- 1Department of Infectious Diseases and Public Health, Jockey Club College of Veterinary Medicine and Life Sciences, City University of Hong Kong, Hong Kong, Hong Kong SAR, China
- 2Departamento de Producción Animal y Pasturas, Facultad de Agronomía, Universidad de la República, Montevideo, Uruguay
- 3Centre de Recherche en Sciences Animales de Deschambault (CRSAD), Deschambault, QC, Canada
- 4Département des Sciences Animales, Université Laval, Québec, QC, Canada
- 5Agriculture and Agri-Food Canada, Sherbrooke, QC, Canada
Increasing dietary Met, Lys, and His supply without increasing the dietary protein content was reported to partially alleviate the productive and physiological impact of heat stress. Nevertheless, the metabolic pathways involved are yet to be identified. Thus, we aimed to explore the metabolic pathways associated with these positive effects and develop new metabolomics-based hypotheses. Twelve lactating Holstein cows (primiparous, n = 6; multiparous, n = 6; 42.2 ± 10.6 kg/d milk yield; 83 ± 28 days in milk) were enrolled in two 3×3 replicated Latin squares consisting of 14-day treatment periods: heat stress [HS; max. Temperature Humidity Index (THI) 84, 16.8% crude protein (CP), 1,741 g/d metabolizable protein (MP), 108 Lys, 33 Met, and 37 His (g/d)], pair feeding in thermo-neutrality (TN; max. THI 64, same diet as HS), and HS with increased Lys, Met and His supply [HS+AA; max. THI 84; 17.0% CP, 1,730 g/d MP, 179 Lys, 58 Met, and 45 His (g/d)]. Blood plasma and milk were sampled on day 14 for metabolomics profiling. Several amino acids (AA) and derivatives differed between the treatments. Plasma and milk Met, Val, Trp and α-amino adipic acid concentrations were highest in HS+AA (false discovery rate-P (FDR) < 0.05). Moreover, only plasma Lys and milk His were highest in HS+AA (FDR < 0.05). Some phosphatidylcholines (PC) and diglycerides had lower concentrations in HS than TN (FDR < 0.05), while HS+AA had similar concentrations as TN. The pathway enrichment analysis revealed that the AA-related pathways were more significantly affected in multiparous than in primiparous cows. Our results suggest that increased supply of Met stimulated PC synthesis in HS+AA to similar concentrations as in TN. Increased Lys supply likely elevated the oxidation rate of Lys and downregulated the catabolism of other essential AA (EAA) such as Val and Trp, stimulating milk protein synthesis. No clear associations were found related to His availability. In conclusion, partial amelioration of productive and physiological effects of heat stress associated with increased dietary Met and Lys supply were likely explained by stimulated PC synthesis and increased plasma and milk concentrations of other EEA.
1 Introduction
The prevalence of heat-stressed conditions in dairy cows is projected to become more frequent and severe due to global warming (Thornton et al., 2021). Advancing our knowledge of the underlying physiology is important for designing nutritional strategies targeting the alleviation of the negative effects of heat stress (Wheelock et al., 2010; Ruiz-González et al., 2023). Studies showed that the drop in dry matter intake (DMI) can only explain up to 50% of the decrease in milk yield, thus the negative metabolic effects caused by the heat stress could be partially responsible for the remaining drop in production performance (Rhoads et al., 2009; Wheelock et al., 2010).
Unlike the metabolic and endocrine regulation during the transition period where the DMI deficiency and negative energy balance are associated with decreased insulin sensitivity and upregulated lipolysis, the DMI drop during heat stress is linked with downregulated lipolysis and decreased glucose use in the mammary gland (as reviewed by Baumgard and Rhoads, 2013). These metabolic changes support the increased energy demand of the activated immune system. The immune activation is mainly due to the increased lipopolysaccharides (LPS) load as a consequence of local hypoxia and impaired integrity of the intestinal epithelial barrier (‘leaky gut’) (Lambert et al., 2002; Pearce et al., 2013). In response, muscle protein catabolism is enhanced supporting the increased hepatic and intestinal AA utilization, while AA availability for the mammary gland decreases (Rhoads et al., 2009; Kvidera et al., 2017a). These metabolic effects triggered by heat stress are parity-dependent as multiparous cows usually show more pronounced changes than primiparous cows (Bernabucci et al., 2014; Chen et al., 2022).
One of the main metabolic responses to heat stress is the enhanced labile protein catabolism, however, an increased supply of metabolizable protein (MP) was found to be unsuccessful in modulating muscle catabolism (Kaufman et al., 2018). Under thermoneutral conditions, dietary supplementation of limiting AA such as Met, Lys and His improved N use efficiency in low-protein diets concomitant with unchanged milk and milk protein synthesis (Lee et al., 2012; Räisänen et al., 2021). Under heat-stress conditions, milk yield and oxidative status of dairy cows was improved by increased Met supply, rather than MP (Mesgaran et al., 2022). In vitro studies on hyperthermic mammary gland epithelial cells indicated that increased Met supply can lead to a higher synthesis of the antioxidants taurine and glutathione and stimulate protein synthesis through the direct activation of the mechanistic target of rapamycin (mTOR) pathway or indirectly by increased branched-chain AA (BCAA) availability (Dong et al., 2018; Lopreiato et al., 2020; Coleman et al., 2022). However, the improved productive performance of heat-stressed cows after dietary Met supplementation was not consistent across the few reported studies (Pate et al., 2020; Mesgaran et al., 2022) and the mechanisms and pathways remain to be understood (Lopreiato et al., 2020; Mesgaran et al., 2022). Preliminary results of our team showed that increasing Met, Lys and His supply during heat stress alleviated the rise in body temperature and inflammation status, and improved milk protein content of high-yielding dairy cows (Ruiz-González et al., 2022a). Increased Met and Lys supply effects have been widely studied in other physiological circumstances (e.g. during the transition period). However, their potential to counteract heat stress effects remains to be comprehensively evaluated in dairy cows. Thus, the metabolomics analysis of blood plasma and milk could uncover the possible metabolic pathways by which increased Met, Lys and His supply contribute to heat stress alleviation.
We hypothesized that these alleviating effects of increased dietary Met, Lys and His availability are associated with increased antioxidant synthesis (e.g. taurine) and decreased AA catabolism, contributing to increased availability of other EAA during heat stress. This could be reflected in the plasma and milk metabolome profiles, with more prominent changes in multiparous cows. Hence, the aims of this study were: 1) to elucidate the potential alleviating effects of increased dietary Met, Lys and His supply on the metabolomic changes triggered by heat stress with particular focus to AA-related metabolism, and 2) to explore the possible interactions between the dietary strategy and parity on the metabolomic response to heat stress.
2 Material and methods
2.1 Experimental design
This experiment was carried out between October and December 2020 at the Dairy Research Farm of the Centre de Recherche en Science Animales de Deschambault (CRSAD; Deschambault, QC, Canada). All animals were owned by CRSAD. The enrolment of the animals in this study as well as the animal care procedures and management practices were approved by the CRSAD animal care committee (approval number 2019-BL-386) in accordance with the Canadian Council on Animal Care guidelines for the use of Farms Animals (CCAC, 1993).
Twelve lactating Holstein cows were allocated to two plots based on their parity: 1) primiparous (n = 6; 29.5 ± 1.2 kg milk/d; 95.2 ± 16.2 days in milk; mean ± SD), and 2) multiparous (n = 6; 40.3 ± 5.5 kg milk/d; 86.8 ± 10.2 days in milk; 3.5 ± 1.6 parity). Treatment subplots were arranged in a replicated 3 × 3 Latin square design balanced for residual effects with a 14-d period of treatment and 7-d period of washout between treatments according to our previous study (Ruiz-González et al., 2023). The number of replicates were comparable with previously reported studies (Rhoads et al., 2009; Kassube et al., 2017). Within each square, cows were submitted to three different treatments: 1) heat stress (HS; 16.8% CP; maximal temperature humidity index (THI): 84) with a diet balanced to supply 33 Met, 108 Lys, 37 His, and 1,741 MP g/d at expected DMI nadir (d7–14; 15.5 kg/d) according to NRC (NRC, 2001), 2) pair feeding in thermoneutrality (TN; same diet as the HS treatment; maximal THI = 64), and 3) heat stress with a diet of a similar CP (17%) and MP (1,730 g/d) content but balanced to supply higher levels of Met (58 g/d, +77%), Lys (179 g/d, +65%) and His (45 g/d, +22%) based on expected DMI reductions (HS+AA; maximal THI = 84) as previously reported (Ruiz-González et al., 2023). Heat stress was induced using a cyclic pattern (THI 72–84) and resulted in decreased DMI (34%) and milk yield (40%) from day 0 to day 14. The diet supplied during HS and TN treatments was formulated to meet the predicted energy and nutrient requirements (NRC, 2001) in a thermoneutral environment and fed at previously observed DMI and consisted of corn silage, alfalfa haylage, ground corn, soybean meal, corn gluten meal and a mineral and vitamin premix. In the HS+AA treatment, the soybean meal and corn gluten meal were substituted by canola meal and blood meal, to increase His supply; in addition, cows received a supplement of rumen-protected Met (Smartamine®-L; Adisseo, Alpharetta, GA) and Lys (Ajipro®-L; Ajinomoto, Chicago, IL) adjusted daily to supply 8.5 g + 4.2 g/kg reduced DMI and 27.85 g/kg reduced DMI, respectively. The 2 diets were prepared as TMR and the cows were individually fed the TMR twice daily (0900 and 1400 h). The rumen-protected AA supplements were mixed with 500 g (fresh basis) of ground corn to ensure complete intake immediately before the morning feeding (0900 h). The same amount of ground corn was provided to the other treatment in order to control for this effect. Drinking water was available at all times. Removal from heat chambers and exclusion of animals from the trial was considered if DMI reductions were greater than 50% for a period of 48 h. No animal was removed from the experiment based on these criteria.
Blood samples were taken at the end of each experimental period (day 14) before the morning feeding by coccygeal venipuncture using heparinized tubes, immediately placed on ice, and centrifuged within 20 min at 1,500 × g for 20 min. The plasma was harvested and stored at -80°C until the metabolomics analysis. Whole milk samples were taken at the morning milking on day 14 in empty tubes with no chemical preservative and immediately stored at -80°C until the metabolomics analysis.
