic sequence can interact with PrPSc in the future.
We also found significantly different sequence homologies of amino acid sequences among frog species (Figure 2). In particular, the N-terminal signal peptide was not predicted in the western clawed frog (Figure 6). This region (~25 amino acids at the N-terminal) is not conserved across species (Figure 2). It may be a western clawed frog-specific sequence, and this region could play a crucial role in influencing the specific structure or stability of the protein. There is a need for further research to validate the structure or function in the future. PrPs are mainly expressed as glycosylphosphatidylinositol (GPI) anchor proteins on the cell surface and have an N-terminal signal peptide that is conserved across species (Mead et al., 2019). Since the N-terminal signal peptide was not observed in western clawed frogs, further study is needed for PrP localization in the western clawed frog.
In Korea, CWD was introduced by the importation of asymptomatic CWD-infected elk. Subsequently, CWD was reported in elk, red deer, and sika deer across the country (Sohn et al., 2020). Except for CWD, there are no reports of animal prion diseases, including scrapie and BSE, in South Korea. In Japan, BSE was first reported in 2001, and the country has the largest number of BSE cases outside Europe (Yamanouchi and Yoshikawa, 2007). Additionally, classical scrapie has been reported in sheep, and atypical scrapie in goats. However, the exact epidemiology remains unclear (Matsuura et al., 2019; Onodera and Saeki, 2000). Although Dybowski’s frog and the American bullfrog have relatively low amyloid propensity (Figure 5), since soil and water can easily become contaminated by scrapie and CWD prions derived from the body fluids of prion-infected animals, these environmental factors may play a pivotal role in the mode of transmission for frogs. Further research on the relationship between prion and mode of transmission for frogs is highly desirable in the future.
In the present study, we present the first report of DNA sequences of the PRNP gene in Dybowski’s frog and the American bullfrog. In addition, we analyzed sequence similarity and evolutionary relationships among frog species and found partially conserved palindromic sequence (PrPC-PrPSc interaction region) and absence of the tandem repeat region among several frog species. We analyzed protein structures of frog species and found that high Himalaya frog has abnormally high aggregation propensity and the western clawed frog does not have the N-terminal signal peptide. To the best of our knowledge, this was the first genetic comparative study among frog species relevant to the potential spread of prion diseases.
Data availability statement
The datasets presented in this study can be found in online repositories. The names of the repository/repositories and accession number(s) can be found in the article/Supplementary Material.
Ethics statement
The animal study was approved by Institutional Animal Care and Use Committee of Andong National University. The study was conducted in accordance with the local legislation and institutional requirements.
Author contributions
S-YW: Data curation, Formal analysis, Investigation, Writing – original draft, Writing – review & editing. Y-CK: Conceptualization, Data curation, Formal analysis, Project administration, Validation, Writing – original draft, Writing – review & editing.
Funding
The author(s) declare financial support was received for the research, authorship, and/or publication of this article. This work was supported by a Research Grant of Andong National University.
Conflict of interest
The authors declare that the research was conducted in the absence of any commercial or financial relationships that could be construed as a potential conflict of interest.
Publisher’s note
All claims expressed in this article are solely those of the authors and do not necessarily represent those of their affiliated organizations, or those of the publisher, the editors and the reviewers. Any product that may be evaluated in this article, or claim that may be made by its manufacturer, is not guaranteed or endorsed by the publisher.
Supplementary material
The Supplementary Material for this article can be found online at: https://www.frontiersin.org/articles/10.3389/fanim.2024.1457653/full#supplementary-material
Supplementary Figure 1 | Gel electrophoresis of polymerase chain reaction of the frog prion protein gene (PRNP) of Rana dybowskii (Dybowski’s frog) and Lithobates catesbeianus (American bullfrog). Lanes 1-2 are Dybowski’s frog, while lanes 3-4 are American bullfrog. Lane M, 100 bp DNA ladder.
