- 1Faculty of Chemistry, Biotechnology and Food Science, Norwegian University of Life Sciences, Ås, Norway
- 2Faculty of Biosciences, Norwegian University of Life Sciences, Ås, Norway
The last century has witnessed many innovations in agriculture and food technologies that have ensured the production of sufficient quantities of good hygienic food. Animal scientists have contributed substantially to efficient breeding and feeding practices by adapting animals for faster growth and improving feed efficiency and utilization. Breeding goals and feeding recommendations have been proposed with a primary focus on profitability to promote significant changes in the macronutrient content, i.e., animal body protein and fat reduction. However, improving the nutritional quality of meat was not included in the profit assessment. Increasing the lean meat fraction is consistent with the goal of public dietary guidelines for human nutrition proposed in 1980, emphasizing the importance of reducing the consumption of animal fat, particularly saturated fat. The application of breeding and feeding tools to modify and improve the fatty acid composition has been partly implemented in pigs and broiler chickens to supplement the dietary recommendations for humans. The health benefits of lean “red meat” have been questioned in recent years, with dietary guidelines and upper limits being introduced for human intake. Animal breeding indirectly reduces the total and heme iron (the redness generator) content in meat, due to covariation with priority breeding goals. Two micronutrients play important roles in the human diet and are derived largely from meat (selenium) and milk (iodine) if the amount provided and absorbed is sufficient and predictable. The iodine content can be highly dependent on the use of novel (more sustainable) feeds. The micronutrients discussed in this study (Fe, Se, I, and vitamin D) highlight opportunities for the utilization of breeding and feeding knowledge to adjust their levels to procure meat with a high nutrient density. The gaps in micronutrient levels in humans must be addressed by navigating within approved animal feeding levels. Animal scientists must recognize the nutritional impact of breeding and feeding and advertise them. In addition, human nutritionists must acknowledge the existing and potential changes in animal production to meet the dietary guidelines. Sustainable food production within the “One Health” concept can only be achieved through cooperation.
1 Introduction
Selective breeding has been practiced since the 18th century to improve carcass quality and meat production; however, significant changes have been reported in this practice since 1960 (Siegel, 2014; Knap and Wang, 2012). Larger carcasses, more milk, meat production with less fat, and improved feed efficiency have remained the focus of animal selection. Breeding and efficient feed utilization have yielded more animal products in recent years, sparring debates on the health and well-being of the animals (Tixier-Boichard, 2018). Access to greater amounts of food through efficient agricultural practices, innovative food technologies, and improved nutrition have facilitated the increase in population from a few million during the hunter-gathering period to eight billion at present.
The rapid growth in the production of farmed animals in the 1970s has increased the focus on sustainability. Revising agricultural practices to facilitate the incorporation of more plant-based food in the human diet, reducing the consumption of meat, and use more non-edible and novel resources as feed was an important approach of the sustainability period (1970–present).
Awareness regarding the importance of food and nutrition has increased over the last century. The period from 1950 to 1970 is important in the human nutrition timeline due to the increased focus on protein malnutrition as a major global challenge (Mozaffarian et al., 2018). Food energy surpluses (fat- and starch-rich products) were prevalent in many industrialized countries in the 1980s. Nutritional guidelines have warned against the high intake of animal fat since the late 1970s, with extensive studies being conducted on this topic. Reducing the intake of lean “red meat” has been recommended by nutritional guidelines, with an upper level (UL) provided from around 2015.
Micronutrient deficiency (hidden hunger) is a global challenge that has persisted for centuries. However, the combination of hidden hunger and energy surplus is a more recent challenge. The situation has been associated with a greater availability of energy rich and nutrient poor industrial foods. Although not a pressing topic at present, micronutrient dilution of raw materials may be a future challenge. Thus, in addition to increasing meat healthiness and nutrient density, improving the efficiency and use of more sustainable or novel feeds is also important.
This article reflects on the measures taken in the domain of animal breeding and feeding guidelines over the past 100 years through international and Norwegian/Nordic loops. Guidelines have been formulated for the nutritional requirements of human beings. Thus, the role of food categories (such as meat and grains) in the human diet was also surveyed in this study. The changes in the fat and Fe levels represent historical changes in animal breeding practices. The Se and I levels represent the dominant choice of animal feeding practices. Changes in the production and feeding practices lead to changes in vitamin D levels. Thus, detailed summaries of fat, a macronutrient, and these four micronutrients (Fe, Se, I, and vitamin D) have been provided in this article. Finally, measures to maintain the nutrient-dense nature of animal products, which may provide health benefits, have also been proposed.
2 Changes in meat and milk composition through efficient breeding and feeding in the last century
2.1 Advances in improving the quality of carcass and meat composition through breeding in the last century
The inheritance of genes was described by the Austrian monk Gregor Mendel in 1860. Sewall Wright, along with Ronald Fisher and John B.S. Haldane, established the theory behind breeding in populations (Larson, 2006) as the basis for selecting animals to maximize genetic gain for certain traits in a population in the 1920s. A model for applied animal breeding was established based on these theories (Lush, 1931), with breeding programs increasing animal production and efficiency over the next few decades (Figure 1). Traits that increase the growth rate and lean meat deposition have been favored in the domain of meat production as they improve feed efficiency and enable the production of more meat per kilogram of feed consumed. The implementation of these breeding programs has yielded successful outcomes in all species, particularly in poultry.

Figure 1. Overview of the historical development in animal genetic and dietary changes. Impact of breeding programs and the development of knowledge regarding animal health and feed efficiency on use and guidelines at USA (National Research Council, NRC) and Norwegian levels with a focus on four micronutrients (Fe, Se, I, and vitamin D).
To the best of our knowledge, the nutritional quality of meat has not been considered an economically valuable trait; thus, it is not acknowledged in breeding programs. Leaner meat is considered favorable economically and nutritionally. However, breeding techniques to facilitate faster growth and the production of leaner meat have changed genetic characteristics and favored an increase in the prevalence of larger glycolytic muscle cells over smaller oxidative muscle cells in broiler chickens. This reduces the inclusion of Fe and Se in muscle cells (Thiruvenkadan et al., 2019). Similarly, realizing that the pig is sufficiently lean has increased the focus on dressing percentage, meat quality, reproduction, and animal health without considering the changes in nutritional quality occurring over time. The benefits of consuming pork meat have increased. However, the amount of unsaturated fatty acids in pork has not been adequately acknowledged. The subsequent sections will discuss this point.
2.2 Advances in feeding standards and recommendations in the last century
The first feed standard was introduced by the German scientist Thaer (1754–1828) in 1810, who compared potential feedstuffs with meadow hay (Coffey et al., 2016). Weende analysis (“the proximate analysis”) of main nutrients, along with the pioneering work conducted by Wolff, Armsby, Kellner, and others on the digestibility and energetic contribution, have facilitated the formulation of basic principles for feed evaluation in the second half of the 19th century. These guidelines continued to be used over 150 years later (Weisbjerg et al., 2010). The National Research Council (NRC) set forth the first edition of its recommendations in 1944 in the USA (Figure 1). The NRC recommendations remain the global standard for all domestic animals, including pets and fish. However, the EU recommendations set forth by the European Food Safety Authority (EFSA) in 2002 are followed in Norway (Figure 1). The EU recommendations have defined risks and proposed upper limits (UL) for human food and animal feed, including health and welfare. Thus, this organization influences the achievements in sustainability. Notably, the influence of some nutrients on product quality has been defined in the One Health concept (Mackenzie and Jeggo, 2011) in Europe. For instance, I and Se have been incorporated in animal feed to ensure the minimum or maximum transfer of these elements into human food in accordance with these recommendations.
2.3 Alteration of animal carcass and meat and milk composition through breeding and feeding
Mozaffarian et al. (2018) stated that “relatively few rigorous evaluations have been conducted on the potential long-term health consequences of modern shifts in agricultural practices (breeding, feeding, and food processing)”. This section discusses the variability in the total fat (TF) composition in the carcass, its derived fatty acid (FA) content, and the four micronutrients that play important roles in maintaining human health.
2.3.1 Total fat and fatty acids in meat
Energy deficiency was more prevalent among humans in previous decades. Consequently, the TF content was considered more important than the quantity of lean meat and quality of fat. However, the increased focus on incorporating high-quality protein in the human diet and increasing awareness regarding the health benefits of certain FAs, along with the strong focus on feed utilization and production costs in terms of quantity and quality, especially pig fat, have changed dramatically since 1950. This reduction has been attributed to the efficient breeding and selection of animals using progeny testing, with an emphasis on the traits linked to the growth rate and feed conversion ratio (FCR), such as carcass dissection, backfat measurements, and body composition determined via computed tomography (CT). The Food and Agriculture Organization/World Health Organization (FAO/WHO) has acknowledged the large changes in TA and FA composition via genetics, feeding, and trimming techniques (FAO, 2010). However, the overarching relevance of transitioning to the consumption of healthier meat is underexplored.
Genetic changes in the quantity and quality of fat have been achieved via long-term shifts. Genotyping, which has replaced progeny testing, has enabled a significant improvement in the speed of genetic gain over the past 20–30 years. Emerging genetic technologies may reduce the time required to achieve quality improvement. The short-term shifts, independent of the “permanent” genetic changes, can be traced back to feeding. The FA composition of monogastric animals is easily affected by feeding as the body fat reflects the FA composition of the feed. As such, substantial knowledge exists for designing the quality of monogastric meat according to potential demands. Section 4 highlights examples related to the influence of breeding and feeding on the TF and FA composition of meat in monogastric animals.
2.3.2 Content of selected micronutrients in meat and milk
Micronutrients are not the primary targets in breeding programs, and genetic changes are the consequence of correlations with traits in breeding indexes. However, breeding and feeding techniques have differential effects on the micronutrient content in meat. Selected essential micronutrients, such as Fe, Se, I, and vitamin D, in feeding protocols within a historical framework for dairy cows, beef cattle, fattening pigs, and broilers have been considered in this study to illustrate this. Table 1 summarizes the main dietary sources and feeding recommendations provided in Europe, the USA (NRC), and Norway/Nordic countries.
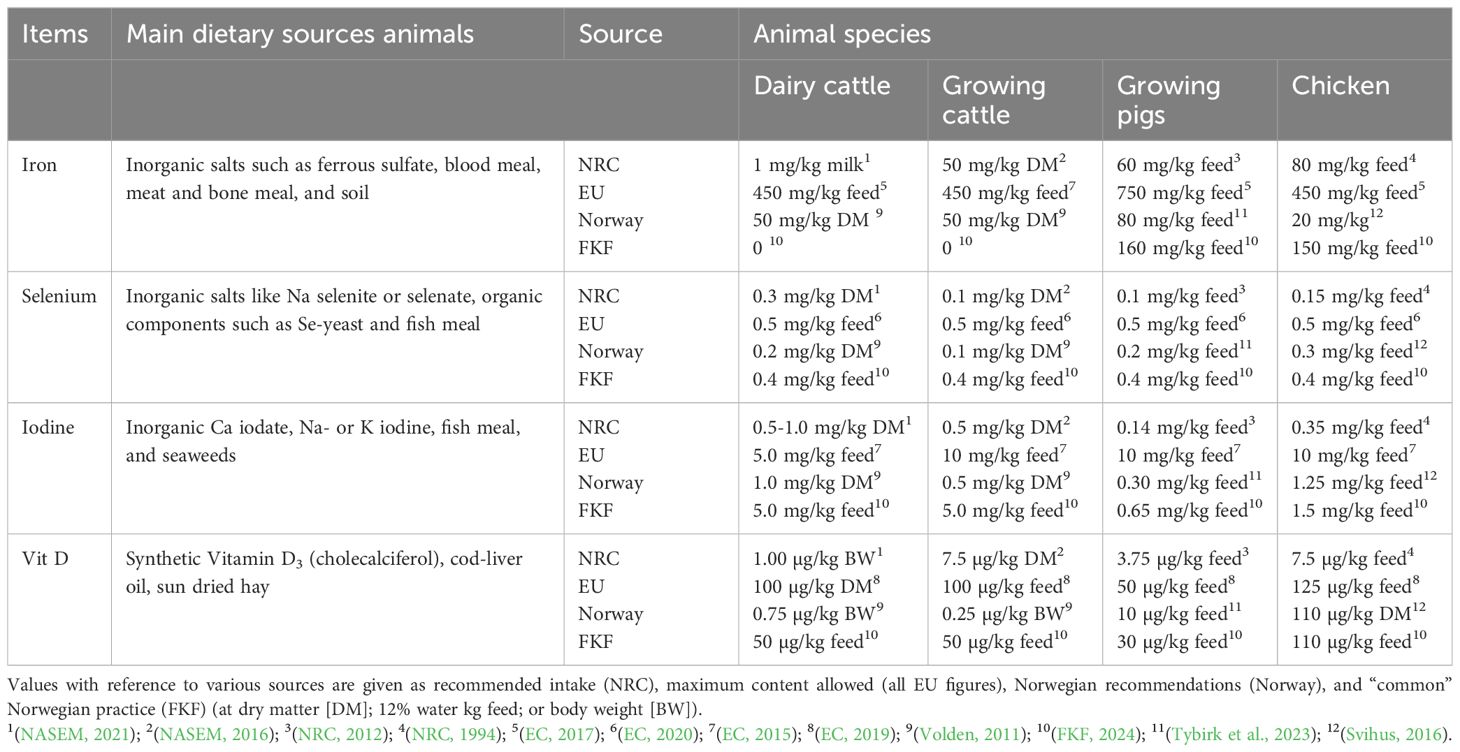
Table 1. Aspects related to animal feed dietary sources and animal recommendations of intake or supply through feed for four micronutrients.
2.3.2.1 Iron
The role of dietary Fe in the prevention of Fe deficiency in young animals has been known since the early 1930s (Blaxter et al., 1957), and animal feed has been supplemented with Fe for more than a century. Commonly used Fe sources have poor bioavailability; consequently, Fe is supplemented in excess to achieve adequate intake (AI). However, Fe is efficiently recycled in the body, and tissue growth is the main factor affecting the requirement for Fe. The fifth revised edition of the NRC for poultry (NRC, 1966) recommended supplementing the feed for starter chickens (0–8 weeks) with 40 mg Fe/kg; however, this was increased to 80 mg/kg in the 1971 revision (NRC, 1971b). The 1979 edition (NRC, 1979) recommended supplementing the feed for growing pigs (20–120 kg BW) with 40–60 mg Fe/kg. The 1971 revision recommended supplementing the feed for all types of dairy cattle with 100 mg Fe/kg; however, this was reduced to 50 mg Fe/kg in 1978 (NRC, 1978). The current recommendations set forth by the NRC and EFSA reflect these figures (Table 1). The NRC recommendations compensate for the expected milk loss (1 mg/kg milk) in adult dairy cows only (NASEM, 2021).
The addition of Fe to ruminant, pig, and chicken feeds was mandated in Norway when feeding standards were introduced in 1950, 1957, and 1972, respectively (Homb, 1979) (Figure 1). Presently, ruminant feed is not usually supplemented with Fe as Fe intake from natural sources is considered sufficient. However, pig and poultry feeds are supplemented with 160 and 150 mg Fe/kg, respectively (FKF, 2024) (Table 1). This amount is twice that recommended by the NRC, but is well within the maximum content recommended in the EU. Although the upper tolerable limit is high, it is normally not a source of concern in animal feeding. Blood meal and meat and bone meal are rich in Fe; however, they are not commonly used in the feeds for farm animals due to disease risks.
2.3.2.2 Selenium
Selenium is a scarce trace element. The Se content in plants reflects the Se content in the soil. Feed ingredients cultivated in the central plains of the USA and Canada are usually rich in Se. In contrast, the soil and thus plants in most European regions, including the Nordic region, are low in Se content. Selenium was recognized as an essential element in the late 1950s. The NRC has recommended supplementation of Se from the mid-1960s; however, supplementation of poultry and pig feed and later ruminant feed was first approved by the FDA in 1974 (Brummer et al., 2014). Se content in the range of 0.03–0.05 mg/kg feed is considered “marginal” in the UK (ARC, 1980). Notably, 0.1 mg Se/kg was required for the optimal activity of glutathione peroxidase (GPx) in milk-fed lamb, which corresponded with NRC recommendations for dairy cattle (NRC, 1971a, 1978).
The NRC currently recommends increasing the Se content to 0.3 mg Se/kg dry matter (DM) feed for dairy cattle (Table 1). The NRC recommends supplementing pig and poultry feed with 0.10 and 0.15 mg Se/kg DM; in contrast, the EU has limited the Se content to ≤0.5 mg/kg feed for all species (Table 1). Inorganic supplements are used most commonly; however, interest in organic supplements, such as Se-yeast, has been increasing in recent years, and they facilitate more efficient transfer into meat (Malbe et al., 1995; Ortman and Pehrson, 1999; Cruickshank et al., 2021). Thus, the maximum Se content was limited to 0.2 mg/kg feed when using organic supplements (EFSA, 2021). The NRC and EFSA list the upper tolerable level as 5 mg Se/kg feed.
The addition of 0.1 mg Se/kg feed in all animal feed was mandated in 1979 in Norway (Figure 1). The concentration was then increased to 0.2 mg/kg feed. However, 0.40–0.45 mg Se/kg is added to all compound feeds (Table 1) at present such that the upper 0.5 mg/kg feed limit is not exceeded. Fish meal is a good natural source of Se in animal feed. However, Se can also be supplied orally as boluses or tablets before slaughter to increase the Se content in the meat.
2.3.2.3 Iodine
Iodine is present in saltwater oceans and is at relatively high levels in fish meal. The I content in plants and soil diminishes as the distance from the sea increases. Animal feeds are supplemented with I in the form of iodide or iodate; notably, iodate which is more resistant to volatilization. The important role played by I in the proper functioning of the thyroid gland in vertebrates and humans has been reported since the early 1900s. The “Proceedings of the Iowa Academy of Science”, (Evvard and Culbertson, 1924) described I as “a Factor in Feeding Young Growing Swine,” citing several articles published between 1913 and 1924 indicating the requirement for I in animal diets. According to ARC (1980), Mitchell and McClure (1937) estimated requirements of non-lactating cows in the range of 0.03–0.07 mg/kg DM feed. The NRC set forth the first recommendations for I in chicken (0.20 mg/kg feed) and swine (0.22 mg/kg feed) feed in 1954 and 1953, respectively. The NRC recommended supplementing the feed of dairy cattle with 0.76 mg I/kg feed in 1958. However, the Norwegian ministry of Agriculture has recommended supplementation with I in dairy cattle through feed to prevent goiter in humans since 1951, considering the efficient transfer of I from feed to milk (Breirem and Homb, 1958). This recommendation corresponded to approximately 0.5 mg I/kg feed.