2.2 Metabolomics analysis
The targeted mass spectrometry-based metabolomics profiling of the plasma and milk samples were carried out at The Metabolomics Innovation Centre, Alberta, Canada. Eleven classes of metabolites, including AA, biogenic amines, monosaccharides, acylcarnitines (AC), diglycerides (DG), triglycerides (TG), lysophosphatidylcholines (LysoPC), phosphatidylcholines (PC), sphingomyelins (SM), ceramides (Cer), and cholesteryl esters (CE), were quantified using the Absolute IDQ p400 Kit (Biocrates Life Sciences AG, Innsbruck, Austria). The chemometric protocol descriptions were previously reported (Foroutan et al., 2019). In brief, plasma and milk samples were thawed, vortexed, and centrifuged at 13,000 × g. Ten µL of samples were loaded onto the filter on the upper 96-well plate and dried under nitrogen flow. Amino acids were derivatized with 5% phenyl-isothiocyanate. Metabolites were extracted with methanol containing 5 mM ammonium acetate, and the extracts were centrifuged into the lower 96-deep well plate. The extracts were split for liquid chromatography-high resolution mass spectrometry to detect the amino acids and biogenic amines, and direct flow injection-mass spectrometry analyses for detecting the remainder of the targeted metabolite classes. The metabolomics assay was performed on a Thermo Scientific QExactive HF OrbiTrap mass spectrometer (Thermo Scientific, Mississauga, Canada). The detection of each metabolite relied on measuring the exact mass of molecular ions or adducts without further fragmentation. Isotope-labeled internal standards and other internal standards were integrated into the kit’s 96-well plate filter for metabolite quantification. The entire workflow was monitored and controlled with the Biocrates MetIDQ software.
2.3 Statistical analysis
Prior to statistical analysis, the variables (metabolites) were filtered within each metabolomic dataset (plasma and milk separately) according to the limit of detection (LOD). Only those metabolites detected above LOD in at least 80% of the samples were retained in the final dataset. No missing values were observed. The metabolomics data was adjusted for the effect of the Latin-square design and sequence prior to any statistical analysis according to suggested workflow (Zhao et al., 2019). This was done by a linear model using the lm package in R software v3.6.3 (R Core Team, 2020).
All statistical analyses were performed on the MetaboAnalyst v5 platform (https://www.metaboanalyst.ca/). Data were log transformed and scaled using the Pareto’s algorithm (Chong et al., 2019) before performing the statistical analysis, which included multivariate, univariate and metabolic pathway analyses. Multivariate analysis based on partial-least squares discriminant analysis (PLS-DA) was performed comparing the treatments and the parity class separately. The cross validation of the model was performed on the basis of the quality assessment statistic (Q2), while the goodness of fit was assessed by the R-square (R2Y) value. The model refinement was done by re-running the model on the base of those metabolites with variable in projection (VIP) value > 1.0 until Q2 did not improve more than 0.0975 between two consecutive versions of the model.
The effects of the treatment (TN, HS, HS+AA), parity (P, M) and their interaction were assessed by ANOVA. ANOVA’s raw P-values were adjusted by the Benjamini-Hochberg false discovery rate (FDR) method according to Vinaixa et al. (2012), and significant effects were declared at FDR ≤ 0.05 and tendencies at 0.05 < FDR ≤ 0.10. If a significant interaction effect was found by the ANOVA, post hoc multiple comparisons were performed by Tukey’s honestly significance difference test, and the level of significance was declared at P ≤ 0.05.
Finally, metabolic pathways were evaluated by quantitative enrichment analysis based on the Kyoto Encyclopedia of Genes and Genomes (KEGG) and the Globaltest algorithm, which calculates the association between the metabolite sets and the phenotype (Chong et al., 2019). As this analysis is based on pairwise comparisons, it was performed considering HS vs TN, and HS vs HS+AA, both performed independently for multiparous and primiparous cows. Significant enrichment of a given metabolic pathway was considered at FDR ≤ 0.05, and only metabolic pathways with at least two metabolites measured in the dataset were considered for discussion.
3 Results
The metabolomic profiling led to the effective quantification of 212 and 164 out of 404 possible metabolites in plasma and milk, respectively. A list of the measured compounds and their abbreviations is available in Supplementary Table S1.
3.1 Plasma metabolomics
Multivariate analysis revealed valid (Q2 > 0) PLS-DA models for the treatment class (TN, HS, HS+AA) with the best model retaining the 3 first components and showing moderate predictive ability (Q2 = 0.50) and high fitness (R2 = 0.86). The top 15 metabolites with the highest VIP scores included α-aminoadipic acid, Met, Gly, and Lys, among others (Figure 1). The parity class also yielded a valid PLS-DA model (Q2 > 0) for the plasma metabolomics data. The best model retained 3 components and had low predictive ability (Q2 = 0.29) but high fitness (R2 = 0.89).
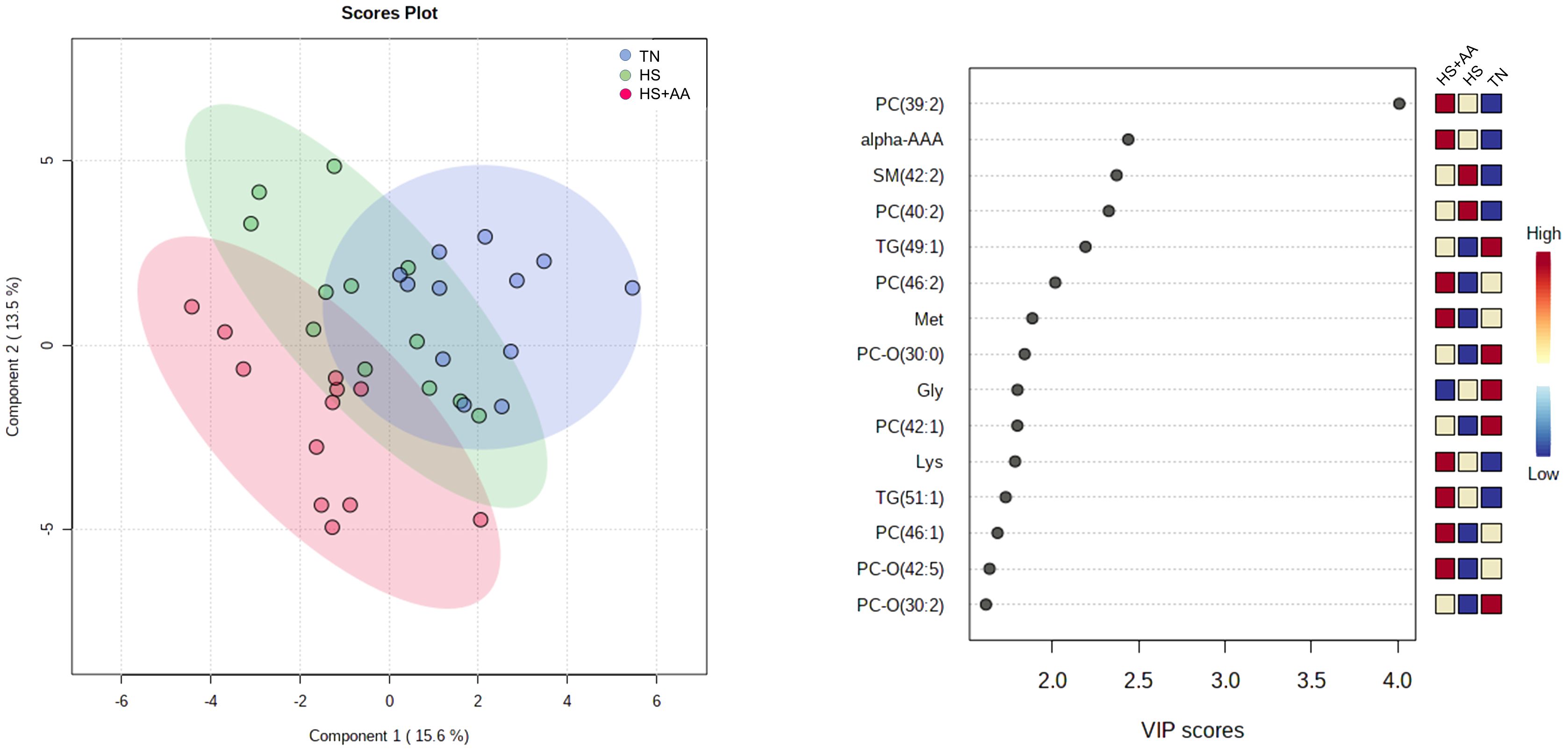
Figure 1 Partial least squares - discriminant analysis (PLS-DA) scores plot for the treatment class (thermoneutral, TN, blue; heat-stressed, HS, green; heat-stressed with high Met, Lys and His supply, HS+AA, red) based on the plasma metabolomics profiles. The panel on the right lists the 15 most important discriminating metabolites based on variable in projection (VIP) scores.
According to the ANOVA, 15 plasma metabolites differed (FDR ≤ 0.048 in all cases) between treatments. Most of them (10 out of 15) were AA or AA-related compounds (Table 1). Among these, Met, Lys, Val, Phe, Trp, α-aminoadipic acid and sarcosine had the greatest concentrations in the HS+AA cows, while the TN and HS treatments had lower metabolite concentrations (Table 1). Gly, Cit, and trans-4-hydroxyproline had greater concentrations in TN, compared with HS and HS+AA. The remaining 5 metabolites comprised lipids and complex lipids which all had the greatest concentrations in the TN. PC 40:9, DG 39:0 had intermediate concentrations in HS+AA and were lowest in HS, compared with TN, while PC 40:8, PC 44:3, and CE 20:5 had similar concentrations in HS+AA and HS and still lower than TN. Regarding the parity effect, multiparous cows had lower plasma concentrations of α-aminoadipic acid (FDR < 0.001) and citrulline (FDR = 0.048) than primiparous (Table 1). None of the plasma metabolites were affected by the interaction between treatment and parity. Despite the lack of an FDR-significant effect, it is still worth noting that plasma His concentrations had a raw-P = 0.038 for the treatment effect, as these was higher in HS+AA (60.0 ± 2.13 mM, mean ± SEM) than in TN (50.6 ± 3.47 mM) and HS (52.8 ± 1.3 mM). The complete set of results of plasma metabolites are listed in Supplementary Table S2.