Supplementary Figure 2 | Dorsal and ventral views of the Rana dybowskii (Dybowski’s frog) and Lithobates catesbeianus (American bullfrog). (A) Dorsal views of both species. (B) Ventral views of both species. Left: Rana dybowskii (Dybowski’s frog); Right: Lithobates catesbeianus (American bullfrog).
References
Anderson R., Donnelly C., Ferguson N., Woolhouse M., Watt C., Udy H., et al. (1996). Transmission dynamics and epidemiology of BSE in British cattle. Nature 382, 779–788. doi: 10.1038/382779a0
Appleby B. S., Shetty S., Elkasaby M. (2022). Genetic aspects of human prion diseases. Front. Neurol. 13. doi: 10.3389/fneur.2022.1003056
Asante E. A., Smidak M., Grimshaw A., Houghton R., Tomlinson A., Jeelani A., et al. (2015). A naturally occurring variant of the human prion protein completely prevents prion disease. Nature 522, 478–481. doi: 10.1038/nature14510
Baron T. G., Madec J.-Y., Calavas D. (1999). Similar signature of the prion protein in natural sheep scrapie and bovine spongiform encephalopathy-linked diseases. J. Clin. Microbiol. 37, 3701–3704. doi: 10.1128/JCM.37.11.3701-3704.1999
Baylis M., Goldmann W. (2004). The genetics of scrapie in sheep and goats. Curr. Mol. Med. 4, 385–396. doi: 10.2174/1566524043360672
Bons N., Mestre-Frances N., Belli P., Cathala F., Gajdusek D. C., Brown P. (1999). Natural and experimental oral infection of nonhuman primates by bovine spongiform encephalopathy agents. Proc. Natl. Acad. Sci. U. S. A 96, 4046–4051. doi: 10.1073/pnas.96.7.4046
Bons N., Mestre-Francés N., Charnay Y., Tagliavini F. (1996). Spontaneous spongiform encephalopathy in a young adult rhesus monkey. Lancet 348, 55. doi: 10.1016/s0140-6736(96)24027-9
Brown D. R., Qin K., Herms J. W., Madlung A., Manson J., Strome R., et al. (1997). The cellular prion protein binds copper in vivo. Nature 390, 684–687. doi: 10.1038/37783
Bruce M. E., Will R. G., Ironside J., Mcconnell I., Drummond D., Suttie A., et al. (1997). Transmissions to mice indicate that ‘new variant’CJD is caused by the BSE agent. Nature 389, 498–501. doi: 10.1038/39057
Calzolai L., Lysek D. A., Pérez D. R., Güntert P., Wüthrich K. (2005). Prion protein NMR structures of chickens, turtles, and frogs. Proc. Natl. Acad. Sci. U. S. A 102, 651–655. doi: 10.1073/pnas.0408939102
Davies G. A., Bryant A. R., Reynolds J. D., Jirik F. R., Sharkey K. A. (2006). Prion diseases and the gastrointestinal tract. Can. J. Gastroenterol. 20, 18–24. doi: 10.1155/2006/184528
Foliaki S. T., Groveman B. R., Yuan J., Walters R., Zhang S., Tesar P., et al. (2020). Pathogenic prion protein isoforms are not present in cerebral organoids generated from asymptomatic donors carrying the E200K mutation associated with familial prion disease. Pathogens 9, 482. doi: 10.3390/pathogens9060482
Gabriel J.-M., Oesch B., Kretzschmar H., Scott M., Prusiner S. B. (1992). Molecular cloning of a candidate chicken prion protein. Proc. Natl. Acad. Sci. U. S. A 89, 9097–9101. doi: 10.1073/pnas.89.19.9097
Garnett A. P., Viles J. H. (2003). Copper binding to the octarepeats of the prion protein: affinity, specificity, folding, and cooperativity: insights from circular dichroism. J. Biol. Chem. 278, 6795–6802. doi: 10.1074/jbc.M209280200
Harrison P. M., Khachane A., Kumar M. (2010). Genomic assessment of the evolution of the prion protein gene family in vertebrates. Genomics 95, 268–277. doi: 10.1016/j.ygeno.2010.02.008