Current recommendations for the supplementation of I in animal feed can be classified as additions for the biofortification of milk and AI to meet the requirements of the animal. To meet the requirements of the animal, I is supplemented at 0.15–1.0 mg I/kg feed, with the highest level being supplied for goitrogenic diets (Table 1). Up to 5 mg I/kg feed can be used for the biofortification of milk; however, the upper recommended limit is 2 mg I/kg feed (EFSA, 2013). Concerns have been raised regarding an excessively high content of I in cow milk, which is not suitable for infants. The upper recommended intake is based on public health issues also in the USA, with the intake being set to 50 mg I/day in dairy cows by the NASEM (2021). This limit corresponds to the recommended limit of 2 mg I/kg feed in the diet of high-yielding cows (EFSA, 2013). Supplementation with up to 10 mg I/kg feed is permitted in the feed of growing cattle, pigs, and chickens in the EU (Table 1).
2.3.2.4 Vitamin D
Vitamin D3 is formed in the skin of most mammals in sunlight through the ultraviolet (UV) lighting of 7-dehydrocholesterol. The introduction of synthetic vitamin D in the 1950s and 1960s has led to cod liver oil and sun-cured hay being replaced as the main sources of vitamin D in animal feeds. The NRC has recommended the intake of vitamin D since its inception (1944) based on publications from the 1930s providing the understanding that Ca and P play essential roles in the normal development of the skeletal system. Initially, the feeds for chicken and pigs (20–120 kg) were supplemented with 2.2 and 5.0 µg vitamin D/kg, respectively (NRC, 1953, 1954). In 1950, the NRC recommended supplementation with 0.17 µg vitamin D/kg live animal body weight (BW) in beef cattle. In contrast, the NRC (1958) recommended supplementation with 0.050–0.075 µg vitamin D/kg BW for young heifers, whereas no recommendation was proposed for lactating cows. In UK, the ARC (1980) defined the requirement of vitamin D as 0.10 and 0.25 µg/kg BW in growing cattle and lactating cows, respectively. Current recommendations for AI of vitamin D vary across different species of animals, ranging from 30 to 125 µg/kg DM feed (Table 1), which are considerably higher than the early recommendations. The upper tolerable levels are high. Vitamin D levels of up to 1,000 µg/kg feed can be tolerated for shorter periods (EFSA, 2014), which is much higher than the present recommendations.
2.4 Sustainability and leaving traditional feeds
The strong focus on animal value chain sustainability-related matters in Europe (Figure 1) began in 1996 due to the bovine spongiform encephalopathy (BSE) crisis and the ban on the inclusion of mammalian meat and bone meal in animal feed in 2001 (Coffey et al., 2016). In addition, the increase in global population has led to an increased demand for protein, thereby increasing the requirement for water and land. Furthermore, achieving sustainable feed and food security, along with animal health and welfare, has become a key objective in Europe due to the reliance on soy imports and concerns about greenhouse gas emissions. The use of alternative protein sources, such as micro- and macro-algae, insects, single-cell proteins, and fermented products will facilitate animal food production in a sustainable manner (Makkar, 2018). Alternatives comprising plant matrices with high nutritional profiles and low environmental impacts (like hemp) have attracted further interest in recent years. The potential of novel or alternative feed sources (ingredients, additives, and functional ingredients) to facilitate the production of sustainable livestock is evaluated based on feed efficiency, environmental impact, and economic value (Halmemies-Beauchet-Filleau et al., 2018). However, the development of a strategy for sustainable livestock production is complex and includes accompanying risks, which are possibly reflected in the safety and nutritional value of animal products. The quality of meat and milk and the effects of manipulating their nutritional value using novel breeding tools remain to be elucidated. Furthermore, evidence supporting the changes in meat and milk composition induced by changes in feed composition and dietary treatments remains to be clarified. Therefore, characterizing the effects of alternative feed sources on the nutritive value of livestock is necessary. Section 4.4 exemplifies this through the renewed interest in using seaweed in animal diets.
3 The initiation and timelines of dietary guidelines for humans
The period during and after the Second World War emphasized the requirement for nutritional guidelines (Figures 2, 3). These guidelines were proposed officially in the late 1970s. Joint reports by the UN FAO/WHO often consider selected nutrients for nationwide assessment. The first relevant joint report focused on protein and energy intake (FAO/WHO, 1973). However, the FAO released reports on energy and protein requirements in 1950 (FAO, 1950) and 1958 (FAO, 1958). The first dietary guidelines (DGA) were released in 1980 (thereafter at 5-year intervals) in the USA. The first Nordic Nutrition Recommendations (NNR) were published in 1980 (thereafter at approximately 8-year intervals). The initiative to develop a joint NNR was based on local nutritional advice supported by Report No. 32 released in 1975 (Norwegian Parliament, 1975). The FAO/WHO releases joint reports on specific macronutrients and micronutrients; in contrast, individual countries or regions provide more comprehensive guidelines. Most dietary recommendations are similar and focus on nutrients, products, or raw materials. The focus on reducing the intake of saturated fat, salt, and sugar is clear. However, the Brazilian guidelines, set forth in 2015, introduced the concept of ultra-processed foods and a new classification system for such foods (Ministry of Health Brazil, 2015) to discourage the high intake of nutrient-poor foods that can contribute to lifestyle-related diseases (Dicken and Batterham, 2022). Established in 2002, the EFSA advises the EU on food safety and nutrition in the complete (plant and animals) food chain (EFSA, 2024b).
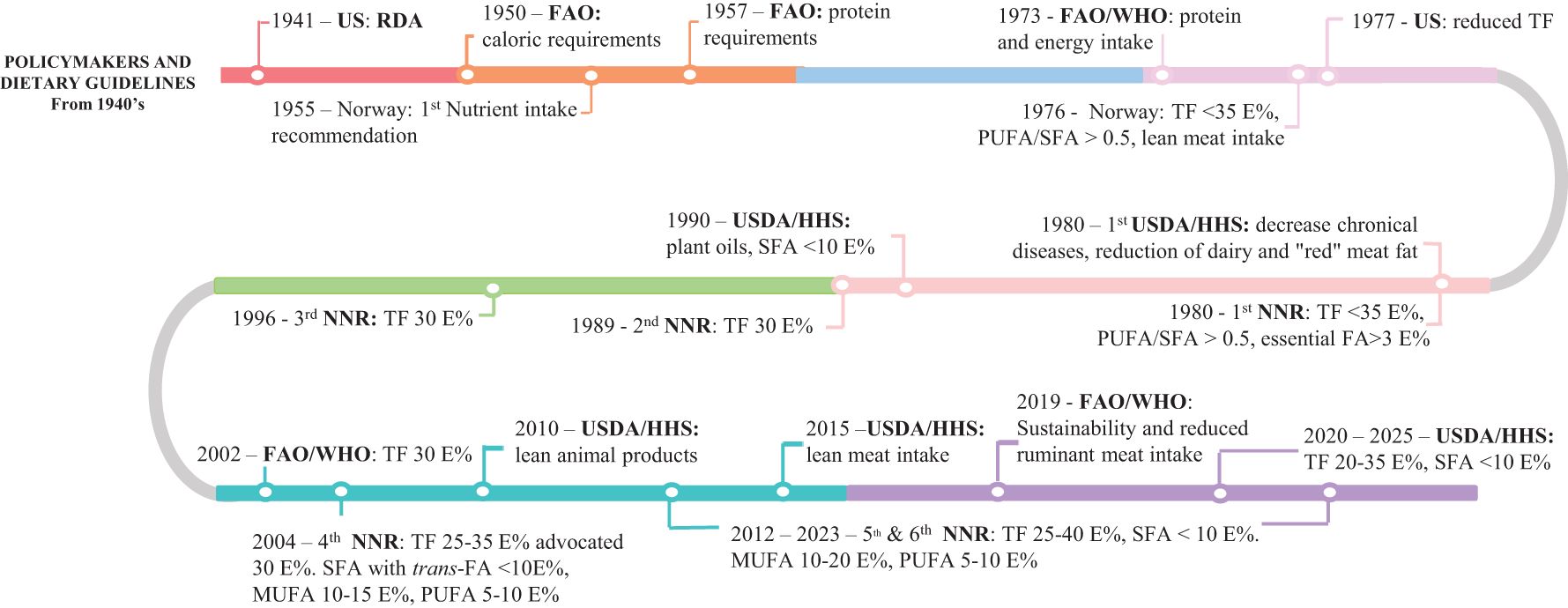
Figure 2. Overview of the development of human dietary guidelines at the global (WHO/FAO) and national (USA and Norway) levels with a focus on macronutrients (protein, fat, FA, and cholesterol). USDA/HHS, Dietary guidelines for Americans; NNR, Nordic Nutrient Recommendations; RDA, Recommended Dietary Allowances; TF, Total fat content; FA, Fatty acids; SFA, Saturated fatty acids; MUFA, Monounsaturated fatty acids; PUFA, Polyunsaturated fatty acids; E%, Energy percentage, i.e., percentage of total energy intake.
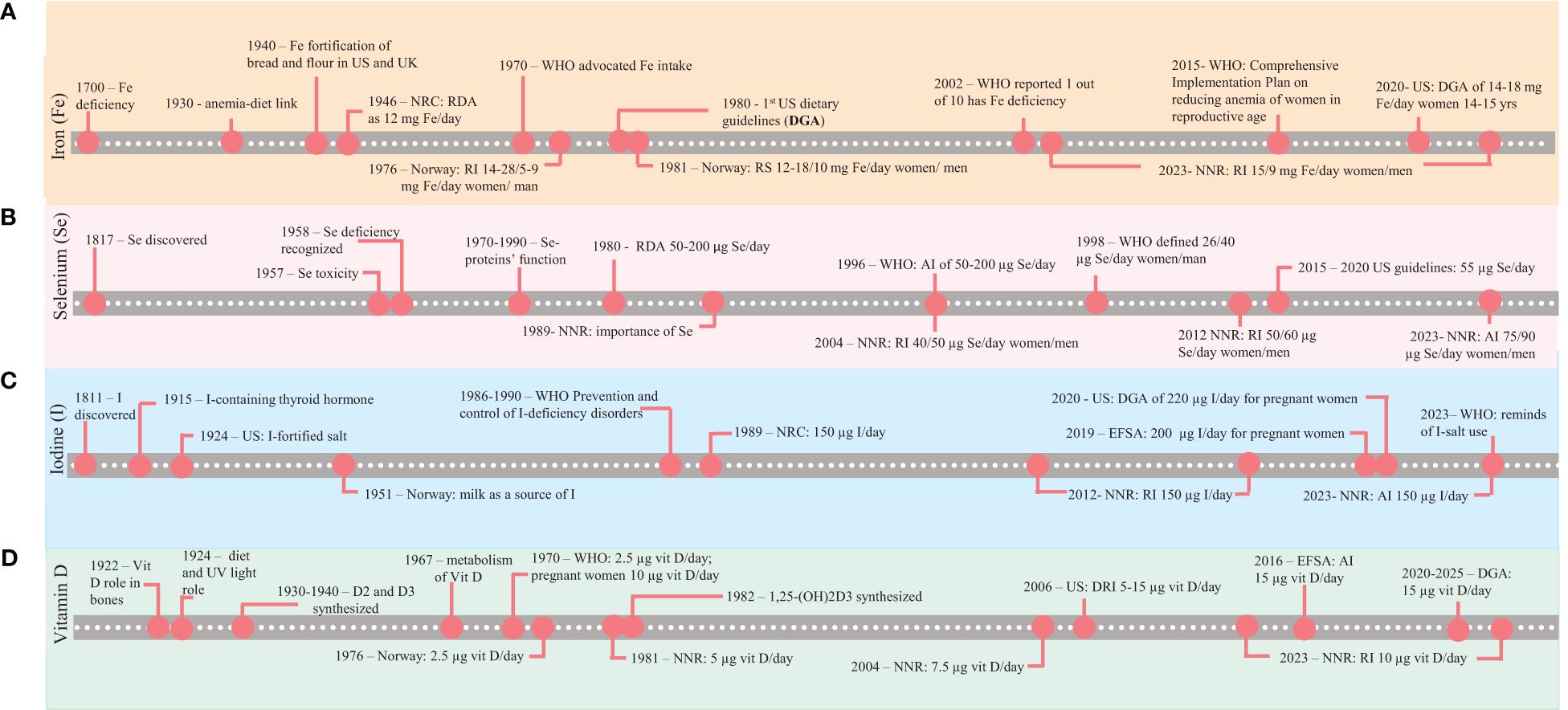
Figure 3. Timeline of the discovery and guidelines for four selected micronutrients, (A) Fe, (B) Se, (C) I, and (D) vitamin D, in the human diet (1800–2023) at global (WHO/FAO) and national (USA and Norway) levels. NRC, National Research Council; NNR, Nordic Nutrient Recommendations; RDA, Recommended Dietary Allowances; RI, recommended intake; RS, Recommended supply; AI, adequate intake; DRI, Dietary reference intake.
The following section describes the guidelines for animal fats (TF and FA) and the fate of the four selected micronutrients playing essential roles in meat nutrition in the past 60 years. The term “meat” does not encompass processed meat products unless stated otherwise.
3.1 Animal fats
3.1.1 Total fat
A political report published in the USA in 1977 (McGovern, 1977) recommended reducing the intake of TF and cholesterol (Figure 2). Subsequent guidelines (USDA/HHS, 1980) have reflected the requirement for maintaining energy balance (preventing obesity) and reducing the intake of refined sugar, TF, cholesterol, saturated fatty acids (SFAs), added salt, and animal fat. The FAO/WHO (2002) recommends limiting the maximum intake of TF to 30 E%. The FAO (2010) has reported that the total E% is not an issue at present; however, the quality of fat must be maintained.
The first Nordic recommendations (NNR issues of 1980, 1989, and 1996) sought to reduce the intake of TF and increase the intake of carbohydrates and fiber (Figure 2). In addition, the consumption of lean meat was also supported. The subsequent recommendations proposed by the NNR recommended the intake of TF first to be 30 E% and then 40 E%. The UL was possibly set to 40 E% as starches per se are not healthy (WCRF, 2023) and should not replace fat or protein to a great extent.
3.1.2 Fat categories and fatty acid composition
The USDA/HHS (1990) recommends SFAs of <10 E% (Figure 2). These guidelines also recommend the intake of dietary plant oils and limiting the intake of land-based animal fats. The USDA/HHS (2005) has published specifications regarding the intake of essential FAs. The DGA 2020 recommended limiting the intake of linoleic acid (LA) and α-linolenic acid ALA at 12(17) g/100 g and 1.1(1.6) g/100 g for women and men (age group 19–50), respectively. The SFA recommendations have not changed over time (USDA/HHS, 2020; Astrup et al., 2020).
A report on diet and chronic diseases published by the FAO/WHO (2002) identified myristic acid (C14:0) and palmitic acid (C16:0) as convincing/probable factors promoting CVD. However, ALA, LA, and oleic acid have exhibited opposite trends. The FAO/WHO published a report on sustainable healthy diets (FAO/WHO, 2019) and expressed that monounsaturated fatty acids (MUFA) are an important component of unsaturated fat.
It is recommended in Norway that the polyunsaturated fatty acid (PUFA):SFA ratio be maintained at >0.5 (Norwegian Parliament, 1975). The NNR reports (NNR, 1989, 1996) focus on the quality of fat. The subsequent reports (NNR, 2004, 2012, 2023) have recommended ranges for SFAs, MUFAs, and PUFAs for the population aged ≥2 years. The sum of MUFAs and PUFAs should constitute at least two-thirds of the total FA intake in the diet. The NNR (2004) have provided detailed recommendations for SFAs <10 E%, trans-FA <1 E%, and with 1 E% from n-3 PUFA. Specific recommendations for LA and ALA have been set as >3 E% and >0.5 E% from ALA (NNR, 2004). The NNR reports published from 2012 to 2023 have made recommendations for n-3 PUFA, LA, and ALA. The recommendations for the intake of PUFAs is maintaining an n-6:n-3 ratio of 5; however, the evidence is insufficient (FAO, 2010; NNR, 2023).
The increase in the number of constraints with time, despite no success in reaching SFAs <10 E% in Norway (Directory of Health, 2023), the USA (Biing-Hwan et al., 2023) and the EU countries (EC, 2023), must be noted.
3.2 Lean meat
Figure 2 presents the transition from supporting the consumption of lean meat to specific restrictions (FAO/WHO, 2019; NNR, 2023) due to the reported negative effects of “red meat” on human health (WCRF, 2024) and the environment. Notably, proper local calculations of the environmental effects are often missing. Nevertheless, “white meat” is now a replacement in many parts of the world, and global poultry meat production has increased from <10 Mt in the early 1960s to almost 150 Mt and 40% of the total meat produced in 2023 (FAO, 2023). Limited documentation exists regarding the significant differences in the protein quality ranking of different meats.
3.3 The development of guidelines for some micronutrients needed in the human diet
Figure 3 presents the development of guidelines for micronutrients by the WHO, the USA, and the Nordic region. Section 4 summarizes human deficiencies in detail.
Table 2 highlights the primary deficiency symptoms and the dietary importance of micronutrients across various food categories.