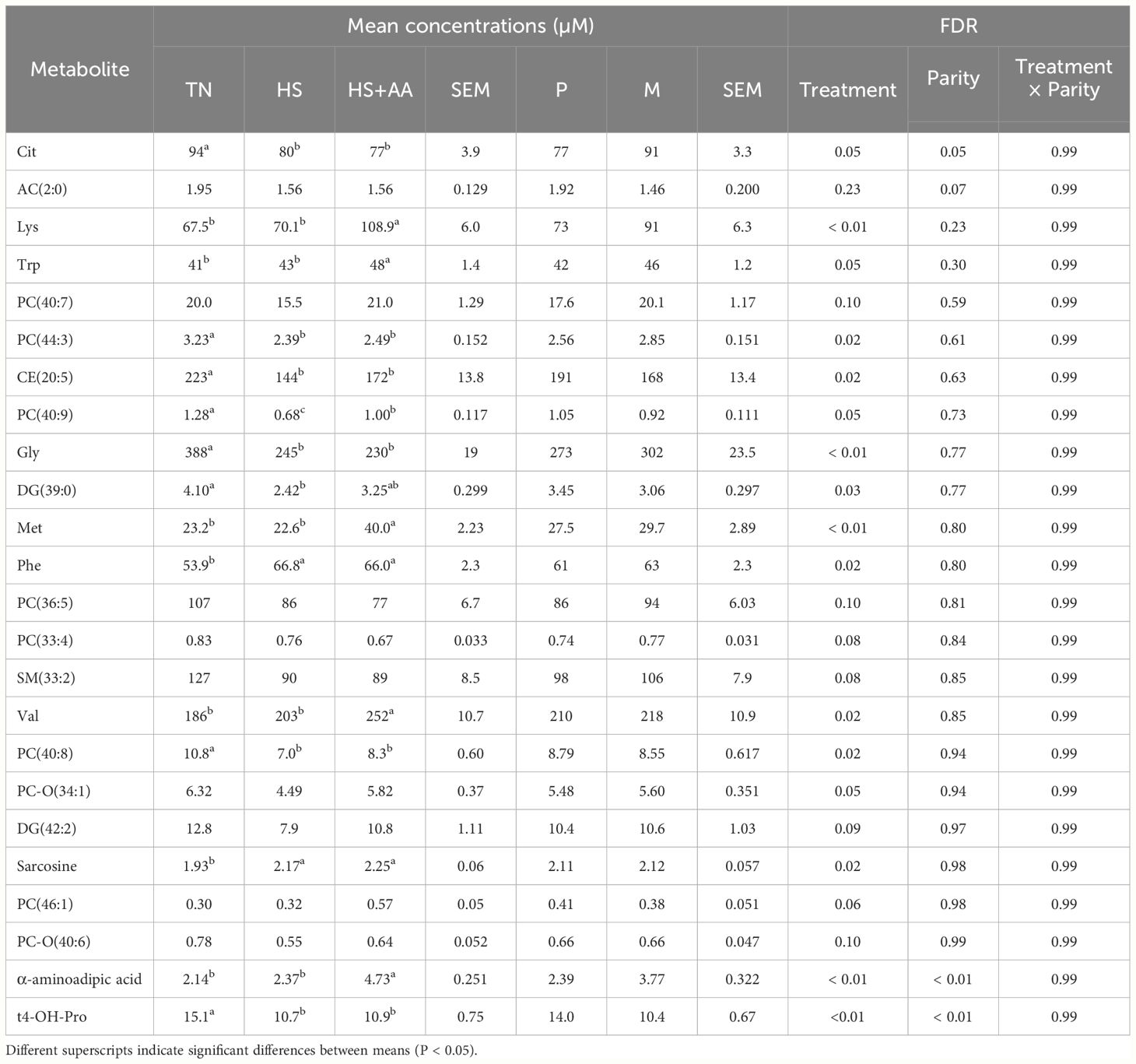
Table 1 Metabolites differing (FDR < 0.05) between treatment (thermoneutral, TN; heat-stressed, HS; increased dietary Met, Lys and His supply, HS+AA), parity (primiparous, P; multiparous, M) and their interactions in plasma upon the end (day 14) of the experimental treatments.
The metabolic pathway enrichment analysis revealed differences in the heat stress response according to the parity. When comparing HS vs TN, several pathways differed (FDR < 0.05) or tended to differ (FDR < 0.10) in multiparous but not in primiparous cows (Table 2). Among these pathways, glutathione metabolism and primary bile acid biosynthesis were associated with lower plasma concentrations of several compounds (e.g. Gly, Glu, ornithine, taurine) for HS than TN (Figure 2). In addition, the His metabolism and β-alanine metabolism were found to be altered due to lower plasma concentrations of Glu and carnosine and greater concentrations of His and Asp in HS, compared with TN (Figure 2). Finally, tryptophan metabolism was affected by numerically higher concentration of Trp and significantly lower concentrations of serotonin and kynurenine in HS, compared with TN. Further, phenylalanine metabolism and phenylalanine, tyrosine, and tryptophan biosynthesis were affected due to greater concentrations of Phe and Tyr in HS than in TN (Figure 2).
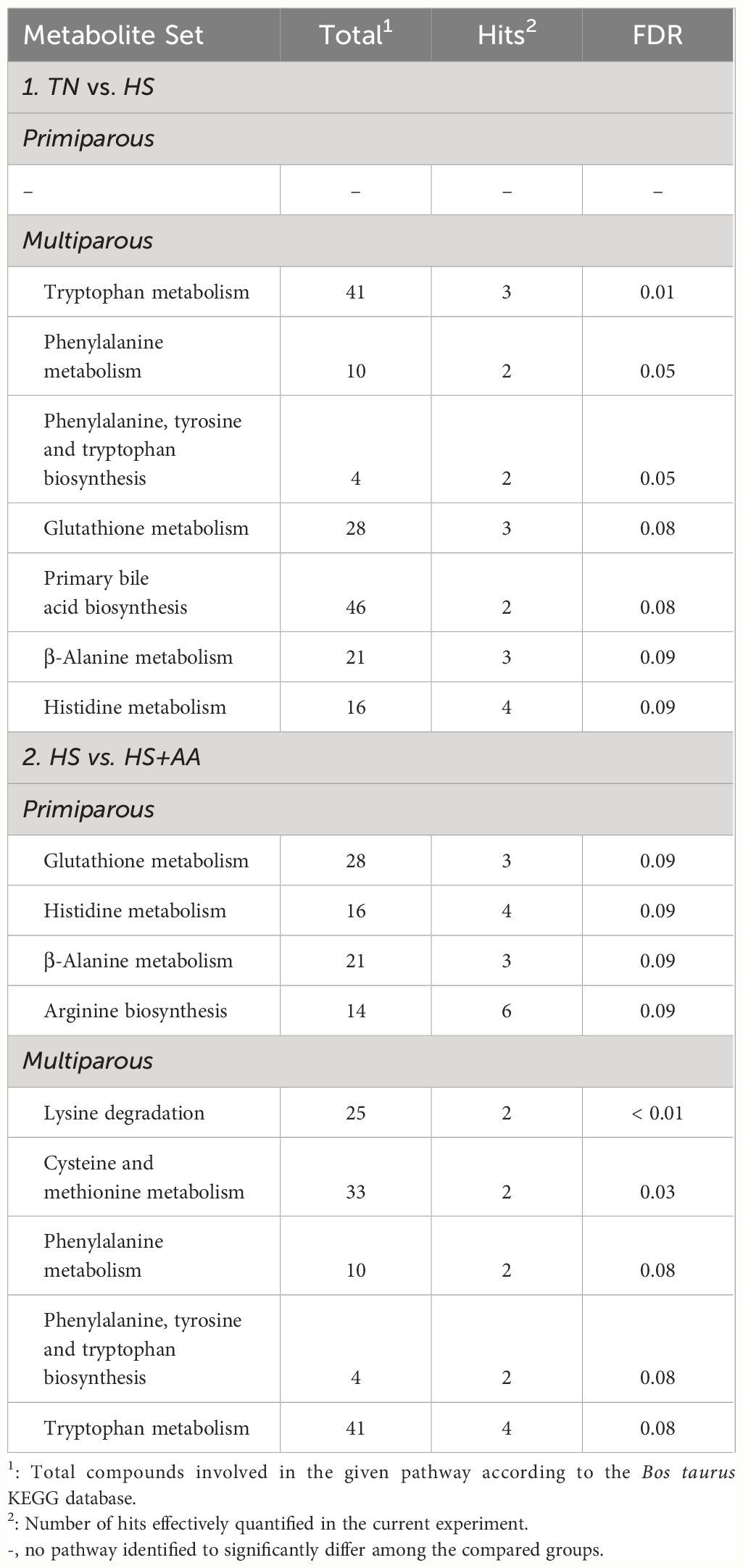
Table 2 Metabolic pathways differing between heat-stressed (HS) vs. thermoneutral (TN), and HS vs. HS with increased dietary Met, Lys and His supply (HS+AA) treatment when compared within each parity class based on plasma metabolomics.
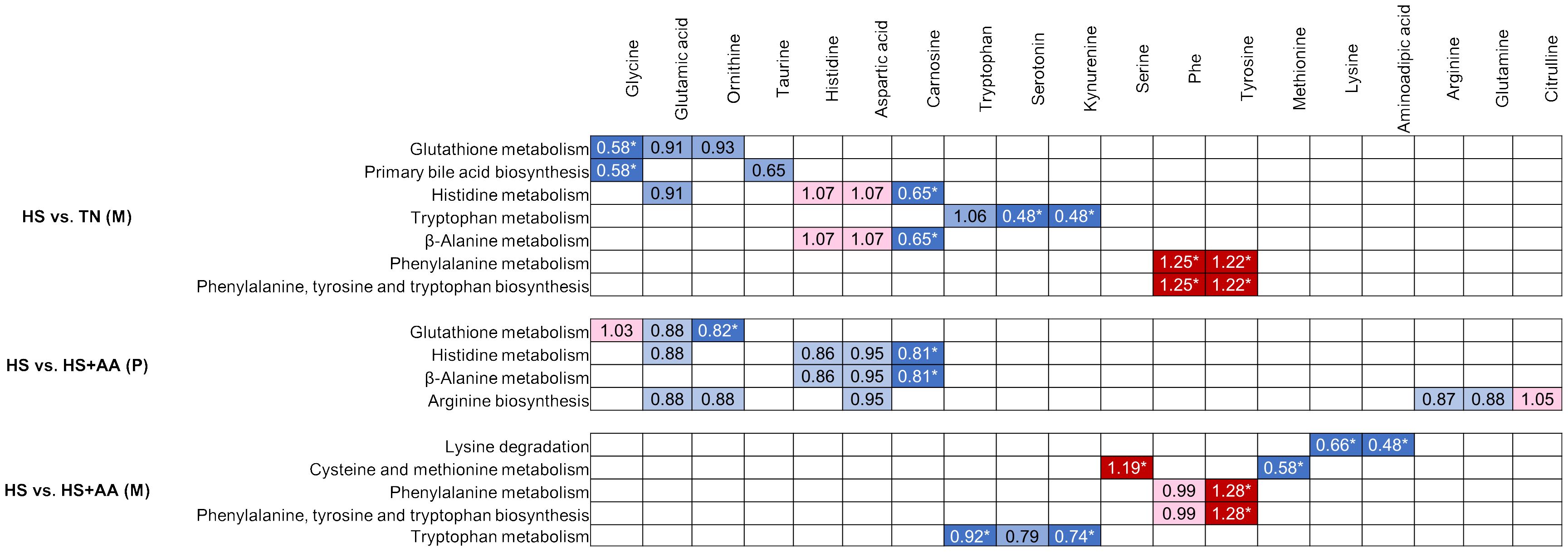
Figure 2 Metabolic pathways differing (FDR ≤ 0.10) between treatments (thermoneutral, TN; heat stress, HS; heat-stressed with high Met, Lys and His supply, HS+AA) within each parity class (primiparous, P; multiparous, M) according to the plasma metabolomic data. The measured metabolites in the current study belonging to each pathway are depicted by colored cells, with higher and lower concentrations for HS vs. TN or HS vs. HS+AA indicated by red and blue tones, respectively. Significant differences (P < 0.05) among the compared pairs are depicted by darker color and *.