Jackson G. S., Clarke A. R. (2000). Mammalian prion proteins. Curr. Opin. Struct. Biol. 10, 69–74. doi: 10.1016/s0959-440x(99)00051-2
Jeong M.-J., Kim Y.-C., Jeong B.-H. (2021). The first report of the Prion Protein Gene (PRNP) sequence in pekin ducks (Anas platyrhynchos domestica): the potential Prion disease susceptibility in ducks. Genes (Basel) 12, 193. doi: 10.3390/genes12020193
Kim Y.-C., Jeong M.-J., Jeong B.-H. (2018). The first report of genetic variations in the chicken prion protein gene. Prion 12, 197–203. doi: 10.1080/19336896.2018.1471922
Kim Y.-C., Won S.-Y., Jeong B.-H. (2021). Altered expression of glymphatic system-related proteins in prion diseases: Implications for the role of the glymphatic system in prion diseases. Cell. Mol. Immunol. 18, 2281–2283. doi: 10.1038/s41423-021-00747-z
Kim Y., Kim Y.-C., Jeong B.-H. (2022). Novel single nucleotide polymorphisms (SNPs) and genetic features of the prion protein gene (PRNP) in quail (Coturnix Japonica). Front. Vet. Sci. 9. doi: 10.3389/fvets.2022.870735
Kim Y., Lee J., Lee C. (2008). In silico comparative analysis of DNA and amino acid sequences for prion protein gene. Transbound Emerg. Dis. 55, 105–114. doi: 10.1111/j.1865-1682.2007.00997.x
Kirkwood J., Cunningham A. A. (1994). Epidemiological observations on spongiform encephalopathies in captive wild animals in the British Isles. Vet. Rec 135, 296–303. doi: 10.1136/vr.135.13.296
Koo K. S., Choe M. (2021). Distribution Change of Invasive American Bullfrogs (Lithobates catesbeianus) by Future Climate Threaten Endangered Suweon Treefrog (Hyla suweonensis) in South Korea. Anim. (Basel) 11. doi: 10.3390/ani11102865
Kupfer L., Hinrichs W., Groschup M. (2009). Prion protein misfolding. Curr. Mol. Med. 9, 826–835. doi: 10.2174/156652409789105543
Lasmezas C., Deslys J. P., Demaimay R., Adjou K., Lamoury F., Dormont D., et al. (1996). BSE transmission to macaques. Nature 381, 743–744. doi: 10.1038/381743a0
Lasmézas C. I., Fournier J.-G., Nouvel V., Boe H., Marcé D., Lamoury F., et al. (2001). Adaptation of the bovine spongiform encephalopathy agent to primates and comparison with Creutzfeldt–Jakob disease: implications for human health. Proc. Natl. Acad. Sci. U. S. A 98, 4142–4147. doi: 10.1073/pnas.041490898
Marsh R. F., Hadlow W. (1992). Transmissible mink encephalopathy. Rev. Sci. Tech 11, 539–550. doi: 10.20506/rst.11.2.606
Marsh R. F., Kincaid A. E., Bessen R. A., Bartz J. C. (2005). Interspecies transmission of chronic wasting disease prions to squirrel monkeys (Saimiri sciureus). J. Virol. 79, 13794–13796. doi: 10.1128/JVI.79.21.13794-13796.2005
Matsuura Y., Miyazawa K., Imamura M., Yokoyama T., Iwamaru Y. (2019). First case of atypical scrapie in a goat in Japan. J. Vet. Med. Sci. 81, 986–989. doi: 10.1292/jvms.18-0710
Mead S., Lloyd S., Collinge J. (2019). Genetic factors in mammalian prion diseases. Annu. Rev. Genet. 53, 117–147. doi: 10.1146/annurev-genet-120213-092352
Moazami-Goudarzi K., Andréoletti O., Vilotte J.-L., Béringue V. (2021). Review on PRNP genetics and susceptibility to chronic wasting disease of Cervidae. Vet. Res. 52, 128. doi: 10.1186/s13567-021-00993-z
Myers R., Cembran A., Fernandez-Funez P. (2020). Insight from animals resistant to prion diseases: Deciphering the genotype–morphotype–phenotype code for the prion protein. Front. Cell. Neurosci. 14. doi: 10.3389/fncel.2020.00254
Nichols J. (2014). Literary anecdotes of the eighteenth century. (United Kingdom: Cambridge University Press).