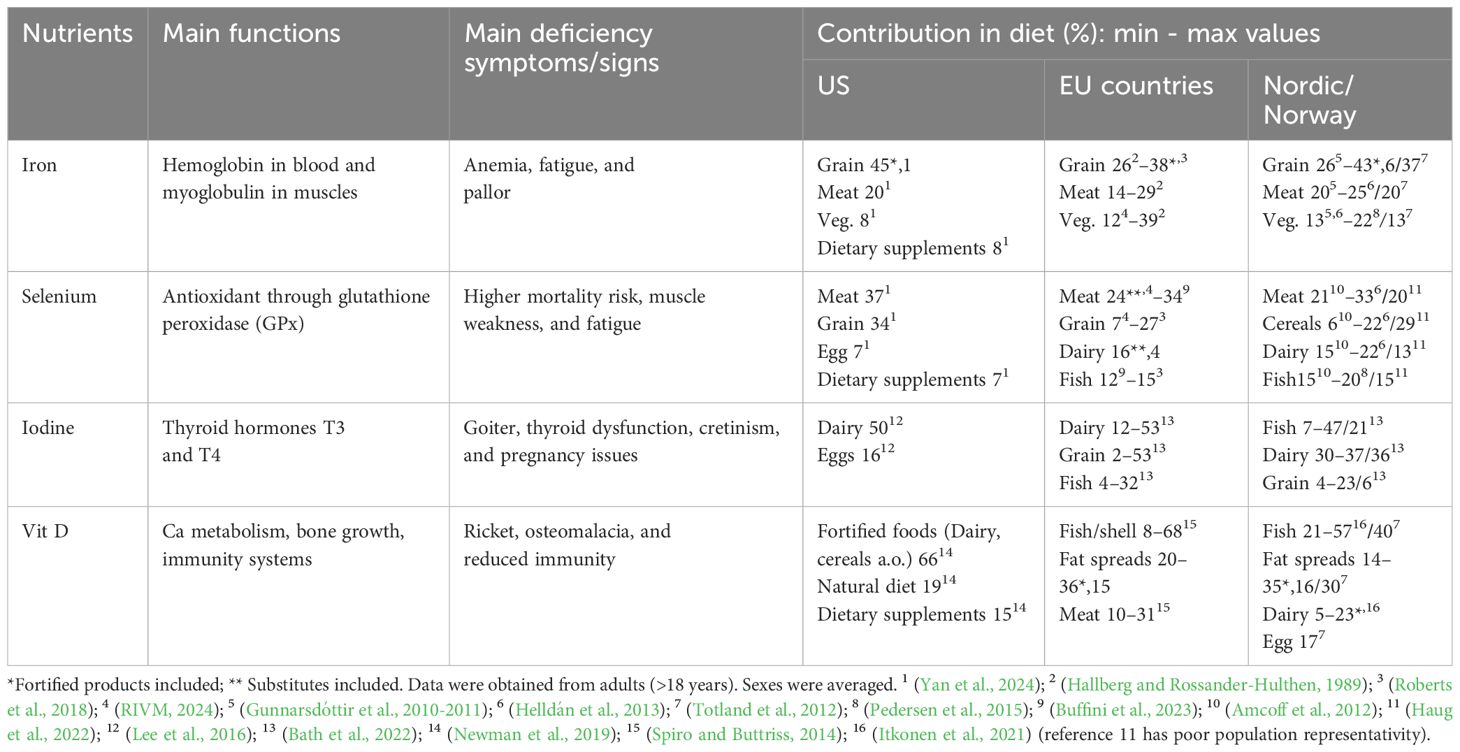
Table 2. Contribution (%) of various food categories in the human diet to selected macronutrients and micronutrients in which animal food (meat and milk) could be relevant.
3.3.1 Iron
Iron deficiency was first recognized as a health issue in the 17th century (Guggenheim, 1995) (Figure 3A). The presence of Fe in blood was recognized in 1825; however, the role of Fe in the diet was then still a speculation (Poskitt, 2003). Table 2 outlines the primary functions of Fe in humans. The FAO/WHO (1970) recommended the intake of iron according to the percentage of animal foods in the diet. The PRI for menstruating women was set as 14, 19, and 28 mg Fe/day, depending on the intake of animal-based food. The recommendations for women (15–50 years) have since been set to approximately 15 mg Fe/day (USDA/HHS, 2020; NNR, 2023). Fe deficiency in vulnerable groups persists as a challenge, as indicated by the WHO (WHO, 2002, 2009; McGuire, 2015). Ferrari et al. (2011) reported Fe sufficiency (serum ferritin levels of >15 µg/L) in 71.7% of girls aged 12.5–14.99 years in 10 European cities. This finding suggests that Fe deficiency (see Section 4) may be detected in subsamples of the population in the coming decades even in developed countries, possibly amplified by gastrointestinal disorders (Pasricha and Moir-Meyer, 2023) or nutrient dilution (Marles, 2017). The UL for human adults has been set to 45 (NASEM, 2006) and 40 mg Fe/day as a “safe level of intake” (a more conservative UL) (EFSA, 2024a).
The FAO/WHO recognized that heme Fe (HeFe) has higher bioavailability than inorganic Fe in 1970 (FAO/WHO, 1970). Meat contains factors that increase human Fe absorption from plant-based foods (Consalez et al., 2022; Nair and Augustine, 2018; Fairweather-Tait, 2023). However, this factor may be less noticeable when considered for all types of diets (Consalez et al., 2022).
3.3.2 Selenium
Figure 3B presents the Se requirements in human nutrition. Table 2 presents the main symptoms of Se deficiency and its function. The WHO emphasized the lack of knowledge about Se requirements in the human diet (WHO, 1996). The initial recommendations for Se intake were imprecise (NRC, 1989; WHO, 1996; FAO/WHO, 2004).
Selenium intake is suboptimal in many locations in Europe (Stoffaneller and Morse, 2015). A 50% increase in the intake was recommended from 2012 to 2023 in the Nordic region (NNR, 1996, 2012, 2023). The UL for Se intake is 255 µg Se/day (EFSA, 2023). Approximately 70–80% of Se is absorbed depending on the food item (Alexander and Olsen, 2023). C-bound Se (organic Se) is absorbed better than inorganic Se.
3.3.3 Iodine
Fortification of salt with I was commenced in 1924 (Figure 3C) to tackle I deficiency (Andersson et al., 2012), and this is still the main strategy recommended by the WHO (2014). Although the WHO aimed to eliminate I deficiency by the year 2000 (WHO, 1986a, 1990), two billion individuals (WHO, 2007), including citizens in several European countries (Ittermann et al., 2020), are still at risk of developing I deficiency.
The recommended AI of I has been set to 150 and 200 µg/day in adults and pregnant women, respectively (NNR, 2023). The AI recommended by the NNRs is consistent with those recommended in the EU and USA (USDA/HHS, 2020), whereas the NNRs set forth in 2023 recommend a slightly lower AI for pregnant women (200 µg I/day vs. 220 µg I/day). The UL for adults is set at 600 µg I/day (NNR, 2023). Inorganic I (iodides, I-), like table salt, is easily absorbed from the diet (Jahreis et al., 2001) and is the dominant form of I in milk (80–93% of total iodine) (van der Reijden et al., 2019).
3.3.4 Vitamin D
A link between sunlight (UV) and health was established between 1890 and 1930 (Figure 3D). Diet was then identified to have a similar function as the sun for the human body, and the vitamers D2 and D3 were identified as relevant (Jones, 2022). The bioactivity of the different vitamers D2, D3, 25-(OH)D3, and 1,25-(OH)2D3, are as follows: 1,25-(OH)2D3 > 25-(OH)D3 > D3 = D2 (Blunt et al., 1968). The relative bioactivity of 25-(OH)D3 compared with those of D3 and D2 has been debated (Tripkovic et al., 2017; Cashman et al., 2012; Durrant et al., 2022).
Figure 3D presents the gradual increase in recommendations for vitamin D intake (FAO/WHO, 1970; EFSA, 2016; USDA/HHS, 2020; NNR, 2023) and the UL for vitamin D is set to 100 µg/day. EFSA (2016) has set the suitable blood target value as 50 nmol/L for all population groups.
3.3.5 Balance in micronutrients
Nutritional guidelines recommend assessing dietary patterns (USDA/HHS, 2020) as an additional measure to determine the intake of single nutrients. However, these guidelines should encompass micronutrient ratios (Kelly et al., 2018), provided that the ratio of these micronutrients is significant. Two ratios (vitamin D/Fe and I/Se) were deemed important in this article. Most observational studies have confirmed the presence of a positive relationship between Fe and vitamin D intake (Azizi-Soleiman et al., 2016). An imbalance between the intakes of I and Se may lead to cancer of the thyroid and exocrine organs and glands, e.g. stomach, breast, and prostate (Dijck-Brouwer et al., 2022). A high I:Se ratio indicated a potential harmful effect in rats (Hotz et al., 1997).
4 Changes in carcass, meat, and milk composition: maintaining food healthiness and sustainability through breeding and feeding
4.1 Case 1 – Improvement in the quality and quantity of fats from monogastric animals
4.1.1 Total fat
Dietary animal fats have garnered significant attention. Pork fat is the most ingested type of animal fat in some countries (World Population Review, 2024); thus, clarifying its health benefits is important. The reduction in backfat thickness in pigs has been a breeding goal in Norway since the mid-1950s (Figure 4). This goal aims to transform pigs into lean and feed-efficient animals. The dramatic change in backfat thickness can be attributed to the demand for increased production efficiency. However, this is consistent with the nutritional debate that emerged later regarding the requirement for more protein and less animal fat. Breeding techniques have increased the amount of meat from approximately 40% to 60% of carcass weight (Figure 4; Supplementary Table 1), with the backfat thickness declining from 34 mm to 5 mm (Figure 4B). The reduction in backfat thickness, on average, was approximately 13 kg of adipose tissue per slaughtered pig. The TF content was estimated based on the supplied pig carcasses (devoid of head and front paws) and the Norwegian human population in 1953 (3.429 million individuals, 4.82 kg of pork fat per capita/year consumed) and 2020 (5.379 million individuals, 4.25 kg pork fat per capita per year, wholesale numbers). Notably, Norway is not involved in the major export or import of pork meat (<0.02% import in 2021). The TF content in the muscles of chickens may have declined over time due to extensive breeding (Zotte et al., 2019).
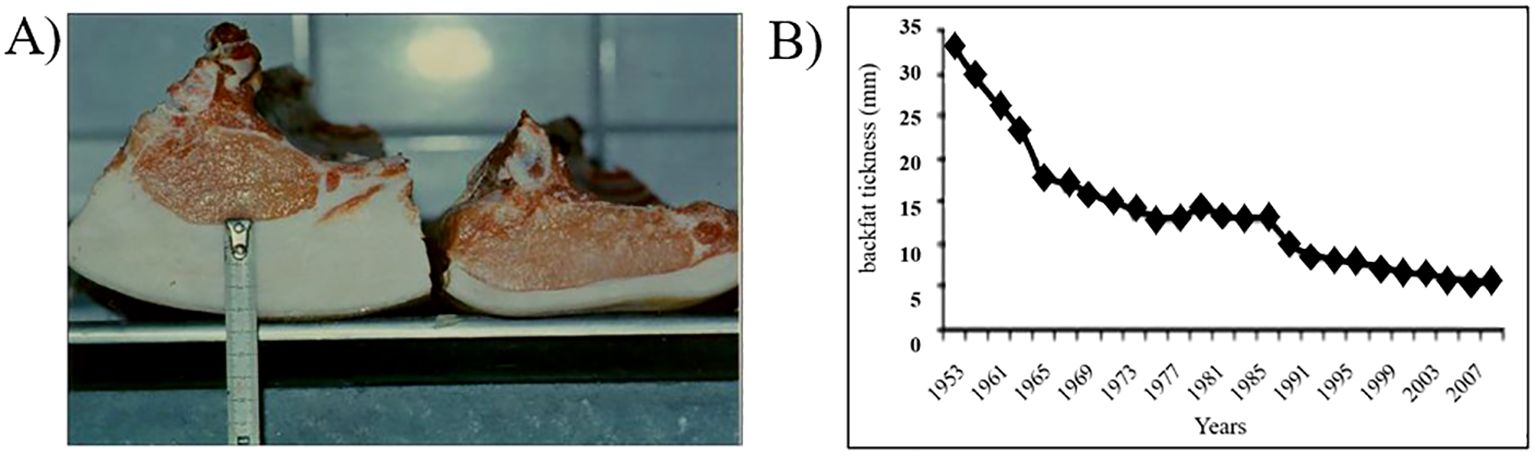
Figure 4. The impact of Norwegian pig breeding programs on carcass traits. (A) Illustrations of backfat thickness between 1953 (left) and 1980 (right). Source: Originally published in “Studies on a two trait selection experiment in pigs. III. Correlated responses in daily feed intake, feed conversion and carcass traits”, O. Vangen, Acta Agriculturae Scandinavica B, 1980, 29, 337–345 (Vangen, 1980), reprinted by permission of the publisher (Taylor & Francis Ltd, http://www.tandfonline.com). (B) The decrease in backfat thickness. Source: Originally published in Genetic analyses of meat, fat and carcass quality traits measured by rapid methods, E. Gjerlaug-Enger, Philosophiae Doctor (PhD) Thesis No. 13, Norwegian University of Life Sciences (NMBU), Ås, Norway (Gjerlaug-Enger, 2011), reprinted by permission of the author.
4.1.2 Fatty acid composition of pork
The changes in the fat composition of pork observed in previous studies can be attributed to genetic factors or animal feed. In addition to the reduction in backfat thickness in pigs facilitated by selective breeding, important changes have also been observed in the FA composition. The SFA content in pork fat has declined, whereas the PUFA content has increased (Table 3), making pork fat a potentially important source of PUFA for humans. The intake of SFAs via pork has decreased by 33% per capita from 1960 to the present. In addition to the genetic changes, the FA composition of meat from monogastric animals, such as pigs, is strongly influenced by the dietary FA composition.
The pork fat ingested at present is consistent with the updated (NNR, 2023) recommendation for a minimum dietary intake of the MUFA+PUFA fraction (two-thirds of the TF content; Table 3). Various effective strategies can be applied to improve the composition of FA to increase the (long chain) n-3 PUFA content.
Studies investigating whether the changes in animal fat composition significantly affect human health are scarce. However, assessing the changes in fat from monogastric animals (chicken and pork) and the accompanying changes in human health over time is difficult due to the lack of time-correct samples. A comparison of the present composition of animal fat with assumed healthier oils (e.g., rapeseed and olive oils) in terms of health benefits using a mouse model (Figure 5) revealed that the health benefits of pork fat are closer to those of some oils than beef fat. This has led to questions regarding whether the grouping of pork in the “red meat” group based on fat quality is appropriate. Data from the human intervention (Liposcale® data, Figure 5) show that the intake of pork fat is more beneficial than the intake of beef fat. Thus, the ranking supports the mouse model experiment.
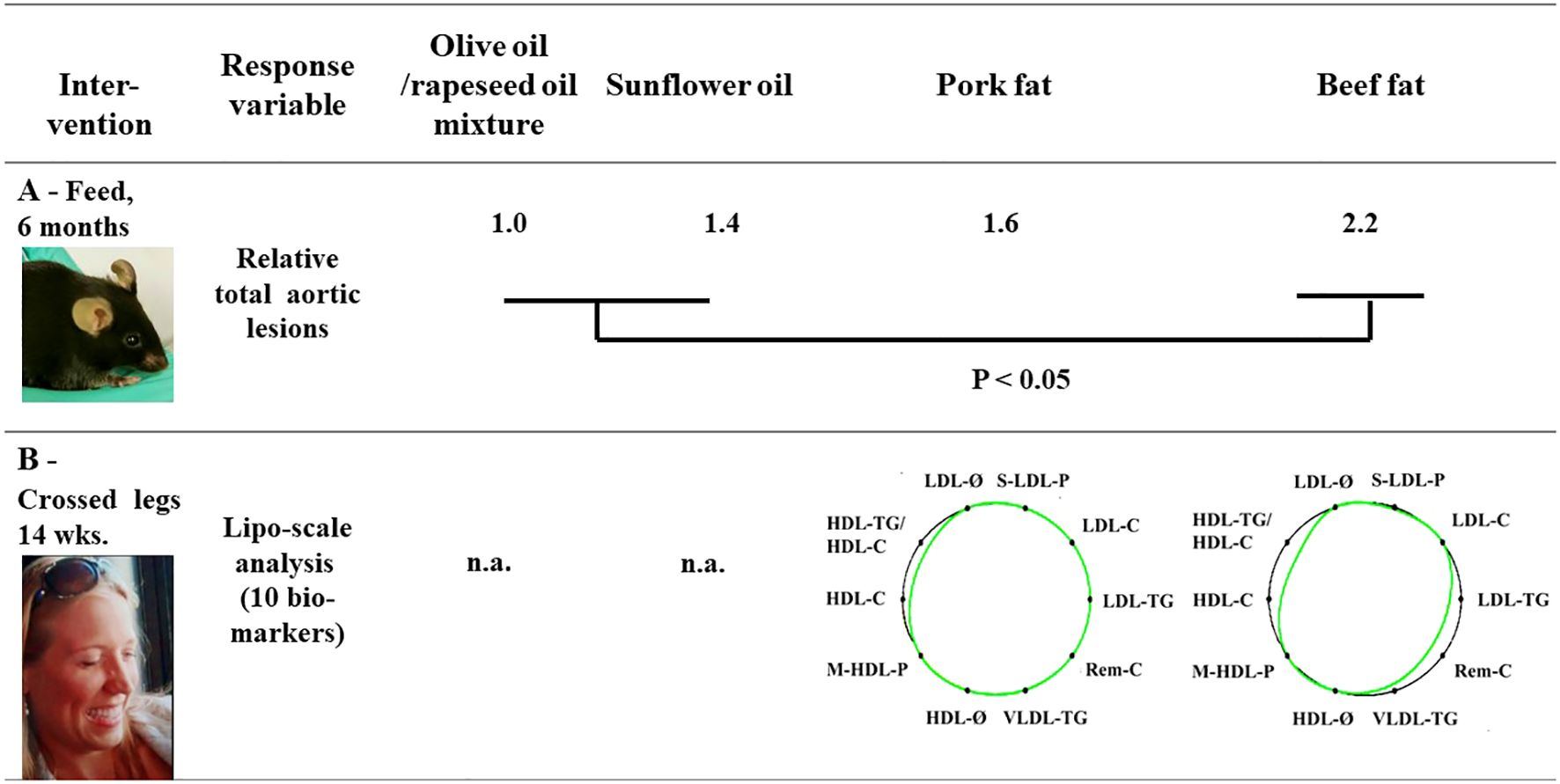
Figure 5. (A) Arthrosclerosis test results using Norwegian Landrace production fat tested for a hard endpoint (aortic lesions) in a mice model. Data adapted from (Morrison et al., 2023). Photo: Harald Carlsen. (B) The results of the Liposcale® test reflect the cardiovascular risk predictions (Mallol et al., 2015). Green lines indicate test diets with fats, whereas the black lines indicate reference lines for the general healthy population (Pinto et al., 2020). The degree of reduction in the area defined by the test lines inside the black line suggests an increased risk prediction. Data were vere adapted from Monfort-Pires et al. (2023). Photo: Anna Haug, used with permission.