When comparing HS vs HS+AA, the pathway analysis also yielded parity-dependent differences. Glutathione metabolism, histidine metabolism, β-alanine metabolism and arginine biosynthesis differed in primiparous cows, while lysine degradation, cysteine and methionine metabolism, tryptophan metabolism, phenylalanine metabolism, and phenylalanine, tyrosine and tryptophan metabolism biosynthesis differed in the multiparous cows (Table 2). In the primiparous cows, most of the affected compounds had a lower concentration in HS than HS+AA (Figure 2). In the multiparous cows, the compounds involved in the lysine degradation, cysteine and methionine metabolism, and tryptophan metabolism (e.g. Trp, serotonin, kynurenine, Met, Lys and α-aminoadipic acid) had lower concentrations in HS than HS+AA. However, the metabolites involved in phenylalanine metabolism, and phenylalanine, tyrosine and tryptophan biosynthesis (e.g. Phe, Tyr) had higher concentrations in HS than HS+AA (Figure 2).
3.2 Milk metabolomics
Multivariate analysis of the milk metabolome yielded a valid PLS-DA model based on the first 2 components for the treatment discrimination, showing low predictive ability (Q2 = 0.27) but high fitness (R2 = 0.84). No valid model was obtained for the parity discrimination (Q2 < 0) (Supplementary Figure S1).
According to the ANOVA, 21 metabolites differed (FDR ≤ 0.048 in all cases) between treatments (Table 3). These metabolites included acetyl-carnitine (C2:0) which had a lower concentration in HS than in TN and HS+AA, and butyryl-carnitine (C4:0) which had a higher concentration in HS than in TN, with intermediate concentration in HS+AA. Additionally, 8 metabolites belonging to the AA metabolism differed between treatments. Among these, Met, His, Trp, Val and α-aminoadipic acid had the highest concentrations in HS+AA with lower concentrations in TN and HS. Gly, Pro and taurine had higher concentrations in TN than HS and HS+AA. Most of the complex lipids differing between treatments included PC (e.g. PC 32:1, 44:3, 44:5, 32:0, 36:2, 36:1) which were lower in HS than in TN and HS+AA. Additionally, 7 milk metabolites differed (FDR < 0.05) according to parity. Among these, carnitine and 2 acyl-carnitines (C2:0, C5:0-OH), SM 39:1 and PC 46:2 had greater concentrations in multiparous than primiparous, while Lys was lower in multiparous than primiparous (Table 3). The complete set of milk metabolites are listed in Supplementary Table S3.
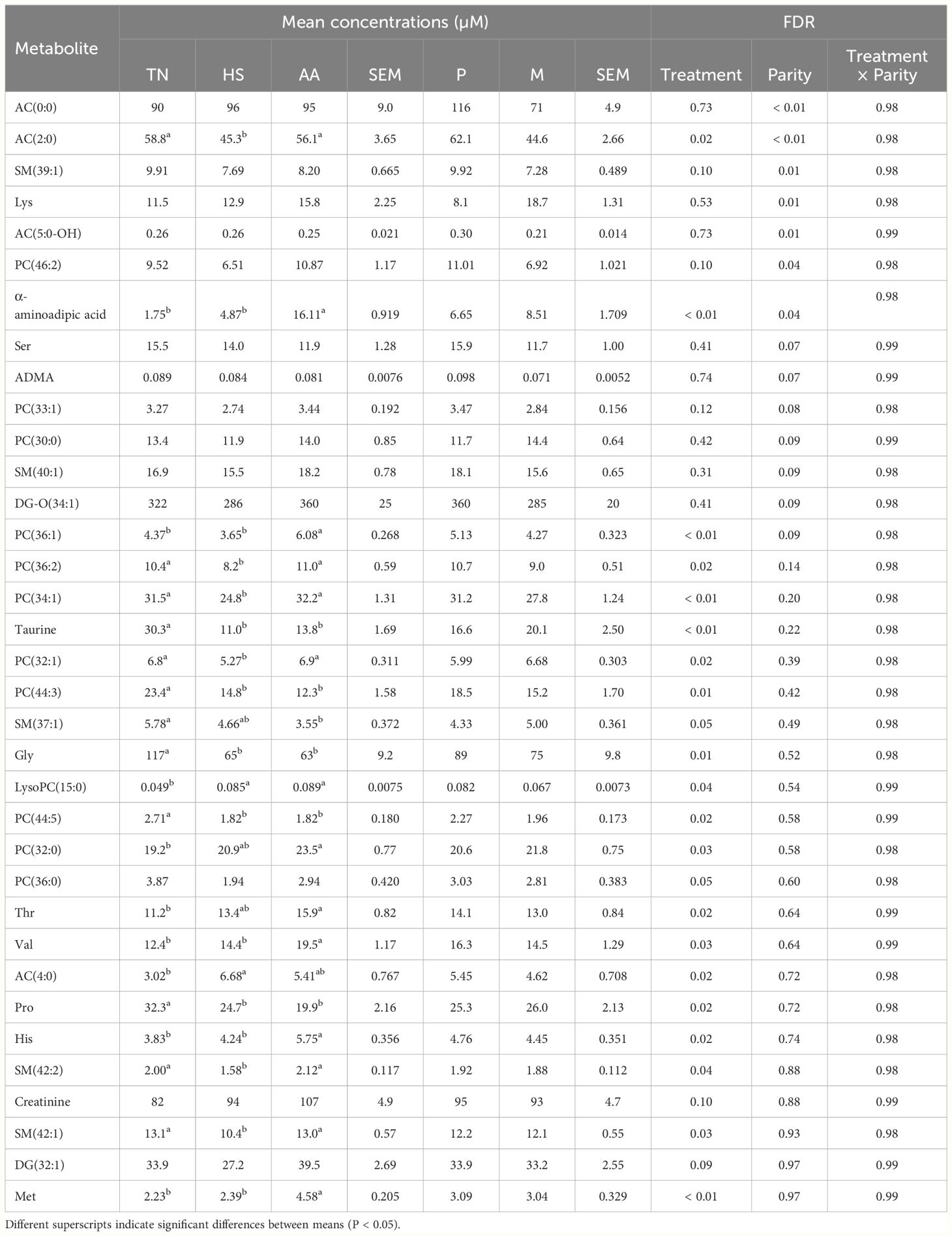
Table 3 Metabolites differing (FDR P < 0.05) between treatment (thermoneutral, TN; heat-stressed, HS; increased dietary Met, Lys and His supply, HS+AA), parity (primiparous, P; multiparous, M) and their interactions in milk upon the end (day 14) of the experimental treatments.
The milk metabolomics pathway analysis identified few pathways to be significantly different between treatments. The primary bile biosynthesis was identified as the only pathway differing (P < 0.01) between HS and TN for both parities, reflecting the lower milk concentrations of taurine and Gly in the HS cows. When comparing HS vs HS+AA, no pathways differed in the primiparous cows. In the multiparous cows, cysteine and methionine metabolism and lysine degradation differed (P ≤ 0.02) due to the lower milk concentrations of α-aminoadipic acid, carnitine, and Lys in HS compared with HS+AA.
4 Discussion
Our plasma and milk metabolomics data suggested that the heat stress-related depletion of the circulating PC pool was reverted by the elevated dietary Met supply. This can be explained by enhanced one-carbon metabolism in response to increased Met availability. In addition, the improved milk protein content in the HS+AA treatment was likely modulated by the increased Val and Trp availability as a consequence of the additional Lys supply. The main findings were linked to increased dietary Met or Lys supply, rather than His, as the increase in His availability was minor relative to the increase in the availability of Met and Lys. Interpretive schemes integrating the main results related to Met and Lys availability are presented in Figures 3, 4, respectively.
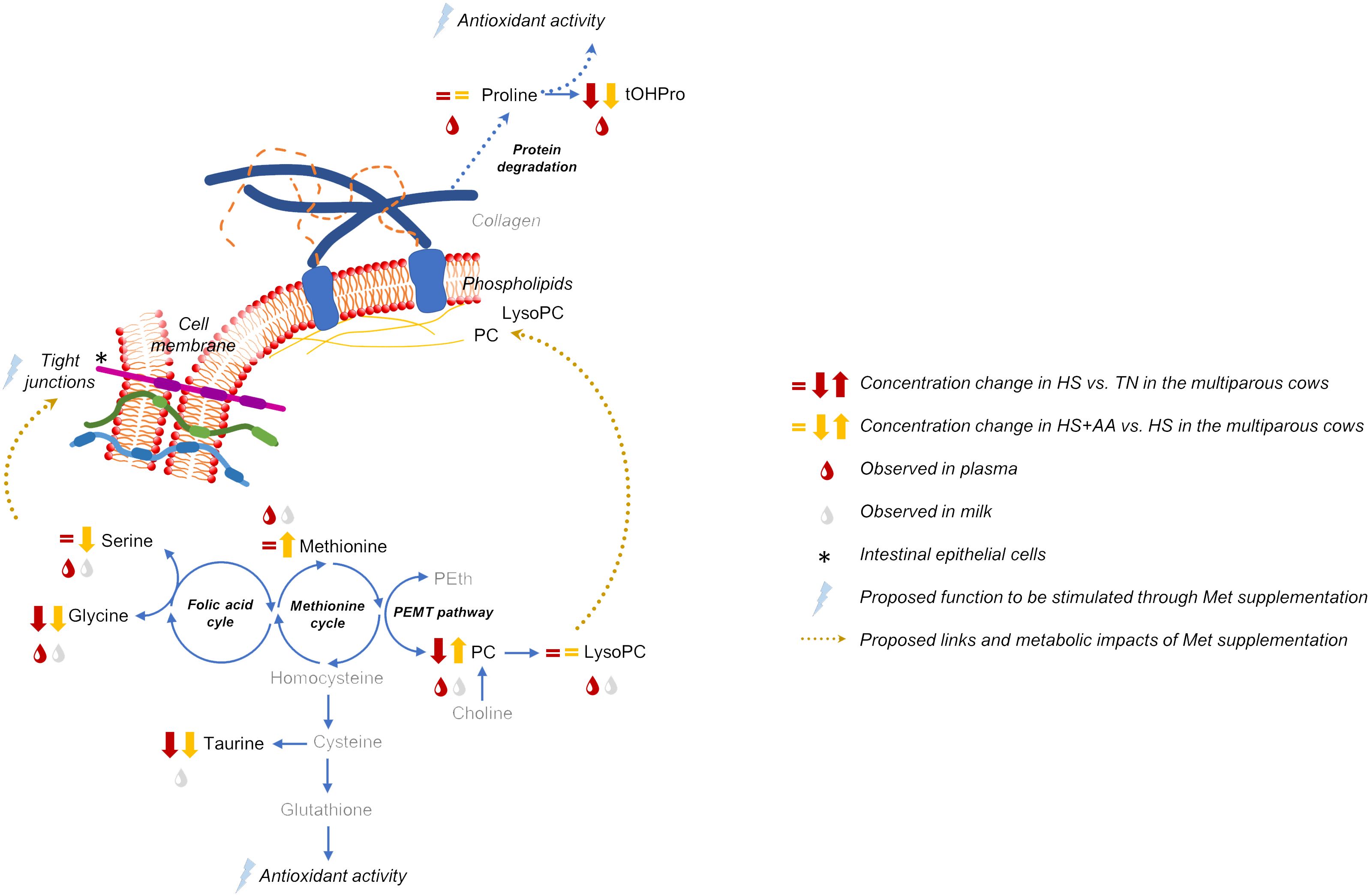
Figure 3 Interpretive metabolic map integrating the main results related to methionine metabolism, with particular regard to the multiparous cows and their response to increased dietary Met supply during heat stress. Metabolite changes depicted here correspond to pairwise comparisons of the pathway enrichment analysis. Metabolites effectively measured in our study are indicated in black, while the compounds in grey were not quantified herein. tOHPro, trans-hydroxyproline; PEth, phosphatidylethanolamine; PEMT, phosphatidylethanolamine N-methyltransferase; PC, phosphatidylcholine; LysoPC, lysophosphatidylcholine.