Norstrom E. M., Mastrianni J. A. (2005). The AGAAAAGA palindrome in PrP is required to generate a productive PrPSc-PrPC complex that leads to prion propagation. J. Biol. Chem. 280, 27236–27243. doi: 10.1074/jbc.M413441200
Orge L., Lima C., MaChado C., Tavares P., Mendonça P., Carvalho P., et al. (2021). Neuropathology of animal prion diseases. Biomolecules 11, 466. doi: 10.3390/biom11030466
Pritzkow S., Morales R., Lyon A., Concha-Marambio L., Urayama A., Soto C. (2018). Efficient prion disease transmission through common environmental materials. J. Biol. Chem. 293, 3363–3373. doi: 10.1074/jbc.M117.810747
Pritzkow S., Morales R., Moda F., Khan U., Telling G. C., Hoover E., et al. (2015). Grass plants bind, retain, uptake, and transport infectious prions. Cell. Rep. 11, 1168–1175. doi: 10.1016/j.celrep.2015.04.036
Prusiner S. B., Dearmond S. J. (1994). Prion diseases and neurodegeneration. Annu. Rev. Neurosci. 17, 311–339. doi: 10.1146/annurev.ne.17.030194.001523
Sanchez-Garcia J., Fernandez-Funez P. (2018). D159 and S167 are protective residues in the prion protein from dog and horse, two prion-resistant animals. Neurobiol. Dis. 119, 1–12. doi: 10.1016/j.nbd.2018.07.011
Sohn H.-J., Mitchell G., Lee Y. H., Kim H. J., Park K.-J., Staskevicus A., et al. (2020). Experimental oral transmission of chronic wasting disease to sika deer (Cervus nippon). Prion 14, 271–277. doi: 10.1080/19336896.2020.1857038
Strumbo B., Ronchi S., Bolis L. C., Simonic T. (2001). Molecular cloning of the cDNA coding for Xenopus laevis prion protein. FEBS. Lett. 508, 170–174. doi: 10.1016/s0014-5793(01)03027-7
Takada L. T., Kim M. O., Cleveland R. W., Wong K., Forner S. A., Gala I. I., et al. (2017). Genetic prion disease: experience of a rapidly progressive dementia center in the United States and a review of the literature. Am. J. Med. Genet. B. Neuropsychiatr. Genet. 174, 36–69. doi: 10.1002/ajmg.b.32505
Yamanouchi K., Yoshikawa Y. (2007). Bovine spongiform encephalopathy (BSE) safety measures in Japan. J. Vet. Med. Sci. 69, 1–6. doi: 10.1292/jvms.69.1
Keywords: amyloid, BSE, CJD, CWD, frog, prion, PRNP, PrP
Citation: Won S-Y and Kim Y-C (2024) The first report of prion protein gene sequences in Dybowski’s frog and the American bullfrog: high amyloid propensity of the frog prion protein. Front. Anim. Sci. 5:1457653. doi: 10.3389/fanim.2024.1457653
Received: 01 July 2024; Accepted: 24 September 2024;
Published: 09 October 2024.
Edited by:
Andrew C. Gill, Nottingham Trent University, United KingdomReviewed by:
Amod Kumar, National Bureau of Animal Genetic Resources (NBAGR), IndiaMartina Miluchová, Slovak University of Agriculture, Slovakia
Copyright © 2024 Won and Kim. This is an open-access article distributed under the terms of the Creative Commons Attribution License (CC BY). The use, distribution or reproduction in other forums is permitted, provided the original author(s) and the copyright owner(s) are credited and that the original publication in this journal is cited, in accordance with accepted academic practice. No use, distribution or reproduction is permitted which does not comply with these terms.
*Correspondence: Yong-Chan Kim, kych@anu.ac.kr