4.1.3 Fatty acid composition of chicken
Chicken is an important source of unsaturated FA, such as n-6 FA, in countries wherein the intake of chicken is twice the intake of pork, and the intake of beef is limited. The SFA content in chicken fat is lower than that in pork fat. The n-6 fatty acid levels are significantly higher than the n-3 fatty acid levels in commercial chicken meat. The ratio of 9.5 (The Norwegian Food Safety Authority, 2016–2017a) is approximately two times higher than the ratio based on the recommended intakes of n-6 and n-3 fatty acids in the NNR (NNR, 2023). However, the n-6:n-3 ratio can be improved by implementing feeding programs with an additional focus on reducing the content of inflammatory arachidonic acid (AA).
Haug et al. (2012a) produced two types of chicken meats via feeding. The first type was obtained by feeding the chicken a commercial feed-like concentrate containing 4% soybean oil (SO). The second type was obtained by feeding the chicken a feed in which the SO was replaced with 2% rapeseed oil + 2% linseed oil (RLO). The content of n-3 PUFAs in the RLO feed is 3.4 times higher. The n-6:n-3 ratio in the RLO feed is 1.75, compared with the n-6:n-3 ratio of 9.8 in the SO feed. The n-3 PUFA content of the breast meat of chickens fed RLO was 2.7 times higher than that of the SO meat, and the n-6:n-3 ratios were 1.57 and 6.5 in the RLO- and SO-fed chickens, respectively (Haug et al., 2012a). A human intervention involving the ingestion of approximately 160 g of the two chicken meats daily for 4 weeks was carried out (Haug et al., 2012a). Consumption of RLO chicken resulted in an increase in the content of EPA+DHA in the blood of participants who had a low EPA+DHA% in serum phospholipids (<4.6%) before the intervention. Thus, a simple change in chicken feed can have beneficial effects on the amount of EPA (increased) and AA: EPA ratio (reduced) in the serum phospholipids in humans.
Improvement in the FA composition of meat can be achieved by modifying the diet of these animals; however, the sustainability demand at present is for the inclusion of locally produced oils (including neighboring countries) in feed. The direct use of oils in the human food chain may present challenges. However, the focus on formulating feed for farmed animals that does not involve using fields suitable for farming plant crops for humans has increased in recent years.
4.2 Case 2 – The effect of genetics and production systems on iron content in meat
4.2.1 The reduction of Fe content in muscle through efficient breeding and reduced physical activity
The Fe content in the meat acquired from all production animals has decreased over the previous 80–100 years. Selective breeding has reduced the requirement for Fe and myoglobin (Mb) in muscle cells (see Sections 2 and 3). Extensive breeding may diminish the “normal” function of mitochondria (Zhang et al., 2023; Suliga et al., 2022). Reduced physical activity (increased use of indoor housing) can reduce the requirement for Mb as an oxygen reservoir in cells. Newcom et al. (2004) reported that approximately one-third of the variation in pork muscle Mb can be attributed to different genetic factors. This finding indicates the genetic potential for variation. Apple et al. (2007) tested 50, 100, or 150 ppm of Fe from Availa-Fe (Fe with amino acid complex) and revealed the absence of any significant differences in the Japanese color score, HeFe level, or total He level in slaughtered animals. Thus, the Fe content in pig meat cannot be easily altered by feeding. Similar results have been reported in chickens. Although outdoor production is expected to yield redder meat, it is not always found (Olsson and Pickova, 2005).
The Fe content in indigenous chickens in Kenya (Chepkemoi et al., 2017) is 2.16 mg Fe/100 g of whole chickens. Red Jungle fowl thighs contain approximately 2.6 mg Fe/100 g meat (Callforlife, 2024). The ancient Polverari chicken (known since 1400–1500) contains 1.62 mg HeFe/100 g thigh (Pellattiero et al., 2020), indicating that the TFe is at least 2.1 mg/100 g (RIVM, 2023a). Whole chicken and thighs may contain 0.8 mg Fe/100 g at present (RIVM, 2023b). In Norway (The Norwegian Food Safety Authority, 2023a; 2016–2017b), deskinned raw chicken and thighs contain 0.5 mg Fe/100 g and 0.7 mg Fe/100 g, respectively. Thus, although it is difficult to obtain accurate Fe data to facilitate the comparison of ancient and present chicken breeds, the Fe content in chicken meat has decreased significantly through breeding, presumably by 70–75%.
The longissimus thoracis et lumborum (LTL) of pigs contain 0.8 mg Fe/100 g (The Norwegian Food Safety Authority, 2023b). Similarly, pork fillet contains 0.4 mg Fe/100 g (RIVM, 2023c). Minced pork meat, which represents a larger part of the carcass, contains 0.8 mg Fe/100 g (The Norwegian Food Safety Authority, 2023c) to 0.9 mg Fe/100 g (RIVM, 2023d). The musculus serratus anterior of wild boar, a presumed oxidative muscle, contains 4.0–5.6 mg Fe/100 g (Skobrák et al., 2011). Compared with the commercially available pork meat, an 18–41% reduction in Fe content was reported by the authors. A Polish study reported that Fe content in domestic pigs was 60% lower than that in wild boars (Babicz and Kasprzyk, 2019).
The Fe content in beef has also exhibited a declining trend. Sambugaro et al. (2023) reported that the Fe content in the LTLs of endangered cattle breeds and dominant well-bred Norwegian Red Cattle was 2.73 mg Fe/100 g and 2.09 mg Fe/100 g, respectively, indicating a reduction of approximately 23%. Fairweather-Tait (2023) provided a more detailed description of the HeFe and HeFe/TFe content in meat. Notably, a lesser decline in the Fe content in the meat was observed in animals subjected to lesser breeding.
4.2.2 Breeding affects heme iron reduction in the muscle
Few food composition databases provide the HeFe data for muscle-based foods. Thus, assessing the changes in the HeFe: TFe ratio is difficult. Pellattiero et al. (2020) revealed that compared with those in ancient slow-breeding animals, present-day commercially available chickens had 70.4% and 69.7% lesser HeFe content in the breast and thigh muscles, respectively. The HeFe content in commercially available chickens is 33% of the TFe (RIVM, 2023e).
Changes in the Fe content of pork meat sourced from wild boars have been observed. Kasprzyk et al. (2019) reported the presence of Mb in wild boars of different weights (45–90 kg), with 1.64–4.5 mg HeFe/100 g being detected in the LTL. However, the data reported for TFe in wild boars vary substantially, thereby hindering the precise calculation of the HeFe : TFe ratio. Sampels et al. (2023) reported the color variables of whiteness and redness that are expected to correlate with Mb content in the M. triceps brachii muscle. Whiteness increased from 31.3 in wild boar meat to 47.2 in commercially available pig meat, whereas redness decreased from 15.4 to 2.4 (Minolta data). Whiteness values >60 in commercially available pigs indicate a muscle defect. The HeFe : TFe ratio in pork mince was reported as 0.55 (RIVM, 2023d). Cross et al. (2012) reported that the HeFe level was <67% of the TFe level in the chuck part of beef in the USA. The HeFe level in beef mince accounts for approximately 80% of the TFe, according to RIVM (2023f), indicating a different breeding strategy than that used for pork and chicken. Thus, as shown in Section 4.2.1, the decline in heme content over time in beef is expected to be lower than that in chicken or pork.
4.2.3 A human intervention elucidating the effect of the decline in iron content through animal breeding and feeding dilution
An insufficient amount of absorbable Fe remains the primary cause of Fe deficiency in women in most countries (Pasricha and Moir-Meyer, 2023). The average daily Fe intake in the Nordic and Baltic Countries ranges from 9.4–14.5 mg Fe/day according to the NNR (2023), with the lowest intake being observed among women in Iceland and the highest intake being observed among men in Latvia (Lemming and Pitsi, 2022). The average daily Fe intake of women in the Nordic and Baltic regions was below the recommended intake in all countries (Lemming and Pitsi, 2022).
A relatively recent peer-reviewed publication from the USA (Sun and Weaver, 2021) measured the serum Fe levels, hemoglobin levels, red cell distribution width and mean size, Fe intake, and diseases contracted from 1999 to 2018. A significant decline in Fe intake and hemoglobin levels was observed in this study. Notably, the Fe content in most food products (62.2%), including meat, fruits, and vegetables, was less in 2018 than that in 1999. The Fe content in meat declined to 80–86% of the value in 1999 during the period from 1999–2015, whereas the Fe content in turkey meat declined to 61% of the value in 1999 (Datasource: USDA Nutrient Database). The decline in dietary Fe intake observed in the USA could be related to the decline in the Fe content of food products due to more intensive agriculture and a shift in dietary patterns (Sun and Weaver, 2021). The findings of the study by Yan et al. (2024) support this conclusion. A recent commentary paper questioned the reliability of the estimated decline in Fe intake in the USA; however, this study acknowledged the presence of groups vulnerable to Fe deficiency (Engle-Stone et al., 2022). Fairweather-Tait (2023) summarized human interventions related to HeFe and TFe absorption and indicated that the absorption efficiency also depends on the degree of deficiency. An increase in Fe intake through the consumption of meat has been reported in Norway (up by 3% from 1959 to 2022) (See Supplementary Material).
4.3 Case 3 – The selenium content in meat is affected by breeding and feeding
Approximately one billion individuals are affected by Se deficiency globally (Jones et al., 2017); however, the symptoms of Se deficiency in humans remain unclear (Table 2). Studies from the USA and Europe (Alexander and Olsen, 2023) have defined Se deficiency as plasma/serum Se values <100 µg/L. The intake of Se was below the AI recommended for the Nordic region (NNR, 2023) (Figure 3) in >80% of the participants in an intervention study (Haug et al., 2022). The average daily intake (ADI) of Se in the Nordic and Baltic countries ranges from 20–88 µg Se/day, with the lowest intake being observed among women in Lithuania and the highest intake being observed among men in Finland (Lemming and Pitsi, 2022). Thus, large parts of the Nordic and Baltic populations are below the AI of 75 and 90 µg Se/day recommended for women and men, respectively (NNR, 2023). The dietary sources of Se may change over time (nutrient dilution) and vary across different years depending on the import pattern of food. A Polish study revealed that cereals provide less amounts of Se over time (Zbigniew, 2002). Thus, the consumption of meat products may be more effective in combating Se deficiency than the consumption of cereals (Zbigniew, 2002). The results shown in Table 2 confirm these findings.
4.3.1 Factors affecting the selenium content in meat during recent decades
The Se concentration in the soil is the key determinant of Se concentration in meat. For instance, the Se content in meat sourced from the USA may be higher than that in meat sourced from a European country with suboptimal levels of Se in the soil. The study conducted by Zhang et al. (1993) revealed that ground beef, pork, and chicken sourced from Texas contained 19.0 µg Se/100 g, 27.0 µg Se/100 g, and 13.0 µg Se/100 g, respectively. In contrast, beef, pork, and chicken sourced from France contained 6.0 µg Se/100 g, 8.0 µg Se/100 g, and 5.0 µg Se/100 g, respectively.
According to the Cicual database in France, raw chicken meat contains 12.4 µg Se/100 g (Cicual, 2020). The Se content in beef and pork meat in the USA (USDA/FDC, 2003) is higher than that in some European countries (e.g., Norway), whereas that of other countries is closer to the USA (e.g., Finland). The implementation of a biofortification program using Se (as selenate) as a fertilizer in Finland since 1985 has led to an increase in the Se content in food crops, livestock, and the human population, with the Se levels in human serum doubling over the subsequent 5-year period (Hartikainen, 2005). However, providing a general conclusion regarding the temporal changes in the Se content of meat is difficult. Selective breeding to decrease the number of oxidative muscle cells may have reduced the requirement for Se (Wesolowski et al., 2022) in the muscle. Feed supplementation increases the Se content in the muscles; however, the magnitude achieved via recommended feeding and that achieved via breeding remains unclear.
4.3.2 Selenium content in meat assessed in a human intervention
An intervention study revealed that the intake of 55, 135, or 215 µg Se/day via Se-enriched meat significantly increased the plasma Se levels (van der Torre et al., 1991). Haug et al. (2018; 2022) reported a human intervention wherein 0.5 mg yeast-Se + 0.2 mg Selenite Se/kg of feed was fed to Norwegian Red bulls (control group was fed 0.2 mg Selenite Se/kg feed only). The raw meat (LTL) sourced from the control and experimental groups contained 11.7 and 15.2 µg Se/100 g, respectively. Commercially available LTL in Norway contains, on average, 7.8 µg Se/100 g (Egelandsdal et al., 2020). Biofortification of beef with Se ensures that meat is a source of Se, even in accordance with the updated NNR (2023) recommendations. A high amount of meat was consumed by young women for a short duration in a human intervention study (Haug et al., 2022). This study revealed that the consumption of Se-fortified beef increased the serum Se levels in young women that had less than 85 µg Se/L serum. Se uptake was surprisingly efficient, consistent with the WHO recommendations (WHO 1986b). Thus, muscle is an important organ for the storage of Se (50% of the Se content in the body), and optimizing the Se content in meat to meet specific consumer needs is possible (Oster et al., 1988).
Chicken feed supplemented with Se-rich yeast (0.5 and 0.84 mg Se/kg feed) increased the Se content from 28 to 39 µg Se/100 g in the chicken thigh muscle (Haug et al., 2007a). The higher concentration is similar to the Se content in wild fish (Haug et al., 2007b). In addition, the Se content in pork can be increased by adding organic Se to the feed (Gjerlaug-Enger et al., 2015), but an increase in the plasma Se levels in a human intervention with Se-enriched pork meat yielded unclear results (Bugel et al., 2004).
4.4 Case 4 – Iodine in milk and meat seen through the loop of ruminant diets
Iodine is commonly added to dairy feed at 3–4-fold higher levels than the requirement of the animal in many countries, including Norway, to increase the I content in milk. Dairy products are a major source of I in Mediterranean and Western diets (Table 2; Niero et al., 2023). However, unlike milk, I is not transferred efficiently to the muscles. Consequently, the I content in animal feed for dairy and meat production should vary, as indicated by Meyer et al. (2008). The average daily intake of I in the Nordic and the Baltic countries ranges from 25–268 µg/day, with the lowest intake being observed in women in Lithuania and the highest intake being observed in men in Denmark (Lemming and Pitsi, 2022). The most recent update from the Iodine Global Network (IGN) lists Norway among the countries with insufficient I intake among school-age children (SAC) (IGN, 2022). This is supported by studies showing I deficiency among young and pregnant women (Henjum et al., 2018; Nystrom et al., 2016) indicating the risk of low I intake in certain population groups of the population. The present Norwegian inclusion of 5 µg I/g in one type of table salt is having a minor dietary relevance.
4.4.1 Iodine content in milk is affected by feeding but varies over time and by season
The I content in the feed is the major factor influencing the I content in the milk. However, goitrogenic components, such as thiocyanates originating from glucosinolates in cruciferous plants (e.g., Brassica rapa), inhibit the transfer of I from the feed to milk. Iodine is transferred from the blood into cells via the sodium iodine symporter (NIS). Thiocyanate acts as a competitive inhibitor in NIS. Information regarding the influence of breeding on NIS or the genetic effect on the I content in milk is unknown; nevertheless, the I content in milk can be efficiently manipulated through feeding.
The variation in the I content in milk over the years is a source of concern. Table 4 summarizes the I content in the summer and winter milk in Norway between 1971 and 2013. The I content in milk decreased dramatically from 231 µg/kg in the winter of 2000 (Dahl et al., 2003) to 122 µg/kg in the winter of 2008 in Norway (Haug et al., 2012a) (Table 4). However, the rate of inclusion of I in the diet remained unchanged. The reduction in the I content in milk can be attributed to the increased inclusion of rapeseed products in the dairy cattle diets (Trøan, 2017). Rapeseed products contain glucosinolates, which result in a 30–70% reduction in the I content in milk even at extremely low levels (Trøan et al., 2015). Notably, the I content in milk increased between 2008 and 2012/13 despite the increased use of rapeseed products in feed (Table 4). This increase was achieved by increasing the dietary inclusion of I from 2 to 5 mg/kg in pelleted compound feed mixtures, corresponding to an increase from approximately 0.8 to 2.0 mg/kg DM in the total ration.
The I content in milk varies seasonally. This reduction in I content in milk during summer could be attributed to the lower concentrate intake, given that I is supplemented through concentrate. However, data from the Norwegian Dairy Recording System from 2011 to 2016 indicated a minor reduction in the intake of the concentrate during summer compared with that in winter. Iodine-containing teat dips are not used commonly (Whist et al., 2007), thus the reduction cannot be attributed to them. The presence of goitrogenic substances in pasture and fresh grass compared with silage could result in a reduction in the I content in milk. Although not well-documented, these substances suppress the transfer of I from feed to milk (Trøan, 2017). Nevertheless, these findings indicate that the I content in the feed should be elevated during summer.
4.4.2 Seaweed as a re-vitalized and iodine rich ingredient in ruminant diets
Seaweed has been used as animal feed in coastal areas for hundreds of years. It was mainly offered along with fish offal as an emergency feed during winter. Isaachsen and Al. (1917) conducted the first experiment on dried seaweed in dairy cow diets (Ringen, 1939) at our university. The palatability and energy value were low; thus, seaweed was not included in quantitative animal feed. Increased focus on sustainable feed systems has garnered attention to the use of seaweed in diet. In addition, seaweed has been identified as a promising mineral source in ruminant diets that positively affects the I content in milk and meat and enhances the physicochemical properties of meat (Grabež et al., 2022, 2023a; Makkar et al., 2016; Newton et al., 2021; Grabež et al., 2023b).
The incorporation of seaweeds in animal diets presents several possibilities and challenges. Compared with that in grazing animals (117 µg I/L milk), supplementation with Ascophyllum nodosum (113 g/day, corresponding to 0.6% in DM) resulted in a significant increase in the I content in milk (480 µg I/L milk) in cows (Antaya et al., 2019). Furthermore, an increase in the inclusion levels of A. nodosum (57 g/d, 113 g/d, and 170 g/d, corresponding to 0.3, 0.6, and 1.0% in DM, respectively) in the diets of dairy cow, had a direct effect on I content in milk (602 µg I/L milk, 1,014 µg I/L milk, and 1369 µg I/L milk) (Antaya et al., 2015). Comparable results were reported by Newton et al. (2023), who demonstrated high I content in milk (1,886 µg I/L milk) when the diet of Holstein cows was supplemented with 330 g (corresponding to 1.3% in DM) A. nodosum daily. Thus, seaweed can biofortify milk with I; however, it is also associated with a risk of the excessive addition of seaweed or the addition of seaweed with excessively high I content, rendering the I content in milk excessive, especially for infants. The transfer of I to milk may be counterbalanced by the simultaneous supplementation of the feed with rapeseed products. To the best of our knowledge, the potential effect in a combination of seaweed and goitrogenic feedstuffs remains underexplored.