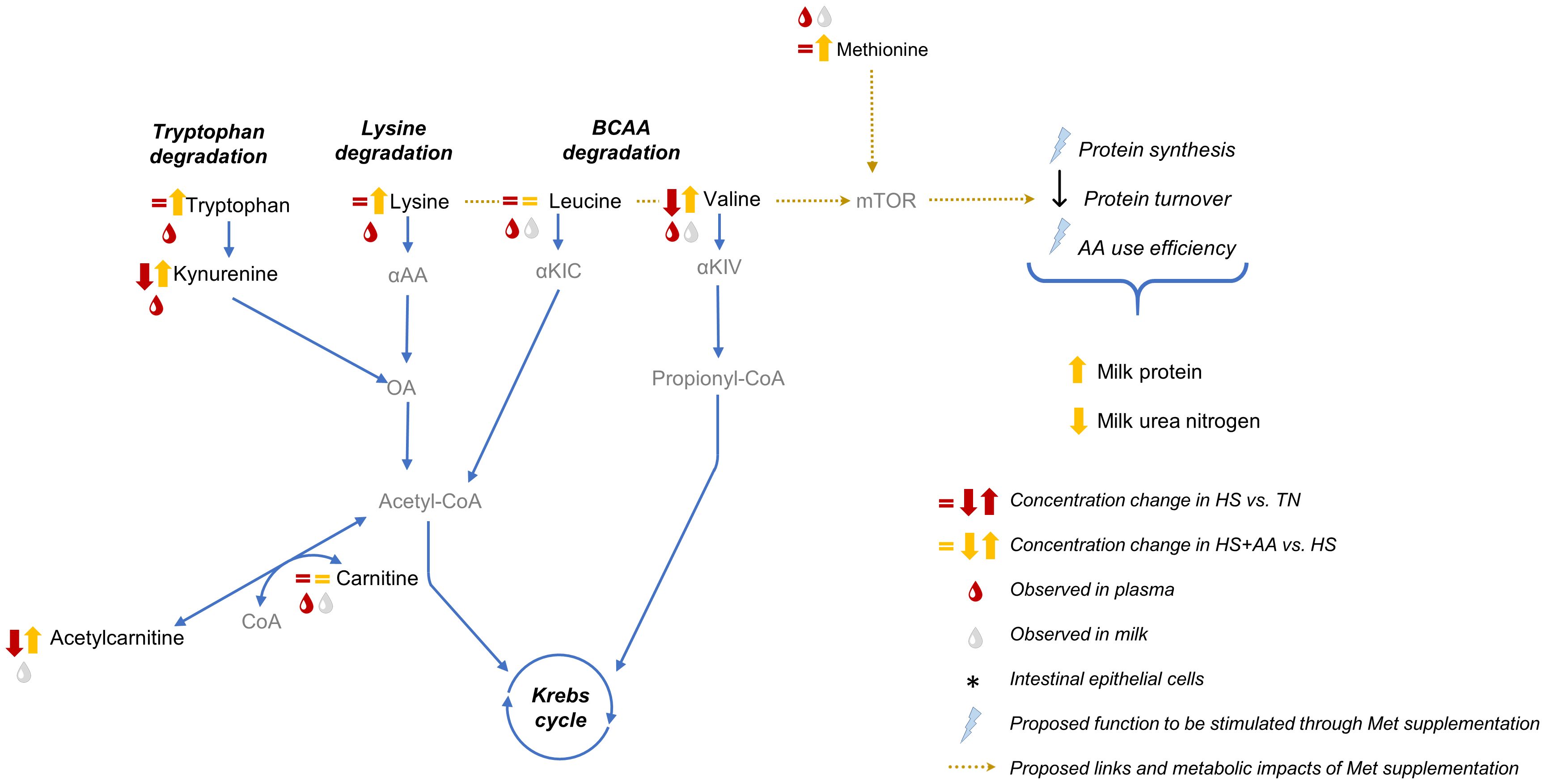
Figure 4 Interpretive metabolic map of Lys metabolism with regard to the multiparous cows’ response to increased Lys supply during heat stress. Altered metabolite concentrations correspond to pairwise comparisons of the pathway enrichment analysis. Metabolites effectively measured in our study are indicated in black, while the compounds in grey were not quantified herein. αAA. α-aminoadipic acid; αKIC. α-ketoisocaproic acid; αKIV, α-ketoisovaleric acid; OA, 2-oxoadipic acid; mTOR, mechanistic target of rapamycin pathway.
In line with their EAA character, i.e. dietary-dependent availability (Schwab and Broderick, 2017), increased dietary supply of Met, Lys and His in HS+AA was reflected by increased plasma and milk concentrations of these AA. Indeed, while Met and Lys supply increased by 77% and 65%, respectively, His was only increased by 22% which was reflected by a less pronounced increase of plasma His in HS+AA, compared with TN or HS treatments. Despite the lack of a significant FDR, plasma His had a significant raw-P value for the treatment effect, since it was higher in HS+AA than in the other two treatments.
The general trend of decreased plasma concentrations of PC, and to a lesser extent some of the LysoPC in HS were likely associated with systemic inflammation as previously proposed (Hung et al., 2012; Liu et al., 2017). While the concentrations of some LysoPC were numerically lower in HS than TN, due to the lack of significant FRD values, the following interpretations aim to propose hypotheses in an exploratory approach for further testing. Phosphatidylcholines are precursors for the synthesis of LysoPC, which play signaling roles at the cellular level and participate in inflammatory response in mammals, including bovines (del Bas et al., 2016; Humer et al., 2018; Rico et al., 2021). Thus, its decreased concentrations in heat-stressed cows were likely related to the systemic inflammation (Kvidera et al., 2017b). In fact, decreased plasma LysoPC concentrations were found after LPS challenge in bovines under thermoneutral conditions (Humer et al., 2018; Javaid et al., 2022). According to the milk lipidomics-based findings of Liu et al. (2017), the decrease in LysoPC should be a structural metabolic shift in response to heat stress as heat stress-sensitive cows have been observed to suffer greater changes in LysoPC than tolerant cows. In the current experiment, PC rather than LysoPC showed more evident changes during heat stress, thus PC were likely further utilized to sustain LysoPC synthesis. In addition, the PC: phosphatidylethanolamine balance plays an important role in membrane response and thermal sensing, which are highly conserved functions across species (as reviewed by Balogh et al., 2013). In summary, the decreased plasma and milk PC concentrations likely indicated increased cell membrane fluidity during HS.
However, increased Met availability in HS+AA appeared to have improved plasma and milk PC concentrations. Six out of 9 plasma PCs, and 4 out of 8 milk PCs that decreased or tended to decrease in HS vs. TN (FDR ≤ 0.10 for the treatment effect) were improved in HS+AA either to similar concentrations as in TN or intermediate concentrations between HS and TN. Moreover, when considering the raw-P values ≤ 0.10, most of the plasma (33 out of 40) and milk (13 out of 17) PCs showed negative fold change in HS compared either to TN or HS+AA (Supplementary Figure S2; Supplementary Tables S2, S3). Thus, our results point to at least a partial recovery of PC synthesis in HS+AA. Interestingly, despite Met being a precursor of taurine, a potent antioxidant that commonly decreases during heat stress (Belal et al., 2018), plasma and milk taurine concentrations did not improve after the increased dietary Met supply in HS+AA. Instead, the increased Met supply likely stimulated the one-carbon metabolism, leading to increased plasma and milk concentration of PC. Met supply can upregulate the phosphatidylethanolamine methyltransferase (PEMT) pathway in bovine liver cells (Zhou et al., 2018) in interaction with folic acid and re-methylation cycles, leading to enhanced synthesis of PC (Myers et al., 2019; McFadden et al., 2020) (Figure 3). In fact, methyl-donor supplementation, through the stimulation of one-carbon metabolism improved the cytoprotective characteristics of heat-stressed polymorphonuclear leukocytes of lactating dairy cows (Lopreiato et al., 2020). In the current experiment, the increased AA supply was associated with a decrease in serum lipopolysaccharide binding protein (LBP) concentration, milk SCC, rectal temperature, and respiration rate during heat stress, suggesting a partial alleviation of the inflammation and oxidative stress (Ruiz-González et al., 2022a). Phosphatidylcholines play a central role in cellular integrity and thus the increased PC availability may help maintain intestinal barrier integrity and prevent the inflammatory consequences of a ‘leaky gut’. In summary, since heat stress appears to enhance PC and LysoPC turnover and utilization by immune activation (Humer et al., 2018), nutritional strategies favoring PC synthesis (including increased dietary Met supply) appear as a potential avenue to alleviate heat stress effects in high-yielding dairy cows.
Plasma hydroxyproline is synthetized from Pro, which also contributes to membrane integrity through the formation of collagen, a key component for cellular thermal stability (Kubow et al., 2015). We detected decreased hydroxyproline concentrations during heat stress in the plasma and decreased Pro concentrations in the milk but not in the plasma. Heat stress enhances protein catabolism including collagen breakdown which is largely composed of Gly and Pro (Ríus, 2019); thus, unchanged Pro and lower hydroxyproline plasma concentrations should be indicative of preferential use of Pro for other processes rather than hydroxyproline synthesis (Figure 3). Pro can act as an antioxidant source (Krishnan et al., 2008) and its lower concentrations in the milk could suggest local utilization to counteract ROS at the mammary gland level. Alternatively, Pro could be channeled to other organs, e.g. the intestines which are particularly challenged by oxidative stress and inflammation during heat stress (Kvidera et al., 2017b). Based on heat-stressed porcine adipocytes, Qu and Ajuwon (2018) suggested that increased Pro catabolism should be linked to antioxidant action during thermal stress.
Lower plasma and milk Gly concentrations in HS and HS+AA are also in agreement with previous studies as this might be the consequence of its increased utilization for antioxidant synthesis and gluconeogenesis in response to heat stress (Ríus, 2019). Fan et al. (2019) reported lower concentrations of both Gly and Pro in the mammary gland of heat-stressed multiparous cows. Gly is involved in the synthesis of folic acid which interacts with the Met cycle and the transsulfuration pathway, hence serving as a precursor for glutathione together with Cys and Glu. In turn, the reduced form of glutathione is a potent antioxidant that typically decreases under heat-stressed conditions (Sordillo and Aitken, 2009; Kawano et al., 2022). In addition, Gly has been suggested to have a direct antioxidant activity in intestinal epithelial cells during heat stress (Wang et al., 2014), supporting the intestinal barrier integrity by regulating the gene expression of tight junction elements (Li et al., 2016). Gly supplementation was shown to alleviate whole-body protein degradation and inflammation in growing piglets challenged by LPS (Wu, 2015). Both of these phenomena (inflammation and labile protein catabolism) also occur in dairy cows in response to heat stress (Ríus, 2019). Thus, the decreased milk concentrations of Gly during HS could be the consequence of its primary use in support of the antioxidant system and counteracting inflammation.
Increased plasma and milk concentrations of Val and Trp in HS+AA likely reflected a combined effect of increased dietary supply and a lower oxidation of these AA due to higher Lys availability. In fact, the HS+AA treatment showed 20% and 27% higher plasma and milk Val concentrations, respectively, and 25% higher milk Trp concentration, compared with HS. However, the dietary supply of Val and Trp was estimated to be 11% higher compared to the other treatments due to the inclusion of blood meal, according to the NASEM system (NASEM, 2021; data not shown). Therefore, we hypothesize that preferential oxidation of Lys due to its increased availability led to downregulated oxidation of Val and Trp and therefore to an increased availability of these EAA. In fact, in the current study, isotopically labeled Leu experiments performed on the last experimental day (day 14) demonstrated that its increased fractional oxidation during heat stress was reversed in HS+AA, possibly due to a higher oxidation of Lys (Ruiz-González et al., 2022b). Our metabolomics data seem to confirm this hypothesis as Lys catabolic products (α-aminoadipic acid, acetyl-carnitine) decreased during heat stress but increased in HS+AA in both plasma and milk (Figure 4). Moreover, Trp which has a common catabolic pathway with Lys, was also increased after the AA increased supply. In particular, α-aminoadipic acid produced from Lys can be metabolized into 2-oxoadipic acid by kynurenine-α-aminoadipic acid transferase, an enzyme previously reported in cows (Deshmukh and Mungre, 1989; Leandro and Houten, 2020). Thus, this enzymatic connection between Trp and Lys could regulate preferential degradation of Lys instead of Trp. Downstream Lys degradation also overlaps with fatty acid degradation, with acetyl-CoA being the catabolic end product. Accordingly, decreased milk acetyl-carnitine in HS was reverted in HS+AA and butyryl-carnitine increased during heat stress and decreased after the increased AA supply, suggesting an enhanced flow of the Lys degradation pathway and improved mitochondrial fatty acid metabolism. In a similar experiment comparing thermoneutral and heat-stressed conditions in multiparous cows, we observed increased milk carnitine and butyryl-carnitine concentrations during heat stress (Jorge-Smeding et al., 2023), suggesting incomplete β-oxidation due to impaired mitochondrial metabolism (Kenéz et al., 2015; Huber et al., 2016; Kinoshita et al., 2018).
Our results disagreed with the findings of Kassube et al. (2017), where Met, Lys and BCAA jugular infusions did not affect milk protein synthesis during heat stress. On the contrary, our previously published results demonstrated that the decreased milk protein content in heat stress was reversed in the HS+AA treatment (Ruiz-González et al., 2022a). Not only did milk protein improve but also milk urea decreased in HS+AA treatment, indicating improved AA utilization efficiency. The increased Met and Lys availability likely also contributed to improved milk protein content as these are usually the most limiting AA (Bequette et al., 1998). Besides, the increased concentrations of other EAA, in particular BCAA, likely stimulated protein synthesis through the mTOR pathway (Doelman et al., 2015). Decreased availability of individual AA and downregulated activity of mTOR, a master regulator of protein synthesis stimulated by BCAA and Lys (Doelman et al., 2015; Lin et al., 2018), were suggested to explain the decreased protein synthesis in the mammary gland during heat stress (Ríus, 2019). Heat stress downregulated the expression of genes involved in AA utilization, AA transport, and mTOR kinase activity in bovine mammary epithelial cells (Kaufman et al., 2018; Dado-Senn et al., 2021). Through a different pathway, insulin also activates downstream mTOR pathways but it is dependent on BCAA availability (Shimobayashi and Hall, 2016; Kenéz et al., 2019). Protein synthesis stimulation in the mammary gland through the mTOR pathway is associated with increased mammary gland insulin/IGF1 sensitivity during lactation (Bionaz and Loor, 2011). In the current experiment, we found increased plasma insulin concentrations during heat stress which was reverted in HS+AA by the end of the experimental period along with the recovery of milk protein content and decrease of milk urea (Ruiz-González et al., 2022a). Lastly, Met was also suggested to activate the mTOR pathway in the mammary gland, however, the exact mechanisms are not yet known (Coleman et al., 2022). Our results indicated that improved milk protein content in HS+AA was associated with the increased availability of EAA, however, the exact interactions between Lys, BCAA, Met and the mTOR pathway are yet to be fully elucidated.
Changes in the concentrations of Phe and Tyr were part of the metabolic response to heat stress, which was independent of Met and Lys availability. Previous heat stress studies reported a parity-dependent increase of Phe and Tyr concentrations, highlighting significant differences only in primiparous cows (Chen et al., 2022). In contrast, our metabolic pathway analysis found the Phe and Tyr metabolism differed only in the multiparous cows. Dado-Senn et al. (2021) observed increased plasma concentrations of Phe after heat stress pointing to links with immune and heat shock response. Phe metabolism interacts with T-cell immune response suppression (Yang et al., 2012) and its supplementation increased HSP70 expression (Plakidou-Dymock and McGivan, 1994). Additionally, Phe is directly catabolized to Tyr by phenylalanine hydroxylase (Crompton et al., 2018). In turn, Tyr can be either oxidized or used to synthesize thyroid hormones (T3, T4) which play a central role in thermal homeostasis and regulation of the basal metabolic rate (Aleena et al., 2016). It has been suggested that decreased blood flow to the thyroid gland due to vasoconstriction during heat stress could lead to downregulated secretion of thyroid hormones in rabbits (Mustafa et al., 2008). Thus, increased concentrations of the Phe and Tyr could reflect downregulated secretion of thyroid hormones leading to the accumulation of its precursors as observed previously (Magdub et al., 1982; Weitzel et al., 2017; Pontiggia et al., 2023).
5 Conclusions
Heat stress caused a shift in the plasma and milk metabolic profiles and some of these changes were restored due to the increased dietary Met, Lys and His availability. Our metabolomics findings herein suggested that this was associated with an increase of the circulating phosphatidylcholine pool. Further, the increased Lys availability associated with the greater availability of the overall BCAA pool can likely explain the increase in milk protein synthesis. Our results suggest that increasing dietary supply of Met, Lys and His can be useful to counteract the negative metabolic effects of heat stress; however, our results pointed to stronger links with Met and Lys, likely due to their higher supplementation rate relative to His. Further studies are warranted to validate these results on a larger independent cohort of cows and to establish specific nutritional recommendations considering parity, genetic merit, and degree of heat stress tolerance.
Data availability statement
The original contributions presented in the study are included in the article/Supplementary Material. Further inquiries can be directed to the corresponding authors.
Ethics statement
The animal study was approved by The Animal Care Committee of the Centre de Recherche en Science Animales de Deschambault. The study was conducted in accordance with the local legislation and institutional requirements.
Author contributions
EJ-S: Writing – original draft, Formal Analysis, Data curation. AR-G: Writing – review & editing, Investigation. YL: Writing – original draft, Investigation. AT: Writing – review & editing, Supervision. AA: Writing – review & editing, Supervision. DO: Writing – review & editing, Resources, Conceptualization. HL: Writing – review & editing, Resources, Conceptualization. DR: Writing – review & editing, Validation, Supervision, Resources, Project administration, Methodology, Funding acquisition, Conceptualization. ÁK: Writing – review & editing, Validation, Supervision, Resources, Project administration, Methodology, Funding acquisition, Conceptualization.
Funding
The author(s) declare financial support was received for the research, authorship, and/or publication of this article. This research was partially supported by the Agriscience program (ASP-60) from Agriculture and Agri-food Canada. The Mitacs Accelerate program provided additional support for AR-G (FR-39554). Internal funding from City University of Hong Kong was received.
Acknowledgments
This paper is dedicated to the memory of our dear PhD student YL (Andy), who passed prematurely and whose enthusiasm for researching animal physiology was unsurpassed. The authors thank P. Ruel, J. Moreau and E. Jacobs for their help during the experiment and for taking care of the technical aspects and animal management. The authors acknowledge The Metabolomics Innovation Centre (Edmonton, Canada) for conducting the metabolomics chemometric analysis.
Conflict of interest
The authors declare that the research was conducted in the absence of any commercial or financial relationships that could be construed as a potential conflict of interest.
Publisher’s note
All claims expressed in this article are solely those of the authors and do not necessarily represent those of their affiliated organizations, or those of the publisher, the editors and the reviewers. Any product that may be evaluated in this article, or claim that may be made by its manufacturer, is not guaranteed or endorsed by the publisher.
Supplementary material
The Supplementary Material for this article can be found online at: https://www.frontiersin.org/articles/10.3389/fanim.2024.1364779/full#supplementary-material
Supplementary Figure 1 | Partial least squares - discriminant analysis (PLS-DA) scores plot for the treatment class (thermoneutral, TN, blue; heat-stressed, HS, green; heat-stressed with high Met, Lys and His supply, HS+AA, red) based on the milk metabolomics profiles. The panel on the right lists the 15 metabolites with the highest variable in projection (VIP) scores.
Supplementary Figure 2 | Log2 fold change of measured phosphatidylcholines (PC) in plasma (A) and milk (B) in HS compared either to TN (blue bars) or HS+AA (orange bars). Negative and positive values indicate lower or higher values in HS, respectively. Treatment effect significance levels indicated in the figure correspond to ANOVA’s raw-P.