A recent experiment conducted at our university farm comprised feeding dairy cows in peak lactation (on average 50 days in milk) a total mixed ration (TMR) comprising grass silage and concentrate (65:35 ratio on DM basis) or a TMR including 1% DM of S. latissima over a 4-week period. Feeding 1% seaweed (corresponding to 220–240 g/day) to dairy cows resulted in a higher feed intake and milk yield (Ueland, 2022). Furthermore, the I content was 6.6 times higher in milk. A potential risk of including high amounts of S. latissima in the feed of dairy animals is the transfer of As, Pb, Cd, and other heavy metals (Sharma et al., 2018). The findings of Ueland (2022) indicate that <1% of the total As content was transferred to milk.
In addition to the use of seaweed in dairy cow diets, the effect of including S. latissima in the diets of finishing lamb and bulls on the I content in meat was also examined (Grabež et al., 2022, 2023b). The inclusion of S. latissima at 2.5% and 5% on a DM basis (105.5 and 204.6 mg I/kg DM feed, respectively) compared with standard feed (5.4 mg I/kg DM feed) in lamb feed increased the I content in lamb meat by 26-fold and 37-fold, respectively, compared with conventionally indoor fed lambs (2.3 µg I/100 g of meat); an intake of 100 g would represent only 1.5% of the recommended AI. Seaweed supplementation could increase this level to 40–59% of the AI (Grabež et al., 2023a), making it another relevant source of I. In another study, 0.8% blanched S. latissima (on DM basis) was added to the feed of finishing bulls as the maximum inclusion level to comply with present law regarding I and As content in ruminant feed. The addition of blanched S. latissima induced a five-fold increase in the I content in meat. Thus, the use of seaweed as an I source in animal feed is feasible. Furthermore, it could be a promising strategy for producing I-rich meat and meat products. However, the level of its inclusion depends on the choice of seaweed species and the characterization of their mineral composition (Cabrita et al., 2016).
4.4.3 I level in milk assessed in human intervention studies
Few studies have investigated the bioavailability of I from various dietary sources. Organically bound I, such as iodiphores (complexed I) and protein-bound I, reduce the bioavailability of I. Nevertheless, Dydykin et al. (2019) reported that iodotyrosine and iodocasein are superior to iodized salt as external additives in meat products in the prevention of I deficiency in children. However, studies assessing the bioavailability of dietary I directly from meat and meat products are scarce.
4.5 Case 5 – The effect of genetics and production systems on the vitamin D content in meat
The vitamin D levels and intake of each species varies depending on the geographical location. Vitamin D deficiency in humans is often defined as blood values of <30 mmol/L for 25-(OH)D3, with a suitable target value of 50 nmol/L for all population groups (EFSA, 2016). This deficiency has been recorded in many countries (Palacios and Gonzalez, 2014). The average daily vitamin D intake in the Nordic and Baltic countries is <10 µg/day, except in Finland (Lemming and Pitsi, 2022). Muscle foods are presently considered vitamin-D-deficient food.
4.5.1 Increasing the low vitamin D content in the muscles via breeding, feed, and UVB light
Vitamin D influences mitochondrial function; thus, breeding glycolytic cells will reduce the requirement for vitamin D (Dzik and Kaczor, 2019). Burild et al. (2016) reported that the total vitamin D content in red pork muscles, independent of the type of vitamer used in feed (max 50 µg 25-(OH)D3 or D3/kg feed), was higher. This may reflect the higher intramuscular fat content in red muscles (Burild et al., 2016). Feeds supplemented with 25-(OH)D3 would be more favorable than those supplemented with D3 if the aim is to increase the total vitamin D content in lean meat (<20% fat). The total vitamin D content varies depending on breeding, feeding, and possibly body mass composition (degree of fatness); the latter has been observed in humans to influence serum 25-(OH)D levels (Kim and Cho, 2019).
A decline in the vitamin D content in meat was also observed when animals were raised indoors without any UV light facility and vitamin D3 content in the feed was low. Outdoor activity (sunlight exposure) may increase vitamin D levels more than feeding (Madson et al., 2012). UVB can increase the vitamin D3 content in pork and poultry (Rosbotham et al., 2022). Schutkowski et al. (2013) reported a vitamin D3 content of 0.5 µg D3/100 g in hen legs following the application of UVB illumination through four daily intervals. The 25-(OH)D3 level also increased, resulting in the effective vitamin D concentration increasing to 1.5 µg/100 g (Jakobsen, 2007) pending the bioactivity factor used for 25-(OH)D3. The use of UV light in pigs for 6 min/day for 10 weeks can result in a significant increase in the D3 level relative to the level recommended by the EFSA in animal feed (Neill et al., 2021, 2023). However, the application of UVB light requires further research (Rana and Campbell, 2021).
4.5.2 Content of 25-(OH)D3 in meat assessed in a human intervention
A higher 25-(OH)D3 content has been observed in beef, with levels of 0.16 µg/100 g being observed in meat (raised indoor) available commercially in the Norwegian market (minced beef) (Egelandsdal et al., 2020). Haug et al. (2018) reported an increase in the 25-(OH)D3 content in minced beef from 0.10 µg/100 g meat to 0.29 µg/100 g when the feed was supplemented with 0.025 g D3/kg feed and 0.1 g D3/kg feed, respectively. The increase in the 25-(OH)D3 content through the EFSA-recommended feed level may not seem large. However, the consumption of beef increased the blood 25-(OH)D3 levels of the intervention participants that had 25-(OH)D3 levels < 30 nmol/l (Haug et al., 2022). Nutrient-optimized beef can improve the blood levels of participants with low to normal 25-(OH)D3. However, the meat was examined in a high-dose-short-time intervention in the study (Haug et al., 2022). Further studies using lower doses and long-term administration must be conducted to verify whether vitamin D optimized meat improves the blood base values of individuals with 25-(OH)D3 levels <50 nmol/L.
4.6 Ratio of the selected micronutrients
The intake ratios for vitamin D/Fe (range 1.18–0.4) and I/Se (range 4.93–0.97) have been reported for the Nordic and Baltic regions (Lemming and Pitsi, 2022; See Supplementary Material, Figure 2 and Table 2 for vitamin D/Fe and I/Se). Although reference blood values are lacking for ratios, extreme ratios may pose an additional burden on the total intake of these micronutrients.
5 Critical views on human and animal nutritional guidelines in the context of improvements in milk and meat healthiness
Europe and the USA have produced enough food to ensure adequate caloric and macronutrient supply since the 1960s (Roser et al., 2023). Obesity (WHO, 2022) and hidden hunger have been included in the agenda. Nevertheless, higher-income consumers consuming fewer but higher-quality foods remains a prevailing trend (Hocquette et al., 2024; Liu et al., 2023).
5.1 The animal breeding and feeding timeline advanced faster than human nutrition guidelines: case fat
Fat limited in or devoid of essential FA and micronutrients is only an energy source. Therefore, the initial focus of the meat value chain is to decrease the ratio of fat to lean meat in animals to improve profitability and human health. However, whether further advancements in fat reduction in pigs, chickens, or turkeys should be continued is debated due to the physiological limitations of the animal. Furthermore, it is unclear whether the focus should be directed toward FA composition and animal health.
An increase in the MUFA and PUFA content is achievable in pork meat via feeding and breeding (Gjerlaug-Enger et al., 2011; van Son et al., 2017). Clear incitements for continuing the improvements have not been proposed, partly due to the challenges in the meat production value chain (e.g., lipid oxidation and soft fat), a lack of recognition from human dietary guideline generators, increased feed and raw food material costs, and constraints on animal feed.
5.2 Breeding achievements: case Fe
The drivers of the increased production of high-quality protein for humans via breeding have not focused on the Fe content in meat. In theory, the losses in Fe with breeding could be compensated for by a higher intake of meat (and organs) from beef in vulnerable groups. However, the consumption of beef is considered to increase the risk of developing chronic diseases (FAO, 2023), which limits intake. Increasing the intake of chicken and turkey may place meat in a category that it is no longer a good source of Fe (i.e., ≥15% of daily intake). Reversing the present breeding choice would increase production costs per kilogram of meat. Thus, increased feed supplementation may be required if high-yield grains/plants with lower mineral accumulation (“the dilution effect”) are used (Marles, 2017).
Few countries record HeFe data in their databases to an extent relevant for epidemiological modeling, bearing in mind that animal breeding is mostly local. Heme, carrying Fe, was in 2015 (WCRF, 2015), but not clearly in 2024 (WCRF, 2024), suggested to be causal for colon cancer. Aglago et al. (2023) reported a negative association between heme and colon cancer only in men.
The genetic changes in cattle, pork, and poultry vary, and the breeding goals for cattle may depend on dairy needs, e.g., if dual-purpose cattle are the focus. Thus, it is questionable that we use only two groups (“red or white” meat) and link these to chronic diseases nationwide. The levels of HeFe and SFAs, two assumed causal predictors implicated in lifestyle diseases, have clearly decreased in pork and chicken over the years. Our Supplementary data suggest that women favor meat with a reduced iron content (for example, chicken) that is less likely to prevent Fe deficiency even when their meat intake is increased.
5.3 Should we aim for nutrient richer or poorer meat: case Se and vitamin D
The Se and vitamin D content in meat may be negatively influenced by breeding choices. However, the existing literature on this topic is not detailed. The approach used here involves the supplementation of the feed with Se. Biofortification will be a necessary step in many parts of the world (Christophersen et al., 2013) to ensure adequate Se intake. This could yield animal products that are beneficial for human health and livestock (Haug et al., 2007b). Thus, focusing on the Se levels in meat and other animal products is important, but the Se content in the feed must be adjusted to obtain an optimal Se content in the products.
The EFSA reported that consumers are unlikely to exceed the UL for Se, except for regular users of supplements (pills) containing high doses of Se or regular users of high-Se foods, such as Brazil nuts (Turck et al., 2023). Supplementing the feed up to the UL of animals, along with intensive end feeding, can increase the Se or vitamin D content of meat.
Increasing the 25-(OH)D3 content beyond 0.5 µg 25-(OH)D3/100 g meat is challenging (Schmid and Walther, 2013); however, even this low level has a high utility in individuals with low vitamin D levels. The preceding sections have discussed the possibilities for increasing the vitamin D content in meat via feeding and technologies. However, further studies must be conducted on increasing the vitamin D levels in lean meat as many individuals have a low vitamin D/Fe ratio. Meat producers should aim to maximize and stabilize vitamin D levels. This is especially relevant in regions where animals are mostly reared indoors.
Nutrient-based criteria (such as proteins, MUFAs, vitamin B, and Zn) must be used by the meat industry to distinguish meat products from refined foods containing mostly fat or sugar and practically no essential nutrients, such as Se or vitamin D. Meat should supply nutrients missing from the diet at the highest level possible while being sustainable. Improving meat production beyond the present solutions is not necessarily costly (Haug et al., 2018). Selenium also accumulates in organs, such as the liver, that are not consumed commonly. The reintroduction of organs into the human food chain appears sustainable, given the global scarcity of Se.
5.4 Human iodine demand may necessitate dairy cattle
Milk and dairy products will continue to be the prime sources of I in the foreseeable future in Norway and many other countries (Bath et al., 2022). Increasing the I content in the feed remains the most efficient way of increasing I content in milk. NASEM (2021) recognizes this role in animal feeding and recommends increasing the levels of I in the ration (from 0.5 to 1.0 mg/kg DM) when using goitrogenic diets. Although the key is to ensure sufficient I content to facilitate thyroxine synthesis in cows, this increase indirectly preserves the I content in milk. Recommendations for achieving the optimal I content in milk remain to be established in Norway. However, considerable knowledge regarding milk production within the preset I range has been accumulated. Trøan et al. (2015) presented a model ensuring that the I content in milk was within certain limits. The I content in milk was related to the dietary I intake and the proportion of rapeseed products in the diet. Such models should be implemented to address the variations in the I content in milk due to the presence of goitrogenic components in the feed. This also plays an important role in facilitating sustainability, as rapeseed products contain sustainable, cost-efficient, and high-quality feed protein. The inclusion of I in the Norwegian compound feed for dairy cows has been set to 5 mg I/kg based on an agreement between the Norwegian feed and dairy industries aiming to declare I content in drinking milk. However, a UL of 5 mg I/kg may not be sufficient to stabilize the I content in milk for declaration. Alternatively, a combination of seaweed and goitrogenic feedstuffs can be used to reduce the level of I in milk.
The transfer of I to meat is not as efficient as that to milk; however, the amount of I in the feed beyond the present UL will increase the I content in meat to a level that makes meat a relevant source of I. In addition, the transfer of I from the feed to meat (and organs) differs among animal species. Implementation of this knowledge would necessitate the adjustment of present practices and regulations regarding the I level permitted in feed rations, depending on which animal to feed and the intended use.
6 Conclusion
More research must be conducted to address the present situation regarding meat. The animal-to-meat value chain must explore the use of their tools, with a stronger focus on meat healthiness. However, human nutrition guidelines are not updated at a sufficient rate in relation to the advances made in livestock research and production. Livestock research is particularly relevant in an era in which the demand for sustainability can accelerate the development of new feed and genetic solutions. Closer collaboration between the entire animal value chain, human nutritionists, and feed and food authorities will aid in addressing the complexity of healthiness and sustainability. The fat case used here would have been developed more efficiently and received more positive publicity through such a collaboration.
The following can be concluded from this article:
● There is an urgent need to terminate the concept of “red meat.” Redness is related to the content of myoglobin-containing heme, which can be measured and adjusted via animal breeding and production. The names of the animal species investigated must be used to accelerate the identification of components associated with an increased risk of colon cancer. This approach will aid in determining how emerging animal science tools can be used to produce healthier meat.
● Regarding sustainability, national accounting must be performed to confirm that an optimal intake of Se can be maintained via the intake of meat (and organs).
● The vitamin D content in meat should be maximized within legislative frames.
● Feeding legislation must be developed to stabilize sustainable I levels in cattle milk, the most important dietary source of I.
● The UL values of feed should be used to improve the nutrient density of meat while maintaining animal health and welfare.
● The nutrient density of meat must be maintained and improved. Consuming less but higher-quality meat should be a beneficial choice in the long run, especially for consumers vulnerable to hidden hunger.
Ethics statement
Written informed consent was obtained from the individual(s), and minor(s)’ legal guardian/next of kin, for the publication of any potentially identifiable images or data included in this article.
Author contributions
BE: Conceptualization, Writing – original draft, Data curation, Formal analysis, Methodology, Project administration, Writing – review & editing. VG-Å: Conceptualization, Project administration, Writing – original draft, Writing – review & editing. LM: Resources, Writing – original draft, Writing – review & editing. AH: Data curation, Formal analysis, Methodology, Validation, Writing – original draft, Writing – review & editing. EP: Conceptualization, Funding acquisition, Methodology, Project administration, Resources, Writing – original draft, Writing – review & editing.
Funding
The author(s) declare financial support was received for the research, authorship, and/or publication of this article. EP and LM are employed by NMBU (University), BE and AH have state pensions. VG-Å was funded by Foods of Norway, Research Project (Research Council of Norway; grant no. 237841/030).
Acknowledgments
We thank Eli Gjerlaug-Enger (Norsvin) and Morten Røe (Animalia) for providing historical slaughter data and Gisken Trøan for providing valuable input regarding iodine and milk. We also thank Professor Emeritus Odd Vangen for providing historical information and data related to pork breeding. We also thank University lector Elin Bjørge Løken for explaining the Norwegian Food Survey data acquired before 1980. We would like to thank Editage (www.editage.com) for English language editing.
Conflict of interest
The authors declare that the research was conducted in the absence of any commercial or financial relationships that could be construed as a potential conflict of interest.
Publisher’s note
All claims expressed in this article are solely those of the authors and do not necessarily represent those of their affiliated organizations, or those of the publisher, the editors and the reviewers. Any product that may be evaluated in this article, or claim that may be made by its manufacturer, is not guaranteed or endorsed by the publisher.
Supplementary material
The Supplementary Material for this article can be found online at: https://www.frontiersin.org/articles/10.3389/fanim.2024.1426044/full#supplementary-material
Abbreviations
AA, Arachidonic acid; AC, After Christ; ADG, Average Daily Gain; AI, Adequate Intake; ALA, α-Linolenic acid; ARC, Agricultural Research Council; BC, Before Christ; CVD, Cardiovascular disease; DGA, Dietary Guidelines for Americans; DM, Dry Matter; DHA, Docosahexaenoic acid; DPA, Docosapentaenoic acid; DRI, Dietary reference intake; E%, Energy percentage, i.e., percentage of total energy intake; EC, European Commission; EFSA, European Food Safety Authority; EPA, Eicosapentaenoic acid; EU, European Union; FA, Fatty acid; FAO, Food and Agriculture Organization; FCR, Feed Conversion Ratio; Fe, Iron; GPx, Glutathione peroxidase; HDL-C, High density lipoprotein cholesterol; HeFe, Heme iron; HHS, Department of Health and Human services; I, Iodine; IDA, Iron deficiency anemia; LDL, Low density lipoprotein; LA, Linoleic acid; LTL, Longissimus thoracis et lumborum; M-HDL-P, Number of medium HDL particles; MUFA, Monounsaturated fatty acids; NASEM, The National Academies of Sciences, Engineering, and Medicine in US; N-HeFe, Non-heme iron; NNR, Nordic Nutrition Recommendations; NRC, The National Research Council in US; PRI, Population Reference Intake; PUFA, Polyunsaturated fatty acids; RDA, Recommended daily allowances; RDI, Recommended Daily Intake; Rem-C, Remaining cholesterol; RI, Recommended intake; RS, Recommended supply; Se, Selenium; SFA, Saturated fatty acids; S-LDL-P, Number of small LDL particles; TF, Total fat; TFe, Total iron; TG, Triglycerides; TMR, Total mixed ration; UL, Upper limit; UN, United Nations; UV, Ultraviolet; UVB, Ultraviolet B; US, United States; USDA, United States Department of Agriculture; VitD, Vitamin D; VLDL-TG, Very low density –triglycerides; WHO, World Health Organization; Ø, Mean diameter.