References
Aleena J., Pragna P., Archana P. R., Sejian V., Bagath M., Krishnan G., et al. (2016). Significance of metabolic response in livestock for adapting to heat stress challenges. Asian J. Anim. Sci. 10, 224–234. doi: 10.3923/ajas.2016.224.234
Balogh G., Péter M., Glatz A., Gombos I., Török Z., Horváth I., et al. (2013). Key role of lipids in heat stress management. FEBS Lett. 587, 1970–1980. doi: 10.1016/j.febslet.2013.05.016
Baumgard L. H., Rhoads R. P. (2013). Effects of heat stress on postabsorptive metabolism and energetics. Annu. Rev. Anim. Biosci. 1, 311–337. doi: 10.1146/annurev-animal-031412-103644
Belal S., Kang D., Cho E., Park G., Shim K. (2018). Taurine reduces heat stress by regulating the expression of heat shock proteins in broilers exposed to chronic heat. Braz. J. Poult. Sci. 20, 479–486. doi: 10.1590/1806-9061-2017-0712
Bequette B. J., Backwell F. R. C., Crompton L. A. (1998). Current concepts of amino acid and protein metabolism in the mammary gland of the lactating ruminant. J. Dairy Sci. 81, 2540–2559. doi: 10.3168/jds.S0022-0302(98)70147-X
Bernabucci U., Biffani S., Buggiotti L., Vitali A., Lacetera N., Nardone A. (2014). The effects of heat stress in Italian Holstein dairy cattle. J. Dairy Sci. 97, 471–486. doi: 10.3168/jds.2013-6611
Bionaz M., Loor J. J. (2011). Gene networks driving bovine mammary protein synthesis during the lactation cycle. Bioinforma. Biol. Insights 5, 83–98. doi: 10.4137/BBI.S7003
CCAC (1993). Guide to the care and use of experimental animals. 2nd ed (Ottawa: Canadian Council on Animal Care).
Chen X., Dong J. N., Rong J. Y., Xiao J., Zhao W., Aschalew N. D., et al. (2022). Impact of heat stress on milk yield, antioxidative levels, and serum metabolites in primiparous and multiparous Holstein cows. Trop. Anim. Health Prod. 54, 159. doi: 10.1007/s11250-022-03159-x
Chong J., Wishart D. S., Xia J. (2019). Using MetaboAnalyst 4.0 for comprehensive and integrative metabolomics data analysis. Curr. Protoc. Bioinforma 68. doi: 10.1002/cpbi.86
Coleman D. N., Vailati-Riboni M., Pate R. T., Aboragah A., Luchini D., Cardoso F. C., et al. (2022). Increased supply of methionine during a heat-stress challenge in lactating Holstein cows alters mammary tissue mTOR signaling and its response to lipopolysaccharide. J. Anim. Sci. 100, skac175. doi: 10.1093/jas/skac175
Crompton L. A., McKnight L. L., Reynolds C. K., Mills J. A. N., Ellis J. L., Hanigan M. D., et al. (2018). An isotope dilution model for partitioning of phenylalanine and tyrosine uptake by the liver of lactating dairy cows. J. Theor. Biol. 444, 100–107. doi: 10.1016/j.jtbi.2017.12.016
Dado-Senn B., Skibiel A. L., Dahl G. E., Arriola-Apelo S. I., Laporta J. (2021). Dry period heat stress impacts mammary protein metabolism in the subsequent lactation. Animals 11, 2676. doi: 10.3390/ani11092676
del Bas J. M., Caimari A., Rodriguez-Naranjo M. I., Childs C. E., Paras Chavez C., West A. L., et al. (2016). Impairment of lysophospholipid metabolism in obesity: altered plasma profile and desensitization to the modulatory properties of n–3 polyunsaturated fatty acids in a randomized controlled trial. Am. J. Clin. Nutr. 104, 266–279. doi: 10.3945/ajcn.116.130872
Deshmukh D. R., Mungre S. M. (1989). Purification and properties of 2-aminoadipate: 2-oxoglutarate aminotransferase from bovine kidney. Biochem. J. 261, 761–768. doi: 10.1042/bj2610761
Doelman J., Kim J. J. M., Carson M., Metcalf J. A., Cant J. P. (2015). Branched-chain amino acid and lysine deficiencies exert different effects on mammary translational regulation. J. Dairy Sci. 98, 7846–7855. doi: 10.3168/jds.2015-9819
Dong X., Zhou Z., Saremi B., Helmbrecht A., Wang Z., Loor J. J. (2018). Varying the ratio of Lys : Met while maintaining the ratios of Thr : Phe, Lys : Thr, Lys : His, and Lys : Val alters mammary cellular metabolites, mammalian target of rapamycin signaling, and gene transcription. J. Dairy Sci. 101, 1708–1718. doi: 10.3168/jds.2017-13351
Fan C., Su D., Tian H., Hu R., Ran L., Yang Y., et al. (2019). Milk production and composition and metabolic alterations in the mammary gland of heat-stressed lactating dairy cows. J. Integr. Agric. 18, 2844–2853. doi: 10.1016/S2095-3119(19)62834-0
Foroutan A., Guo A. C., Vazquez-Fresno R., Lipfert M., Zhang L., Zheng J., et al. (2019). Chemical composition of commercial cow’s milk. J. Agric. Food Chem. 67, 4897–4914. doi: 10.1021/acs.jafc.9b00204
Huber K., Dänicke S., Rehage J., Sauerwein H., Otto W., Rolle-Kampczyk U., et al. (2016). Metabotypes with properly functioning mitochondria and anti-inflammation predict extended productive life span in dairy cows. Sci. Rep. 6, 24642. doi: 10.1038/srep24642
Humer E., Aditya S., Zebeli Q. (2018). Innate immunity and metabolomic responses in dairy cows challenged intramammarily with lipopolysaccharide after subacute ruminal acidosis. Animal 12, 2551–2560. doi: 10.1017/S1751731118000411
Hung N. D., Sok D.-E., Kim M. R. (2012). Prevention of 1-palmitoyl lysophosphatidylcholine-induced inflammation by polyunsaturated acyl lysophosphatidylcholine. Inflamm. Res. 61, 473–483.
Javaid A., Wang F., Horst E., Diaz-Rubio M. E., Wang L., Baumgard L. H., et al. (2022). Effects of acute intravenous lipopolysaccharide administration on the plasma lipidome and metabolome in lactating Holstein cows experiencing hyperlipidemia. Metabolomics 18, 75. doi: 10.1007/s11306-022-01928-1
Jorge-Smeding E., Leung Y. H., Ruiz-González A., Xu W., Astessiano A. L., Trujillo A. I., et al. (2023). Plasma and milk metabolomics revealed changes in amino acid metabolism in Holstein dairy cows under heat stress. Animal, 101049. doi: 10.1016/j.animal.2023.101049
Kassube K. R., Kaufman J. D., Pohler K. G., McFadden J. W., Ríus A. G. (2017). Jugular-infused methionine, lysine and branched-chain amino acids does not improve milk production in Holstein cows experiencing heat stress. Animal 11, 2220–2228. doi: 10.1017/S1751731117001057
Kaufman J. D., Pohler K. G., Mulliniks J. T., Ríus A. G. (2018). Lowering rumen-degradable and rumen-undegradable protein improved amino acid metabolism and energy utilization in lactating dairy cows exposed to heat stress. J. Dairy Sci. 101, 386–395. doi: 10.3168/jds.2017-13341
Kawano K., Sakaguchi K., Madalitso C., Ninpetch N., Kobayashi S., Furukawa E., et al. (2022). Effect of heat exposure on the growth and developmental competence of bovine oocytes derived from early antral follicles. Sci. Rep. 12, 8857. doi: 10.1038/s41598–022-12785–2
Kenéz Á., Ruda L., Dänicke S., Huber K. (2019). Insulin signaling and insulin response in subcutaneous and retroperitoneal adipose tissue in Holstein cows during the periparturient period. J. Dairy Sci. 102, 11718–11729. doi: 10.3168/jds.2019-16873
Kenéz Á., Tienken R., Locher L., Meyer U., Rizk A., Rehage J., et al. (2015). Changes in lipid metabolism and β-adrenergic response of adipose tissues of periparturient dairy cows affected by an energy-dense diet and nicotinic acid supplementation. J. Anim. Sci. 93, 4012–4022. doi: 10.2527/jas.2014–8833
Kinoshita A., Kenéz Á., Hasselmann M., Dänicke S., Huber K. (2018). Inter-individual variation in adaptive capacity at onset of lactation: Linking metabolic phenotype with mitochondrial DNA haplotype in Holstein dairy cows. Sci. Rep. 8, 15439. doi: 10.1038/s41598–018-33853–6
Krishnan N., Dickman M. B., Becker D. F. (2008). Proline modulates the intracellular redox environment and protects mammalian cells against oxidative stress. Free Radic. Biol. Med. 44, 671–681. doi: 10.1016/j.freeradbiomed.2007.10.054
Kubow K. E., Vukmirovic R., Zhe L., Klotzsch E., Smith M. L., Gourdon D., et al. (2015). Mechanical forces regulate the interactions of fibronectin and collagen I in extracellular matrix. Nat. Commun. 6, 8026. doi: 10.1038/ncomms9026
Kvidera S. K., Dickson M. J., Abuajamieh M., Snider D. B., Fernandez M. V. S., Johnson J. S., et al. (2017a). Intentionally induced intestinal barrier dysfunction causes inflammation, affects metabolism, and reduces productivity in lactating Holstein cows. J. Dairy Sci. 100, 4113–4127. doi: 10.3168/jds.2016-12349
Kvidera S. K., Horst E. A., Abuajamieh M., Mayorga E. J., Fernandez M. V. S., Baumgard L. H. (2017b). Glucose requirements of an activated immune system in lactating Holstein cows. J. Dairy Sci. 100, 2360–2374. doi: 10.3168/jds.2016-12001
Lambert G. P., Gisolfi C. V., Berg D. J., Moseley P. L., Oberley L. W., Kregel K. C. (2002). Hyperthermia-induced intestinal permeability and the role of oxidative and nitrosative stress. J. Appl. Physiol. 92, 1750–1761. doi: 10.1152/japplphysiol.00787.2001
Leandro J., Houten S. M. (2020). The lysine degradation pathway: Subcellular compartmentalization and enzyme deficiencies. Mol. Genet. Metab. 131, 14–22. doi: 10.1016/j.ymgme.2020.07.010
Lee C., Hristov A. N., Cassidy T. W., Heyler K. S., Lapierre H., Varga G. A., et al. (2012). Rumen-protected lysine, methionine, and histidine increase milk protein yield in dairy cows fed a metabolizable protein-deficient diet. J. Dairy Sci. 95, 6042–6056. doi: 10.3168/jds.2012-5581
Li W., Sun K., Ji Y., Wu Z., Wang W., Dai Z., et al. (2016). Glycine Regulates Expression and distribution of claudin-7 and ZO-3 proteins in intestinal porcine epithelial cells. J. Nutr. 146, 964–969. doi: 10.3945/jn.115.228312
Lin X., Shanshan L., Zou Y., Zhao F.-Q., Liu K., Liu H. (2018). Lysine stimulates protein synthesis by promoting the expression of ATB0,+ and activating the mTOR pathway in bovine mammary epithelial cells. J. Nutr. 148, 1426–1143. doi: 10.1093/jn/nxy140
Liu Z., Ezernieks V., Wang J., Arachchillage N. W., Garner J. B., Wales W. J., et al. (2017). Heat stress in dairy cattle alters lipid composition of milk. Sci. Rep. 7, 961. doi: 10.1038/s41598–017-01120–9
Lopreiato V., Vailati-Riboni M., Parys C., Fernandez C., Minuti A., Loor J. J. (2020). Methyl donor supply to heat stress-challenged polymorphonuclear leukocytes from lactating Holstein cows enhances 1-carbon metabolism, immune response, and cytoprotective gene network abundance. J. Dairy Sci. 103, 10477–10493. doi: 10.3168/jds.2020-18638
Magdub A., Johnson H. D., Belyea R. L. (1982). Effect of environmental heat and dietary fiber on thyroid physiology of lactating cows. J. Dairy Sci. 65, 2323–2331. doi: 10.3168/jds.S0022-0302(82)82504-6
McFadden J. W., Girard C. L., Tao S., Zhou Z., Bernard J. K., Duplessis M., et al. (2020). Symposium review: One-carbon metabolism and methyl donor nutrition in the dairy cow. J. Dairy Sci. 103, 5668–5683. doi: 10.3168/jds.2019-17319
Mesgaran M., Kargar H., Janssen R., Danesh Mesgaran S., Ghesmati A., Vatankhah A. (2022). Rumen-protected zinc–methionine dietary inclusion alters dairy cow performances, and oxidative and inflammatory status under long-term environmental heat stress. Front. Vet. Sci. 9. doi: 10.3389/fvets.2022.935939
Mustafa S., Al-Bader M. D., Elgazzar A. H., Alshammeri J., Gopinath S., Essam H. (2008). Effect of hyperthermia on the function of thyroid gland. Eur. J. Appl. Physiol. 103, 285–288. doi: 10.1007/s00421-008-0701-2
Myers W. A., Rico J. E., Davis A. N., Fontoura A. B. P., Dineen M. J., Tate B. N., et al. (2019). Effects of abomasal infusions of fatty acids and one-carbon donors on hepatic ceramide and phosphatidylcholine in lactating Holstein dairy cows. J. Dairy Sci. 102, 7087–7101. doi: 10.3168/jds.2018-16200
NASEM (2021). Nutrient requirements of dairy cattle: eighth revised edition (Washington, DC: National Academies Press). doi: 10.17226/25806
NRC (2001). Nutrient requirements of dairy cattle. 7th ed (Washington, DC: National Research Council).