References
Aglago E. K., Cross A. J., Riboli E., Fedirko V., Hughes D. J., Fournier A., et al. (2023). Dietary intake of total, heme and non-heme iron and the risk of colorectal cancer in a European prospective cohort study. Br. J. Cancer 128, 1529–1540. doi: 10.1038/s41416-023-02164-7
Alexander J., Olsen A. K. (2023). Selenium - a scoping review for Nordic nutrition recommendations 2023. Food Nutr. Res. 67, 1–7. doi: 10.29219/fnr.v67.10320
Amcoff E., Edberg A., Barbieri H. E., Lindroos A. K., Nälsén C., Pearson M., et al. (2012). “Riksmaten – vuxna 2010–11, Livsmedels- och näringsintag bland vuxna i Sverige,” in Livsmedelsdataenheten, Livsmedelsverket(Swedish). (ed.). Uppsala: Livsmedelsverket (Swedish Food Agency).
Andersson M., Karumbunathan V., Zimmermann M. B. (2012). Global iodine status in 2011 and trends over the past decade. J. Nutr. 142, 744–750. doi: 10.3945/jn.111.149393
Antaya N. T., Ghelichkhan M., Pereira A. B. D., Soder K. J., Brito A. F. (2019). Production, milk iodine, and nutrient utilization in Jersey cows supplemented with the brown seaweed Ascophyllum nodosum (kelp meal) during the grazing season. J. Dairy Sci. 102, 8040–8058. doi: 10.3168/jds.2019-16478
Antaya N. T., Soder K. J., Kraft J., Whitehouse N. L., Guindon N. E., Erickson P. S., et al. (2015). Incremental amounts of Ascophyllum nodosum meal do not improve animal performance but do increase milk iodine output in early lactation dairy cows fed high-forage diets. J. Dairy Sci. 98, 1991–2004. doi: 10.3168/jds.2014-8851
Apple J. K., Wallis-Phelps W. A., Maxwell C. V., Rakes L. K., Sawyer J. T., Hutchison S., et al. (2007). Effect of supplemental iron on finishing swine performance, carcass characteristics, and pork quality during retail display. J. Anim. Sci. 85, 737–745. doi: 10.2527/jas.2006-231
ARC. (1980). The nutrient requirements of ruminant livestock. Technical review. 2nd ed (Agric. Res. Coun. by the Commonwealth Agricultural Bureaux: UK). Available at: https://openlibrary.org/books.
Astrup A., Magkos F., Bier D. M., Brenna J. T., De Oliveira Otto M. C., Hill J. O., et al. (2020). Saturated fats and health: A reassessment and proposal for food-based recommendations: JACC State-of-the-Art Review. J. Am. Coll. Cardiol. 76, 844–857. doi: 10.1016/j.jacc.2020.05.077
Azizi-Soleiman F., Vafa M., Abiri B., Safavi M. (2016). Effects of iron on vitamin D metabolism: A systematic review. Int. J. Prev. Med. 7, 126–132. doi: 10.4103/2008-7802.195212
Babicz M., Kasprzyk A. (2019). Comparative analysis of the mineral composition in the meat of wild boar and domestic pig. Ital. J. Anim. Sci. 18, 1013–1020. doi: 10.1080/1828051x.2019.1610337
Bath S. C., Verkaik-Kloosterman J., Sabatier M., Ter Borg S., Eilander A., Hora K., et al. (2022). A systematic review of iodine intake in children, adults, and pregnant women in Europe-comparison against dietary recommendations and evaluation of dietary iodine sources. Nutr. Rev. 80, 2154–2177. doi: 10.1093/nutrit/nuac032
Biing-Hwan L., Guthrie J., Smith T. (20232023). Dietary quality by food source and demographics in the United States 1977–2018 (U.S.: Department of Agriculture. United States Department of Agriculture), EIB–249. Available at: https://www.ers.usda.gov/webdocs/publications/105956/eib-249.pdf?v=4454.8. ERS, ed.
Blaxter K. L., Sharman G. A., MacDonald A. M. (1957). Iron-deficiency anaemia in calves. Br. J. Nutr. 11, 234–246. doi: 10.1079/bjn19570043
Blunt J. W., Deluca H. F., Schnoes H. K. (1968). 25-hydroxycholecalciferol. A biologically active metabolite of vitamin D3. Biochemistry 7, 3317–3322. doi: 10.1021/bi00850a001
Breirem K., Homb T. (1958). Mineral-tilskudd til melkekyr (Norwegian: Landbrukshøyskolens institutt for husdyrernæring og fôringslære, Ås).
Brummer F. A., Pirelli G. J., Hal J. A. (2014). Selenium supplementation Strategies for livestock in Oregon. Oregon State: Extension Service. Available online at: https://extension.oregonstate.edu/sites/default/files/documents/em9094.pdf#EM%209094%20%20%E2%80%A2%20%20June%202014 (Accessed 03.04.2024).
Buffini M., Nugent A. P., Walton J., Flynn A., McNulty B. A. (2023). Selenium intakes in the Irish adult population. J. Nutr. Sci. 12, 1–10. doi: 10.1017/jns.2023.23
Bugel S., Sandstrom B., Skibsted L. H. (2004). Pork meat: a good source of selenium? J. Trace Elem. Med. Biol. 17, 307–311. doi: 10.1016/S0946-672X(04)80033-6
Burild A., Lauridsen C., Faqir N., Sommer H. M., Jakobsen J. (2016). Vitamin D3 and 25-hydroxyvitamin D3 in pork and their relationship to vitamin D status in pigs. J. Nutr. Sci. 5, e3. doi: 10.1017/jns.2015.28
Cabrita A. R. J., Maia M. R. G., Oliveira H. M., Sousa-Pinto I., Almeida A. A., Pinto E., et al. (2016). Tracing seaweeds as mineral sources for farm-animals. J. App. Phycol. 28, 3135–3150. doi: 10.1007/s10811-016-0839-y
Callforlife. (2024). Jungle fowl, thigh nutrition facts (Calorie and nutrient database Callforlife). Available online at: https://www.calforlife.com/calories/jungle-fowl-thigh (Accessed 05.01.2024).
Cashman K. D., Seamans K. M., Lucey A. J., Stocklin E., Weber P., Kiely M., et al. (2012). Relative effectiveness of oral 25-hydroxyvitamin D3 and vitamin D3 in raising wintertime serum 25-hydroxyvitamin D in older adults. Am. J. Clin. Nutr. 95, 1350–1356. doi: 10.3945/ajcn.111.031427
Chepkemoi M., W. M. J., Sila D., Oyier P., Malaki P., Ndiema E., et al. (2017). Physical characteristics and nutritional composition of meat and eggs of five poultry species in Kenya. Livest. Res. Rural Dev. 29. Available at: http://www.lrrd.org/lrrd29/8/somm29153.html.
Christophersen O. A., Lyons G., Haug A., Steinnes E. (2013). “Selenium,” in heavy metals in soils. Ed. Alloway B. J. Whiteknights, Reading, UK: Springer University of Reading, 429–463. doi: 10.1007/978-94-007-4470-7_16
Cicual. (2020). French Food Composition database. Chicken, meat, raw. Selenium 2009. Available online at: https://ciqual.anses.fr/#/aliments/36003/chicken-meat-raw (Accessed 25.02.2024).
Coffey D., Dawson K., Ferket P., Connolly A. (2016). Review of the feed industry from a historical perspective and implications for its future. J. Appl. Anim. Nutr. 4, 3. doi: 10.1017/jan.2015.11
Consalez F., Ahern M., Andersen P., Kjellevold M. (2022). The effect of the meat factor in animal-source foods on micronutrient absorption: A scoping review. Adv. Nutr. 13, 2305–2315. doi: 10.1093/advances/nmac089
Cross A. J., Harnly J. M., Ferrucci L. M., Risch A., Mayne S. T., Sinha R. (2012). Developing a heme iron database for meats according to meat type, cooking method and doneness level. Food Nutr. Sci. 3, 905–913. doi: 10.4236/fns.2012.37120
Cruickshank K. M., Hatew B., Gehman A. M., Koenig K. M., Ribeiro E. S., Steele M. A. (2021). Effect of supplementary selenium source on dairy cow performance, Antioxidant Status, and apparent absorption and retention. J. Anim. Sci. 99, 80–80. doi: 10.1093/jas/skab235.145
Dahl L., Opsahl J. A., Meltzer H. M., Julshamn K. (2003). Iodine concentration in Norwegian milk and dairy products. Br. J. Nutr. 90, 679–685. doi: 10.1079/bjn2003921
Dicken S. J., Batterham R. L. (2022). Ultra-processed food: a global problem requiring a global solution. Lancet Diabetes Endocrinol. 10, 691–694. doi: 10.1016/S2213-8587(22)00248-0
Dijck-Brouwer D. A. J., Muskiet F. A. J., Verheesen R. H., Schaafsma G., Schaafsma A., Geurts J. M. W. (2022). Thyroidal and extrathyroidal requirements for iodine and selenium: A combined evolutionary and (patho)physiological approach. Nutrients 14, 1–29. doi: 10.3390/nu14193886
Directory of Health. (2023). Utviklingen i norsk kosthold (Norwegian). Available online at: www.helsedirektoratet.no/rapporter/utviklingen-i-norsk-kosthold (Accessed 18.04 2024).
Durrant L. R., Bucca G., Hesketh A., Moller-Levet C., Tripkovic L., Wu H., et al. (2022). Vitamins D2 and D3 have overlapping but different effects on the human immune system revealed through analysis of the blood transcriptome. Front. Immunol. 13, e790444. doi: 10.3389/fimmu.2022.790444
Dydykin A. S., Aslanova M. A., Derevitskaya О.К., Soldatova N. E. (2019). Effectiveness of using iodine-containing additives in meat products for child nutrition. IOP Conf. Ser.: Earth Environ. Sci. 333, 012060. doi: 10.1088/1755-1315/333/1/012060
Dzik K. P., Kaczor J. J. (2019). Mechanisms of vitamin D on skeletal muscle function: oxidative stress, energy metabolism and anabolic state. Eur. J. Appl. Physiol. 119, 825–839. doi: 10.1007/s00421-019-04104-x
EC. (2015). Commission implementing regulation (EU) 2015/861 of 3 June 2015 concerning the authorisation of potassium iodide, calcium iodate anhydrous and coated granulated calcium iodate anhydrous as feed additives for all animal species. OJEU. C/2015/3628. Available online at: https://eur-lex.europa.eu/legal-content. (Accessed: April 24, 2024).
EC. (2017). Corrigendum to Commission Implementing Regulation (EU) 2017/2330 of 14 December 2017 concerning the authorisation of Iron(II) carbonate, Iron(III) chloride hexahydrate, Iron(II) sulphate monohydrate, Iron(II) sulphate heptahydrate, Iron(II) fumarate, Iron(II) chelate of amino acids hydrate, Iron(II) chelate of protein hydrolysates and Iron(II) chelate of glycine hydrate as feed additives for all animal species and of Iron dextran as feed additive for piglets and amending Regulations (EC) No 1334/2003 and (EC) No 479/2006. OJEU. L 351/203. Available online at: https://eur-lex.europa.eu/legal-content. (Accessed: April 24, 2024).
EC. (2019). Commision Implementing Regulation (EU) 2019/49 of 4 January 2019 concerning the authorisation of sodium selenite, coated granulated sodium selenite and zinc-L-selenomethionine as feed additives for all animal species. OJEU. L 10/2. Available online at: https://eur-lex.europa.eu/legal-content. (Accessed: April 24, 2024).
EC. (2020). Commission implementing regulation (EU) 2020/377 of 5 March 2020 concerning the authorisation of sodium selenate as a feed additive for ruminants. OJEU. C/2020/1234. Available online at: https://eur-lex.europa.eu/legal-content. (Accessed: April 24, 2024).
EC. (2023). Saturated fat intake across the EU, Norway and the United Kingdom. Available online at: https://knowledge4policy.ec.europa.eu/health-promotion-knowledge-gateway/dietary-fats-5b_en. (Accessed: Sept 11, 2024)
EFSA. (2013). Scientific Opinion on the safety and efficacy of iodine compounds (E2) as feed additives for all species: calcium iodate anhydrous and potassium iodide, based on a dossier submitted by HELM AG. EFSA J. 11, e3101. doi: 10.2903/j.efsa.2013.3101
EFSA. (2014). Scientific Opinion on the Safety and efficacy of vitamin D3 (cholecalciferol) as a feed additive for all animal species or categories based on a dossier submitted by Lohmann Animal Health GmbH. Available online at: https://efsa.onlinelibrary.wiley.com/doi/epdf/10.2903/j.efsa.2014.3568.
EFSA. (2016). Dietary reference values for vitamin D. EFSA J. 14, e04547. doi: 10.2903/j.efsa.2016.4547
EFSA. (2021). Panel on Additives and Products or Substances used in Animal Feed (FEEDAP), Bampidis V, Azimonti G, Bastos ML, Christensen H, Dusemund B, et al. Safety and efficacy of the feed additive consisting of selenium-enriched yeast (Saccharomyces cerevisiae CNCM I-3060) for all animal species (Alltech Ireland). EFSA J. 19, e06979. doi: 10.2903/j.efsa.2021.6979
EFSA. (2023). Panel on Nutrition, Novel Foods and Food Allergens (NDA). (Turck D, et al.) Scientific opinion on the tolerable upper intake level for selenium. EFSA J. 21, e07704. doi: 10.2903/j.efsa.2023.7704
EFSA. (2024a). Draft scientific opinion on the tolerable upper intake level for iron. Available online at: https://connect.efsa.europa.eu/RM/s/publicconsultation2/a0lTk000000Af57/pc0835 (Accessed 29.05.2024).
EFSA. (2024b). EFSA: science, safe food, sustainability. Available online at: https://www.efsa.europa.eu/en (Accessed 18.04.2024).
Egelandsdal B., Oostindjer M., Hovland E. M., Okholm B., Saarem K., Bjerke F., et al. (2020). Identifying labelling and marketing advantages of nutrients in minced beef meat: A case study. Meat Sci. 159, e107920. doi: 10.1016/j.meatsci.2019.107920
Engle-Stone R., Haile D., Luo H. (2022). We pose some uncertainties in the analysis of national trends in iron intake and risk of deficiency, but bupport the need for addressing iron deficiency among vulnerable groups in the United States. J. Nutr. 152, 639–640. doi: 10.1093/jn/nxab360
Evvard J. M., Culbertson C. C. (1924). Iodine, a factor in feeding young growing swine. Proc. Iowa Acad. Sci. 31, 309–317. Available at: https://scholarworks.uni.edu/pias/vol31/iss1/89.
Fairweather-Tait S. (2023). The role of meat in iron nutrition of vulnerable groups of the UK population. Front. Anim. Sci. 4, e1142252. doi: 10.3389/fanim.2023.1142252
FAO. (1950). Calorie requirements: report of the Committee of Calorie Requirements. Sept. 1949. FAO Nutr. Stud. 5, 1-97. Available at: https://www.fao.org/nutrition/requirements/archive/en/.
FAO. (1958). Protein requirements: report of the FAO Committe Oct. 1955. FAO Nutr. Ser. 16, 1-118. Available at: https://www.fao.org/nutrition/requirements/archive/en/.
FAO. (2010). Fats and fatty acids in human nutrition (Rome: FAO Food Nutri. pap 91 Food and Agriculture Organization of the United Nations, FAO).
FAO. (2023). Meat Market Review: Emerging trends and outlook 2023 (Rome: Food and Agriculture Organization of the United Nations, Rome, Italy). Available at: https://www.fao.org/3/cc9074en/cc9074en.pdf.
FAO/WHO. (1970). Requirements of ascorbic acid, vitamin D, vitamin B12, folate and iron. WHO Techn. Rep. Ser. 452, 1-76.
FAO/WHO. (1973). Energy and protein requirements. WHO Tech. Rep.Ser. 552, 329-332. doi: 10.1002/food.19740180314
FAO/WHO. (2002). Diet, nutrition and the prevention of chronic diseases. WHO Tech. Rep. Ser. 916, 1-149.
FAO/WHO. (2004). Human vitamin and mineral requirements (with 1998 expert consultation) (Geneva: FAO/WHO NFS team).
FAO/WHO. (2019). Sustainable healthy diets- guiding principles (Rome: Food and Agriculture Organization of the United Nations/WORLD HEALTH ORGANIZATION, Rome, Italy). Available at: https://iris.who.int.
Ferrari M., Mistura L., Patterson E., Sjostrom M., Diaz L. E., Stehle P., et al. (2011). Evaluation of iron status in European adolescents through biochemical iron indicators: the HELENA Study. Eur. J. Clin. Nutr. 65, 340–349. doi: 10.1038/ejcn.2010.279
Gjerlaug-Enger E. (2011). Genetic analyses of meat, fat and carcass quality traits measured by rapid methods. Philosophiae Doctor (PhD) Thesis No. 13, Norwegian University of Life Sciences (NMBU), Ås, Norway.
Gjerlaug-Enger E., Aass L., Odegard J., Kongsro J., Vangen O. (2011). Genetic parameters of fat quality in pigs measured by near-infrared spectroscopy. Animal 5, 1495–1505. doi: 10.1017/S1751731111000528
Gjerlaug-Enger E., Haug A., Gaarder M., Ljokjel K., Stenseth R. S., Sigfridson K., et al. (2015). Pig feeds rich in rapeseed products and organic selenium increased omega-3 fatty acids and selenium in pork meat and backfat. Food Sci. Nutr. 3, 120–128. doi: 10.1002/fsn3.182
Grabež V., Coll-Brasas E., Fulladosa E., Hallenstvedt E., Haseth T. T., Overland M., et al. (2022). Seaweed inclusion in finishing lamb diet promotes changes in micronutrient content and flavour-Related compounds of raw meat and dry-Cured leg (Fenalår). Foods 11, e1043. doi: 10.3390/foods11071043
Grabež V., Devle H., Kidane A., Mydland L. T., Overland M., Ottestad S., et al. (2023a). Sugar Kelp (Saccharina latissima) Seaweed added to a growing-finishing lamb diet has a positive effect on quality traits and on mineral content of meat. Foods 12, e2131. doi: 10.3390/foods12112131
Grabež V., Mydland L. T., Papoutsis D., Øverland M., Egelandsdal B. (2023b). Effect of low-dose blanched Saccharina latissima in finishing bulls’ diets on carcass and meat quality traits. Front. Anim. Sci. 4, e1233244. doi: 10.3389/fanim.2023.1233244
Guggenheim K. Y. (1995). Chlorosis: the rise and disappearance of a nutritional disease. J. Nutr. 125, 1822–1825. doi: 10.1093/jn/125.7.1822
Gunnarsdóttir S., Guðmannsdóttir R., Þorgeirsdóttir H., Torfadóttir J. E., Steingrímsdóttir L., Tryggvadóttir E. A., et al. (2010-2011). Hvað borða Íslendingar? Könnun á mataræði Íslendinga 2019-2021 (In Iclandic) (Reykjavik: Directorate of Health).