Pate R. T., Luchini D., Murphy M. R., Cardoso F. C. (2020). Effects of rumen-protected methionine on lactation performance and physiological variables during a heat stress challenge in lactating Holstein cows. J. Dairy Sci. 103, 2800–2813. doi: 10.3168/jds.2019-17305
Pearce S. C., Mani V., Weber T. E., Rhoads R. P., Patience J. F., Baumgard L. H., et al. (2013). Heat stress and reduced plane of nutrition decreases intestinal integrity and function in pigs1. J. Anim. Sci. 91, 5183–5193. doi: 10.2527/jas.2013-6759
Plakidou-Dymock S., McGivan J. D. (1994). Amino acid deprivation-induced stress response in the bovine renal epithelial cell line NBL-1: Induction of HSP 70 by phenylalanine. Biochim. Biophys. Acta BBA - Mol. Cell Res. 1224, 189–197. doi: 10.1016/0167-4889(94)90190-2
Pontiggia A., Münger A., Ammer S., Philipona C., Bruckmaier R. M., Keil N. M., et al. (2023). Short-term physiological responses to moderate heat stress in grazing dairy cows in temperate climate. Animal 17, 100718. doi: 10.1016/j.animal.2023.100718
Qu H., Ajuwon K. M. (2018). Metabolomics of heat stress response in pig adipose tissue reveals alteration of phospholipid and fatty acid composition during heat stress1. J. Anim. Sci. 96, 3184–3195. doi: 10.1093/jas/sky127
Räisänen S. E., Lage C. F. A., Fetter M. E., Melgar A., Pelaez A. M., Stefenoni H. A., et al. (2021). Histidine dose-response effects on lactational performance and plasma amino acid concentrations in lactating dairy cows: 2. Metabolizable protein-deficient diet. J. Dairy Sci. 104, 9917–9930. doi: 10.3168/jds.2021-20189
R Core Team. (2021). R: A language and environment for statistical computing. (Vienna, Austria: R Foundation for Statistical Computing). Available at: https://www.R-project.org/.
Rhoads M. L., Rhoads R. P., VanBaale M. J., Collier R. J., Sanders S. R., Weber W. J., et al. (2009). Effects of heat stress and plane of nutrition on lactating Holstein cows: I. Production, metabolism, and aspects of circulating somatotropin. J. Dairy Sci. 92, 1986–1997. doi: 10.3168/jds.2008-1641
Rico J. E., Saed Samii S., Zang Y., Deme P., Haughey N. J., Grilli E., et al. (2021). Characterization of the plasma lipidome in dairy cattle transitioning from gestation to lactation: Identifying novel biomarkers of metabolic impairment. Metabolites 11, 290. doi: 10.3390/metabo11050290
Ríus A. G. (2019). Invited Review: Adaptations of protein and amino acid metabolism to heat stress in dairy cows and other livestock species. Appl. Anim. Sci. 35, 39–48. doi: 10.15232/aas.2018-01805
Ruiz-González A., Leung Y. H., Celemin A., Kenéz A., Chouinard P. Y., Gervais R., et al. (2022b). “Whole-body protein and glucose metabolism in cows fed diets with varying amino acid supply under heat stress,” in Abstracts of the 2022 American dairy science association annual meeting, vol. 93, Suppl. 1. (Kansas, USA: Journal of Dairy Science). J. Dairy Sci.
Ruiz-González A., Leung Y. H., Celemin A., Kenéz A., Ouellet D. R., Lapierre H., et al. (2022a). “Production performance and metabolism of Holstein cows fed low protein diet balanced for lysine, methionine and histidine during,” in Abstracts of the 2022 American dairy science association annual meeting, vol. 141, Suppl. 1. (Kansas, USA). J. Dairy Sci.
Ruiz-González A., Suissi W., Baumgard L. H., Martel-Kennes Y., Chouinard P. Y., Gervais R., et al. (2023). Increased dietary vitamin D3 and calcium partially alleviate heat stress symptoms and inflammation in lactating Holstein cows independent of dietary concentrations of vitamin E and selenium. J. Dairy Sci. 106, 3984–4001. doi: 10.3168/jds.2022-22345
Schwab C. G., Broderick G. A. (2017). A 100-Year Review: Protein and amino acid nutrition in dairy cows. J. Dairy Sci. 100, 10094–10112. doi: 10.3168/jds.2017-13320
Shimobayashi M., Hall M. N. (2016). Multiple amino acid sensing inputs to mTORC1. Cell Res. 26, 7–20. doi: 10.1038/cr.2015.146
Sordillo L. M., Aitken S. L. (2009). Impact of oxidative stress on the health and immune function of dairy cattle. Vet. Immunol. Immunop 128, 104–109. doi: 10.1016/j.vetimm.2008.10.305
Thornton P., Nelson G., Mayberry D., Herrero M. (2021). Increases in extreme heat stress in domesticated livestock species during the twenty-first century. Glob. Change Biol. 27, 5762–5772. doi: 10.1111/gcb.15825
Vinaixa M., Samino S., Saez I., Duran J., Guinovart J. J., Yanes O. (2012). A guideline to univariate statistical analysis for LC/MS-based untargeted metabolomics-derived data. Metabolites 2, 775–795. doi: 10.3390/metabo2040775
Wang W., Wu Z., Lin G., Hu S., Wang B., Dai Z., et al. (2014). Glycine stimulates protein synthesis and inhibits oxidative stress in pig small intestinal epithelial Cells. J. Nutr. 144, 1540–1548. doi: 10.3945/jn.114.194001
Weitzel J. M., Viergutz T., Albrecht D., Bruckmaier R., Schmicke M., Tuchscherer A., et al. (2017). Hepatic thyroid signaling of heat-stressed late pregnant and early lactating cows. J. Endocrinol. 234, 129–141. doi: 10.1530/JOE-17-0066
Wheelock J. B., Rhoads R. P., VanBaale M. J., Sanders S. R., Baumgard L. H. (2010). Effects of heat stress on energetic metabolism in lactating Holstein cows. J. Dairy Sci. 93, 644–655. doi: 10.3168/jds.2009-2295
Wu H. T. (2015). Regulative effect of glycine on intestinal injury and muscle protein synthesis and degradation of piglets after lipopolysaccharide challenge. Wuhan Polytechnic Univ., Dongxihu Qu, Wuhan Shi, Hubei Sheng, China.
Yang B., Wang X., Ren X. (2012). Amino acid metabolism related to immune tolerance by MDSCs. Int. Rev. Immunol. 31, 177–183. doi: 10.3109/08830185.2012.679989
Zhao X., Niu L., Clerici C., Russo R., Byrd M., Setchell K. D. R. (2019). Data analysis of MS-based clinical lipidomics studies with crossover design: A tutorial mini-review of statistical methods. Clin. Mass Spectrom. 13, 5–17. doi: 10.1016/j.clinms.2019.05.002
Zhou Y. F., Zhou Z., Batistel F., Martinez-Cortés I., Pate R. T., Luchini D. L., et al. (2018). Methionine and choline supply alter transmethylation, transsulfuration, and cytidine 5′-diphosphocholine pathways to different extents in isolated primary liver cells from dairy cows. J. Dairy Sci. 101, 11384–11395. doi: 10.3168/jds.2017-14236
Keywords: targeted metabolomics, metabolic health, one-carbon metabolism, phosphatidylcholines, essential amino acids
Citation: Jorge-Smeding E, Ruiz-González A, Leung YH, Trujillo AI, Astessiano AL, Ouellet DR, Lapierre H, Rico DE and Kenéz Á (2024) Increased dietary methionine, lysine and histidine supply modulated the heat stress-induced metabolic remodeling of dairy cows. Front. Anim. Sci. 5:1364779. doi: 10.3389/fanim.2024.1364779
Received: 03 January 2024; Accepted: 21 May 2024;
Published: 10 June 2024.
Edited by:
Gianni Battacone, University of Sassari, ItalyReviewed by:
Gibson Maswayi Alugongo, China Agricultural University, ChinaSamer Wassim El-Kadi, Virginia Tech, United States
Copyright © 2024 Jorge-Smeding, Ruiz-González, Leung, Trujillo, Astessiano, Ouellet, Lapierre, Rico and Kenéz. This is an open-access article distributed under the terms of the Creative Commons Attribution License (CC BY). The use, distribution or reproduction in other forums is permitted, provided the original author(s) and the copyright owner(s) are credited and that the original publication in this journal is cited, in accordance with accepted academic practice. No use, distribution or reproduction is permitted which does not comply with these terms.
*Correspondence: Daniel E. Rico, ZGFuaWVsLnJpY29AY3JzYWQucWMuY2E=; Ákos Kenéz, YWtvcy5rZW5lekBjaXR5dS5lZHUuaGs=