Hallberg L., Rossander-Hulthen L. (1989). Prevalence of iron deficiency in European countries and attempts to analyze possible causes of differences. Bibl. Nutr. Dieta 44, 94–105. doi: 10.1159/000417305
Halmemies-Beauchet-Filleau A., Rinne M., Lamminen M., Mapato C., Ampapon T., Wanapat M., et al. (2018). Review: Alternative and novel feeds for ruminants: nutritive value, product quality and environmental aspects. Animal 12, 295–309. doi: 10.1017/S1751731118002252
Hartikainen H. (2005). Biogeochemistry of selenium and its impact on food chain quality and human health. J. Trace Elem. Med. Biol. 18, 309–318. doi: 10.1016/j.jtemb.2005.02.009
Haug A., Eich-Greatorex S., Bernhoft A., Wold J. P., Hetland H., Christophersen O. A., et al. (2007a). Effect of dietary selenium and omega-3 fatty acids on muscle composition and quality in broilers. Lipids Health Dis. 6, 29–37. doi: 10.1186/1476-511X-6-29
Haug A., Graham R. D., Christophersen O. A., Lyons G. H. (2007b). How to use the world's scarce selenium resources efficiently to increase the selenium concentration in food. Microb. Ecol. Health Dis. 19, 209–228. doi: 10.1080/08910600701698986
Haug A., Nyquist N. F., Mosti T. J., Andersen M., Hostmark A. T. (2012a). Increased EPA levels in serum phospholipids of humans after four weeks daily ingestion of one portion chicken fed linseed and rapeseed oil. Lipids Health Dis. 11, e104. doi: 10.1186/1476-511X-11-104
Haug A., Taugbol O., Prestlokken E., Govasmark E., Salbu B., Schei I., et al. (2012b). Iodine concentration in Norwegian milk has declined in the last decade. Acta Agric. Scand. Section A -Anim. Sci. 62, 127–134. doi: 10.1080/09064702.2012.754932
Haug A., Vermeer C., Ruud L., Monfort-Pires M., Grabez V., Egelandsdal B. (2022). Nutrient-optimized beef enhances blood levels of vitamin D and selenium among young women. Foods 11, e631. doi: 10.3390/foods11050631
Haug A., Vhile S. G., Berg J., Hove K., Egelandsdal B. (2018). Feeding potentially health promoting nutrients to finishing bulls changes meat composition and allow for product health claims. Meat Sci. 145, 46–468. doi: 10.1016/j.meatsci.2018.07.015
Helldán A., Raulio S., Kosola M., Tapanainen H., Ovaskainen M.-L., Virtanen S. (2013). The National FINDIET 2012 Survey (Tampere, Finland: Juvenes Print - Suomen Yliopistopaino Oy). Available at: https://urn.fi/URN.
Henjum S., Aakre I., Lilleengen A. M., Garnweidner-Holme L., Borthne S., Pajalic Z., et al. (2018). Suboptimal iodine status among pregnant women in the Oslo area, Norway. Nutrients 10, e280. doi: 10.3390/nu10030280
Hocquette J.-F., Chriki S., Fournier D., Ellies-Oury M.-P. (2024). Review: Will “cultured meat” transform our food system towards more sustainability? Animal [In Press]. doi: 10.1016/j.animal.2024.101145
Homb T. (1979). Grain and other concentrates in Norwegian animal production (In Norwegian) (Steinkjer, Norway: Statens Kornforretning).
Hotz C. S., Fitzpatrick D. W., Trick K. D., L'abbe M. R. (1997). Dietary iodine and selenium interact to affect thyroid hormone metabolism of rats. J. Nutr. 127, 1214–1218. doi: 10.1093/jn/127.6.1214
IGN. (2022). Global scorecard of iodine nutrition in 2022 in the general population based on school-age children (SAC) (Ottawa, Canada: IGN). Available online at: https://ign.org/app/uploads/2024/01/Scorecard_2023_References_July-2023_Final.pdf (Accessed 01.11.2024).
Itkonen S. T., Andersen R., Bjork A. K., Brugard Konde A., Eneroth H., Erkkola M., et al. (2021). Vitamin D status and current policies to achieve adequate vitamin D intake in the Nordic countries. Scand. J. Public Health 49, 616–627. doi: 10.1177/1403494819896878
Ittermann T., Albrecht D., Arohonka P., Bilek R., De Castro J. J., Dahl L., et al. (2020). Standardized map of iodine status in Europe. Thyroid 30, 1346–1354. doi: 10.1089/thy.2019.0353
Jahreis G., Hausmann W., Kiessling G., Franke K., Leiterer M. (2001). Bioavailability of iodine from normal diets rich in dairy products–results of balance studies in women. Exp. Clin. Endocrinol. Diabetes 109, 163–167. doi: 10.1055/s-2001-14840
Jakobsen J. (2007). Bioavailability and bioactivity of vitamin D3 active compounds – Which potency should be used for 25-hydroxyvitamin D3? Int. Congr. Ser. 1297, 133–142. doi: 10.1016/j.ics.2006.08.026
Jones G. (2022). 100 years of vitamin D: Historical aspects of vitamin D. Endocr. Connect. 11, e210594. doi: 10.1530/EC-21-0594
Jones G. D., Droz B., Greve P., Gottschalk P., Poffet D., Mcgrath S. P., et al. (2017). Selenium deficiency risk predicted to increase under future climate change. Proc. Natl. Acad. Sci. U.S.A. 114, 2848–2853. doi: 10.1073/pnas.1611576114
Kasprzyk A., Stadnik J., Stasiak D. (2019). Technological and nutritional properties of meat from female wild boars (Sus scrofa scrofa L.) of different carcass weights. Arch. Anim. Breed 62, 597–604. doi: 10.5194/aab-62-597-2019
Kelly O. J., Gilman J. C., Ilich J. Z. (2018). Utilizing dietary micronutrient ratios in nutritional research may be more informative than focusing on single nutrients. Nutrients 11, e282. doi: 10.3390/nu10010107
Kim Y.-A., Cho Y. J. (2019). The association between visceral fat, subcutaneous fat and serum 25-hydroxyvitamin D3 levels. Obes. Med. 13, 29–33. doi: 10.1016/j.obmed.2018.12.005
Knap P. W., Wang L. (2012). “Pig breeding for improved feed efficiency,” in Feed efficiency in swine. Ed. Patience J. F. (Wageningen Academic Press Publishers, Wageningen), 167–181.
Landsforeningen for Kosthold og Helse. (1988). Statens ernæringsråds matvaretabell, vedlegg 2: Fettsyreinnhold i matvarer, product 3.148 (In Norwegian) (Oslo: Statens ernæringsråd).
Larson E. J. (2006). Evolution: The remarkable history of a scientific theory (Modern Library Chronicles) (New York, USA: Random House Publishing Group), 221–243.
Lee K. W., Shin D., Cho M. S., Song W. O. (2016). Food group intakes as determinants of iodine status among US adult population. Nutrients 8, 1–13. doi: 10.3390/nu8060325
Lemming E. W., Pitsi T. (2022). The Nordic Nutrition Recommendations 2022 - food consumption and nutrient intake in the adult population of the Nordic and Baltic countries. Food Nutr. Res. 66, e8572. doi: 10.29219/fnr.v66.8572
Liu J., Almeida J. M., Rampado N., Panea B., Hocquette E., Chriki S., et al. (2023). Perception of cultured "meat" by Italian, Portuguese and Spanish consumers. Front. Nutr. 10, e1043618. doi: 10.3389/fnut.2023.1043618
Lush J. L. (1931). The number of daughters necessary to prove a sire. J. Dairy Sci. 14, 209–220. doi: 10.3168/jds.S0022-0302(31)93466-8
Mackenzie J. S., Jeggo M. H. (2011). 1st international one health congress. EcoHealth, 2011 1–2. doi: 10.1007/s10393-011-0676-z
Madson D. M., Ensley S. M., Gauger P. C., Schwartz K. J., Stevenson G. W., Cooper V. L., et al. (2012). Rickets: case series and diagnostic review of hypovitaminosis D in swine. J. Vet. Diagn. Invest. 24, 1137–1144. doi: 10.1177/1040638712461487
Makkar H. P. S. (2018). Review: Feed demand landscape and implications of food-not feed strategy for food security and climate change. Animal 12, 1744–1754. doi: 10.1017/S175173111700324X
Makkar H. P. S., Tran G., Heuzé V., Giger-Reverdin S., Lessire M., Lebas F., et al. (2016). Seaweeds for livestock diets: A review. Anim. Feed Sci. Technol. 212, 1–17. doi: 10.1016/j.anifeedsci.2015.09.018
Malbe M., Klaassen M., Fang W., Myllys V., Vikerpuur M., Nyholm K., et al. (1995). Comparisons of selenite and selenium yeast feed supplements on Se-incorporation, mastitis and leucocyte function in Se-deficient dairy cows. Zentralbl. Veterinarmed. A 42, 111–121. doi: 10.1111/j.1439-0442.1995.tb00362.x
Mallol R., Amigo N., Rodriguez M. A., Heras M., Vinaixa M., Plana N., et al. (2015). Liposcale: a novel advanced lipoprotein test based on 2D diffusion-ordered 1H NMR spectroscopy. J. Lipid Res. 56, 737–746. doi: 10.1194/jlr.D050120
Marles R. J. (2017). Mineral nutrient composition of vegetables, fruits and grains: The context of reports of apparent historical declines. J. Food Compost. Anal. 56, 93–103. doi: 10.1016/j.jfca.2016.11.012
McGovern G. (1977). Nutrition and human needs, dietary goals for the United States: US Senate Select Committee (Washington DC, USA: US Government Printing Office).
McGuire S. (2015). Comprehensive implementtion plan on maternal, infant, and young child nutrition. WHO, Geneva, Switzerland 2014. Adv. Nutr. 6, 134–135. doi: 10.3945/an.114.007781
Meyer U., Weigel K., Schöne F., Leiterer M., Flachowsky G. (2008). Effect of dietary iodine on growth and iodine status of growing fattening bulls. Livest. Sci. 115, 219–225. doi: 10.1016/j.livsci.2007.07.013
Ministry of Health Brazil. (2015). Dietary guidelines for the Brazilian population. Available online at: https://bvsms.saude.gov.br/bvs/publicacoes/dietary_guidelines_Brazilian_population.pdf. (Accessed: Sept 11, 2024)
Mitchell H. H., McClure F. J. (1937). Mineral nutrition of farm animals, Committee on Animal Nutrition, Division of Biology and Agriculture, National Research Council. Bull. Natl. Res. Coun. 99, 1937. Available at: https://www.cabidigitallibrary.org/doi/full/10.5555/19371404117.
Monfort-Pires M., Lamichhane S., Alonso C., Egelandsdal B., Oresic M., Jordahl V. O., et al. (2023). Classification of common food lipid sources regarding healthiness using advanced lipidomics: A four-Arm crossover study. Int. J. Mol. Sci. 24, e4941. doi: 10.3390/ijms24054941
Morrison M. C., Egelandsdal B., Harvei S., Rocha S. D. C., Pieterman E. J., Kleemann R., et al. (2023). Differential effects of plant and animal fats on obesity-induced dyslipidemia and atherosclerosis in Ldlr-/-.Leiden mice. FASEB J. 37, e23096. doi: 10.1096/fj.202300585R
Mozaffarian D., Rosenberg I., Uauy R. (2018). History of modern nutrition science-implications for current research, dietary guidelines, and food policy. BMJ 361, k2392. doi: 10.1136/bmj.k2392
Nair K. M., Augustine L. F. (2018). Food synergies for improving bioavailability of micronutrients from plant foods. Food Chem. 238, 180–185. doi: 10.1016/j.foodchem.2016.09.115
NASEM. (2006). Institute of Medicine of National Academies, Dietary eference intakes: The essential guide to nutrient requirements (Washington, DC: The National Academies Press).
NASEM. (2016). Nutrient requirements of beef cattle. 8th rev. ed (Washington, DC: The National Academies Press).
Neill H. R., Gill C. I. R., McDonald E. J., McMurray R., McRoberts W. C., Loy R., et al. (2023). Improving vitamin D content in pork meat by UVB biofortification. Meat Sci. 199, e109115. doi: 10.1016/j.meatsci.2023.109115
Neill H. R., Gill C. I. R., McDonald E. J., McRoberts W. C., Rosbotham E. J., Boland R., et al. (2021). Improving vitamin D content in pork meat by UV bio-enrichment. Proc. Nutr. Soc 80:E140. doi: 10.1017/s0029665121002639.
Newcom D. W., Stalder K. J., Baas T. J., Goodwin R. N., Parrish F. C., Wiegand B. R. (2004). Breed differences and genetic parameters of myoglobin concentration in porcine longissimus muscle. J. Anim. Sci. 82, 2264–2268. doi: 10.2527/2004.8282264x
Newman J. C., Malek A. M., Hunt K. J., Marriott B. P. (2019). Nutrients in the US Diet: Naturally occurring or enriched/fortified food and beverage sources, plus dietary supplements: NHANES 2009-2012. J. Nutr. 149, 1404–1412. doi: 10.1093/jn/nxz066
Newton E. E., Petursdottir A. H., Rikharethsson G., Beaumal C., Desnica N., Giannakopoulou K., et al. (2021). Effect of dietary seaweed supplementation in cows on milk macrominerals, Trace elements and heavy metal concentrations. Foods 10, e1526. doi: 10.3390/foods10071526
Newton E. E., Theodoridou K., Terre M., Huws S., Ray P., Reynolds C. K., et al. (2023). Effect of dietary seaweed (Ascophyllum nodosum) supplementation on milk mineral concentrations, transfer efficiency, and hematological parameters in lactating Holstein cows. J. Dairy Sci. 106, 6880–6893. doi: 10.3168/jds.2022-23074
Niero G., Visentin G., Censi S., Righi F., Manuelian C. L., Formigoni A., et al. (2023). Invited review: Iodine level in dairy products-A feed-to-fork overview. J. Dairy Sci. 106, 2213–2229. doi: 10.3168/jds.2022-22599
NNR. (1989). Nordic Nutrient Recommendations 1989. Available online at: https://www.norden.org/en/nordic-council-ministers.
NNR. (1996). Nordic Nutrition Recommendations 1996 (Sandstrøm, B. et al.). Scand. J. Nutr. 40, 161–165.
NNR. (2004). Nordic Nutrition Recommendations 2004. Integrating nutrition and physical activity, Copenhagen 2004, Scanprint AS, Århus. (Oslo, Norway: Sosial og helsedirektoratet IS-1219) (Accessed: Sept 11, 2024)
NNR. (2012). Nordic Nutrition Recommendations 2012. Integrating nutrition and physical activity. Available online at: https://norden.diva-portal.org/smash/get/diva2:704251/FULLTEXT01.pdf. (Accessed: Sept 11, 2024).
NNR. (2023). Nordic Nutrition Recommendations 2023 -Integrating Environmental Aspects. (Blomhoff, R. et al.). Available online at: https://pub.norden.org/nord2023-003/nord2023-003.pdf.
Norwegian Parliament. (1975). Om norsk ernærings- og matforsyningspolitikk, (1975-1976). Report 32. (In Norwegian) (Oslo, Norway: Ministry of Agriculture). Available at: https://www.stortinget.no/no/Saker-og-publikasjoner/Stortingsforhandlinger/Lesevisning/?p=1975-76&paid=3&wid=d&psid=DIVL97&pgid=d_0095.
NRC. (1953). “Recommended nutrient allowances for swine,” in Recommended nutrient allowances for domestic animals, 2nd rev. ed (National Research Council (U.S, Washington DC).
NRC. (1954). Nutrient requirements for poultry (Washington DC: The National Academies Press). doi: 10.17226/21448. 3rd rev. National Research Council (U.S.).
NRC. (1958). Nutrient Requirements of Dairy Cattle. 2nd rev, ed (Washington DC: The National Academies Press).
NRC. (1966). Nutrient Requirements of Poultry. 5th rev ed (Washington DC: The National Academies Press).
NRC. (1971a). Nutrient Requirements of Dairy Cattle. 4th rev. ed (Washington DC: The National Academies Press).
NRC. (1971b). Nutrient Requirements of Poultry. 6th rev. ed (Washington DC: The National Academies Press).
NRC. (1978). Nutrient Requirements of Dairy Cattle. 5th rev. ed (Washington DC: The National Academies Press).
NRC. (1979). Nutrient Requirements of Swine. 8th rev. ed (Washington DC: The National Academies Press).
NRC. (1994). Nutrient Requirements of Poultry. 9th rev. ed (Washington DC: The National Academies Press).
NRC. (2012). Nutrient Requirements of Swine. 11th rev. ed (Washington DC: National Academies Press).
Nystrom H. F., Brantsaeter A. L., Erlund I., Gunnarsdottir I., Hulthen L., Laurberg P., et al. (2016). Iodine status in the Nordic countries - past and present. Food Nutr. Res. 60, e31969. doi: 10.3402/fnr.v60.31969
Olsson V., Pickova J. (2005). The influence of production systems on meat quality, with emphasis on pork. Ambio 34, 338–343. doi: 10.1639/0044-7447(2005)034[0338:tiopso]2.0.co;2
Ortman K., Pehrson B. (1999). Effect of selenate as a feed supplement to dairy cows in comparison to selenite and selenium yeast. J. Anim. Sci. 77, 3365–3370. doi: 10.2527/1999.77123365x
Oster O., Schmiedel G., Prellwitz W. (1988). The organ distribution of selenium in German adults. Biol. Trace Elem. Res. 15, 23–45. doi: 10.1007/BF02990125
Palacios C., Gonzalez L. (2014). Is vitamin D deficiency a major global public health problem? J. Steroid Biochem. Mol. Biol. 144 Pt A, 138–145. doi: 10.1016/j.jsbmb.2013.11.003
Pasricha S. R., Moir-Meyer G. (2023). Measuring the global burden of anaemia. Lancet Haematol. 10, e696. doi: 10.1016/S2352-3026(23)00171-0
Pedersen A. N., Christensen T., Matthiessen J., Knudsen V. K., Rosenlund-Sørensen M., Biltoft-Jensen A., et al. (2015). Danskernes kostvaner 2011-2013 (In Danish). Ed. Ernæring D. F. A. F. (Albertslund: DTU National Food Institute (English)).
Pellattiero E., Tasoniero G., Cullere M., Gleeson E., Baldan G., Contiero B., et al. (2020). Are meat quality traits and sensory attributes in favor of slow-growing chickens? Anim. (Basel) 10, e960. doi: 10.3390/ani10060960
Pinto X., Masana L., Civeira F., Real J., Ibarretxe D., Candas B., et al. (2020). Consensus document of an expert group from the Spanish Society of Arteriosclerosis (SEA) on the clinical use of nuclear magnetic resonance to assess lipoprotein metabolism (Liposcale(R)). Clin. Investig. Arterioscler. 32, 219–229. doi: 10.1016/j.arteri.2020.04.004
Poskitt E. M. (2003). Early history of iron deficiency. Br. J. Haematol. 122, 554–562. doi: 10.1046/j.1365-2141.2003.04529.x
Rana M. S., Campbell D. L. M. (2021). Application of ultraviolet light for poultry production: A review of impacts on behavior, physiology, and production. Front. Anim. Sci. 2, e699262. doi: 10.3389/fanim.2021.699262
Renaa T., Staveland K. (1974). Undersøkelse av innholdet av jod i melk fra forskjellige steder i Norge i 12-måneders-perioden juli 1971-juni 1972. (In Norwegian). Tidsk. norske Lægeforening 94, 990–993.
Ringen J. (1939). The feed value of seaweed meal (In Norwegian) (Norges landbrukshøyskole, Ås: Fôringsforsøkene).
RIVM. (2023a). Dutch Food Composition Database. Available online at: https://www.rivm.nl/en/dutch-food-composition-database (Accessed 07.08.2024).
RIVM. (2023b). Dutch Food Composition Database- Chicken w skin raw NEVO code 108. Available online at: https://nevo-online.rivm.nl/Home/En (Accessed 07.08.2024).
RIVM. (2023c). Dutch Food Composition Database-Pork fillet raw. Nevo Code 1418. Available online at: https://nevo-online.rivm.nl/Home/En (Accessed 07.08.2024).
RIVM. (2023d). Dutch Food Composition Database-Minced pork raw. Nevo code 1421. 08. Available online at: https://nevo-online.rivm.nl/Home/En (Accessed 07.08.2024).
RIVM. (2023e). Dutch Food Composition Database-Mince chicken raw Nevo code 3139. 08. Available online at: https://nevo-online.rivm.nl/Home/En (Accessed 07.08.2024).
RIVM. (2023f). Dutch Food Composition Database-Minced beef raw Nevo code 1405. 08. Available online at: https://nevo-online.rivm.nl/Home/En (Accessed 07.08.2024).
RIVM. (2024). DNFCS 2019-2021: Mean contribution of food sources to intake of nutrients. Available online at: https://statline.rivm.nl/#/RIVM/nl/dataset/50122NED/table?ts=1718976020313 (Accessed 07.08.2024).
Roberts C., Steer T., Maplethorpe N., Cox L., Meadows S., Nicholson S., et al. (2018). National diet and nutrition survey, results from years 7 and 8 (combined) of the Rolling Programme, (2014/2015 to 2015/2016) (London: Public Health England Road). Agency, P. H. E. A. F. S. (ed.).
Rosbotham E. J., Gill C. I. R., McDonald E. J., Mcroberts W. C., Rainey N., Loy R., et al. (2022). “Enhanced vitamin D content of chicken by UVB bio-enrichment does not influence sensory evaluation,” in Irish Section Conference 2022, 15–17 June 2022, Proc. Nutr. Soc.-Impact of nutrition science to human health: past perspectives and future directions, Vol. 81. Cambridge, UK: Cambridge University Press. doi: 10.1017/s0029665122001835
Roser M., Ritchie H., Rosado P. (2023). Food supply-Our world in data. Available online at: https://ourworldindata.org/food-supply (Accessed 01.07.2024).
Sambugaro N., Egelandsdal B., Grabez V., Røe M., Zotte A. D., Therkildsen M., et al. (2023). “Identifying possible market advantages of meat from native endangered cattle,” in 69th International Congress of Meat Science and Technology, Padua, Italy: 69th International Congress of Meat Science and Technology, Aug 20, 2023 to Aug 25, 2023. (Helsinki, Finland: DigICoMST, leader Eero Puolanne Viikki).
Sampels S., Jonsson M., Sandgren M., Karlsson A., Segerkvist K. A. (2023). Sustainable delicacy: variation in quality and sensory aspects in wild boar (Sus scrofa). Meat and comparison to pork meat-A case study. Foods 12, e1664. doi: 10.3390/foods12081644
Schmid A., Walther B. (2013). Natural vitamin D content in animal products. Adv. Nutr. 4, 453–462. doi: 10.3945/an.113.003780
Schutkowski A., Kramer J., Kluge H., Hirche F., Krombholz A., Theumer T., et al. (2013). UVB exposure of farm animals: study on a food-based strategy to bridge the gap between current vitamin D intakes and dietary targets. PloS One 8, e69418. doi: 10.1371/journal.pone.0069418
Sharma S., Neves L., Funderud J., Mydland L. T., Øverland M., Horn S. J. (2018). Seasonal and depth variations in the chemical composition of cultivated Saccharina latissima. Algal Res. 32, 107–112. doi: 10.1016/j.algal.2018.03.012
Siegel P. B. (2014). Evolution of the modern broiler and feed efficiency. Annu. Rev. Anim. Biosci. 2, 375–385. doi: 10.1146/annurev-animal-022513-114132
Skobrák E. B., Bodnár K., Jónás E. M., Gundel J., Jávor A. (2011). The comparison analysis of the main chemical composition parameters of wild boar meat and pork. J. Anim. Sci. Biotech. 44, 105–112.
Spiro A., Buttriss J. L. (2014). Vitamin D: An overview of vitamin D status and intake in Europe. Nutr. Bull. 39, 322–350. doi: 10.1111/nbu.12108
Stoffaneller R., Morse N. L. (2015). A review of dietary selenium intake and selenium status in Europe and the Middle East. Nutrients 7, 1494–1537. doi: 10.3390/nu7031494
Suliga P., Abie S. M., Egelandsdal B., Alvseike O., Johny A., Kathiresan P., et al. (2022). Beyond standard PSE testing: An exploratory study of bioimpedance as a marker for ham defects. Meat Sci. 194, 1–9. doi: 10.1016/j.meatsci.2022.108980
Sun H., Weaver C. M. (2021). Decreased iron intake parallels rising iron deficiency anemia and related mortality rates in the US Population. J. Nutr. 151, 1947–1955. doi: 10.1093/jn/nxab064
Svihus B. (2016). “Fôr og næringsbehov (In Norwegian),” in Fjørfeboka. Ed. Bagly M. F. (Fagbokforlaget, Bergen), 79–114.
The Norwegian Food Safety Authority. (2016-2017a). Matvaretabellen, Chicken, without skin, raw, code 140. Available online at: https://www.matvaretabellen.no (Accessed 07.08.2024).
The Norwegian Food Safety Authority. (2016-2017b). Matvaretabellen. Chicken, thigh (leg without drumstick), without skin, raw. Code 224b. Available online at: https://www.matvaretabellen.no (Accessed 07.08.2024).
The Norwegian Food Safety Authority. (2023a). Food Composition Table, Chicken, with skin, raw. 2020, code 140. Available online at: https://www.matvaretabellen.no (Accessed 07.08.2024).
The Norwegian Food Safety Authority. (2023b). Food Composition Table, Pork, striploin, raw. 2023 Code 332. Available online at: https://www.matvaretabellen.no (Accessed 07.08.2024).
The Norwegian Food Safety Authority. (2023c). Food Composition Table, Pork, minced meat, 9 % fat, raw. 2023. Code 332. Available online at: https://www.matvaretabellen.no (Accessed 07.08.2024).
Thiruvenkadan A. K., Prabakaran R., Panneerselvam S. (2019). Broiler breeding strategies over the decades: an overview. World's Poult. Sci. J. 67, 309–336. doi: 10.1017/s0043933911000328
Tixier-Boichard M. (2018). “Are there limits to selection in poultry? Alternative production and local breeds,” in LVI Symposium Científico de Avicultura, Asociación Española de Ciencia Avícola - American European Community Association – Wildlife Preservation Society of Australia. Spain: (WPSA-AECA) World’s Poultry Science Association La Asociación Española de Ciencia AvícolaValladolid.
Totland T. H., Melnæs B. K., Lundberg-Hallén N., Helland-Kigen K. M., Lund-Blix N. A., Myhre J. B., et al. (2012). National dietary survey among men and women aged 18-70 years 2010-11. (Norkost 3 landsomfattende kostholdsundersøkelse blant menn og kvinner i Norge i alderen 18–70 år, 2010–2011.). (Oslo, Norway: Norwegian Directorate of Health). pp. 1-70.
Tripkovic L., Wilson L. R., Lanham‐New S. A. (2017). Vitamin D2 vs. vitamin D3: They are not one and the same. Nutr. Bull. 42, 331–337. doi: 10.1111/nbu.12293
Trøan G. (2017). Effect of feeding rapeseed products differing in glucosinolate concentration on iodine concentration in cow milk. Philosophiae Doctor (PhD) Thesis No. 14, Norwegian University of Life Sciences (NMBU). Ås Norway.
Trøan G., Dahl L., Meltzer H. M., Abel M. H., Indahl U. G., Haug A., et al. (2015). A model to secure a stable iodine concentration in milk. Food Nutr. Res. 59, e29829. doi: 10.3402/fnr.v59.29829
Turck D., Bohn T., Castenmiller J., de Henauw S., Hirsch-Ernst K. I., Knutsen H. K., et al. (2023). Scientific opinion on the tolerable upper intake level for selenium. EFSA J 21, e07704. doi: 10.2903/j.efsa.2023.7704
Tybirk P., Sloth N. M., Blaabjerg K. (2023). Normer for næringsstoffer (In Danish) (Aarhus Denmark (In Danish: SEGES Innovation). Available online at: https://Svineproduksjon.dk (Accessed 10.01.2024).
Ueland M. (2022). Saccharina latissima in rations for dairy cows; effects on feed intake, milk yield and chemical composition of the milk. Master thesis. (Norwegian University of Life Sciences, Ås, Norway). pp. 1-62. Available at: https://hdl.handle.net/11250/3039209
USDA/FDC. (2003). FoodData Central. Beef ground FDC ID 174030 Pork ground PDC ID 167902. 2002 (beef) and 1992 (pork). Available online at: https://fdc.nal.usda.gov. (Accessed: Sept 11, 2024).
USDA/HHS. (1980). Dietary guidelines for Americans 1980. Available online at: https://www.dietaryguidelines.gov/about-dietary-guidelines. (Accessed: Sept 11, 2024).
USDA/HHS. (1990). Dietary guidelines for Americans 1990. Available online at: https://www.dietaryguidelines.gov/sites. (Accessed: Sept 11, 2024).
USDA/HHS. (2005). Dietary guidelines for Americans 2005. Available online at: https://www.dietaryguidelines.gov/about-dietary-guidelines. (Accessed: Sept 11, 2024).
USDA/HHS. (2020). Dietary guidelines for Americans 2020-2025. Available online at: https://www.dietaryguidelines.gov/about-dietary-guidelines. (Accessed: Sept 11, 2024).
van der Reijden O. L., Galetti V., Burki S., Zeder C., Krzystek A., Haldimann M., et al. (2019). Iodine bioavailability from cow milk: a randomized, crossover balance study in healthy iodine-replete adults. Am. J. Clin. Nutr. 110, 102–110. doi: 10.1093/ajcn/nqz092
van der Torre H. W., Van Dokkum W., Schaafsma G., Wedel M., Ockhuizen T. (1991). Effect of various levels of selenium in wheat and meat on blood Se status indices and on Se balance in Dutch men. Br. J. Nutr. 65, 69–80. doi: 10.1079/bjn19910067
van Son M., Enger E. G., Grove H., Ros-Freixedes R., Kent M. P., Lien S., et al. (2017). Genome-wide association study confirm major QTL for backfat fatty acid composition on SSC14 in Duroc pigs. BMC Genom. 18, e369. doi: 10.1186/s12864-017-3752-0
Vangen O. (1980). Studies on a two trait selection experiment in pigs. III. Correlated responses in daily feed intake, feed conversion and carcass traits. Acta Agric. Scand. 29, 337-345.
Volden H. (2011). NorFor – The Nordic feed evaluation system to ruminants (Wageningen: Wageningen Academic Press Publishers).
WCRF. (2015). Red meat and bowel cancer risk – how strong is the evidence? Available online at: https://www.wcrf.org/red-meat-and-bowel-cancer-risk-how-strong-is-the-evidence/ (Accessed 01.07. 2024).
WCRF. (2023). Diet activity and cancer. Available online at: https://www.wcrf.org/diet-activity-and-cancer/risk-factors/ (Accessed 10.01. 2024).
WCRF. (2024). Red and processed meat and cancer risk - Preventing cancer. Available online at: https://www.wcrf-uk.org/preventing-cancer/what-can-increase-your-risk-of-cancer/red-and-processed-meat-and-cancer-risk/ (Accessed 18.04. 2024).
Weisbjerg M. R., Rinne R., Spörndly A., Ekern O., Harstad M. (2010). The history of feed evaluation for ruminants, with special emphasis on the Nordic countries, in 1st Nordic Feed Sci. Conf, Uppsala,Sweden: Swedish University of Agricultural Sciences, 20-23 June 2010. Available at: https://jukuri.luke.fi/handle/10024/477010.
Wesolowski L. T., Semanchik P. L., White-Springer S. H. (2022). Beyond antioxidants: Selenium and skeletal muscle mitochondria. Front. Vet. Sci. 9, e1011159. doi: 10.3389/fvets.2022.1011159
Whist A. C., Østerås O., Solverød L. (2007). Staphylococcus aureus and Streptococcus dysgalactiae in Norwegian herds after introduction of selective dry cow therapy and teat dipping. J. Dairy Res. 74, 1–8. doi: 10.1017/S0022029906002135
WHO. (1986a). “Prevention and control of iodine deficiency disorders,” in Thirty-ninth world health assembly, Agenda item 29, , 16th May. (Geneva, Switserland: World Health Orgnization)
WHO. (1986b). Environmental Health Criteria 58: Selenium (Geneva: International Programme on Chemical Safety). Available at: http://www.inchem.org/.
WHO. (1990). Prev WHO., 1986b). Environmental Health Criteria 58: Selenium (Geneva: International Programme on Chemical Safety). Available at: http://www.inchem.org/. ention and control of iodine deficiency disorders. Forty-third world health assembly. Agenda item 17, 14th May.
WHO. (1996). Trace elements in human nutrition and health (Geneva Belgia). (Geneva, Switzerland: World Health Organization) (ed.).
WHO. (2002). World Health Report: Reducing risks, Promoting Healthy Life (Geneva, Switzerland: World Health Organization). Available at; World health report: 2002 (who.int).
WHO. (2007). Assessment of iodine deficiency disorders and monitoring their elimination: a guide for programme managers. 3rd ed (Geneva, Switzerland: World Health Organization Nutrition and Food Safety (NFS) Team).
WHO. (2009). Global health risks: mortality and burden of disease attributable to selected major risks (Geneva: World Health Organization).
WHO. (2014). World Health Organization. Guideline: fortification of foodgrade salt with iodine for the prevention and control of iodine deficiency disorders. (Geneva, Switzerland: World Health Organization).
WHO. (2022). European regional obesity report. Regional Office for Europe (Copenhagen: World Health Organization).
World Population Review. (2024). Pork consumption by country. Available online at: https://worldpopulationreview.com/ (Accessed 01.16.2024).
Yan X., Wang X., Zhang J., Ming Z., Zhang C., Ma P., et al. (2024). National trends in nine key minerals intake (quantity and source) among U.S. adults 1999 to March 2020. Nutr. J. 23, 1–13. doi: 10.1186/s12937-024-00950-4
Zbigniew M. (2002). Cereal products as a source of selenium in Polish food rations. (In Polish. Rocz. Panstw. Zakl. Hig. 53, 377–383.
Zhang X., Shi B., Spallholz J. E. (1993). The selenium content of selected meats, seafoods, and vegetables from Lubbock, Texas. Biol. Trace Elem. Res. 39, 161–169. doi: 10.1007/BF02783186
Zhang X., Xing T., Li J., Zhang L., Gao F. (2023). Mitochondrial dysfunction and calcium dyshomeostasis in the pectoralis major muscle of broiler chickens with wooden breast myopathy. Poult. Sci. 102, 1–11. doi: 10.1016/j.psj.2023.102872
Keywords: livestock, breeding, feeding, nutritional guidelines, meat, milk
Citation: Egelandsdal B, Grabez-Ågren V, Mydland LT, Haug A and Prestløkken E (2024) Animal breeding and feeding tools may close human nutrition gaps. Front. Anim. Sci. 5:1426044. doi: 10.3389/fanim.2024.1426044
Received: 30 April 2024; Accepted: 27 August 2024;
Published: 14 October 2024.
Edited by:
Jeff Wood, University of Bristol, United KingdomReviewed by:
Susan Fairweather-Tait, University of East Anglia, United KingdomDavide Lanzoni, University of Milan, Italy
Copyright © 2024 Egelandsdal, Grabez-Ågren, Mydland, Haug and Prestløkken. This is an open-access article distributed under the terms of the Creative Commons Attribution License (CC BY). The use, distribution or reproduction in other forums is permitted, provided the original author(s) and the copyright owner(s) are credited and that the original publication in this journal is cited, in accordance with accepted academic practice. No use, distribution or reproduction is permitted which does not comply with these terms.
*Correspondence: Egil Prestløkken, ZWdpbC5wcmVzdGxva2tlbkBubWJ1Lm5v
†These authors share senior authorship