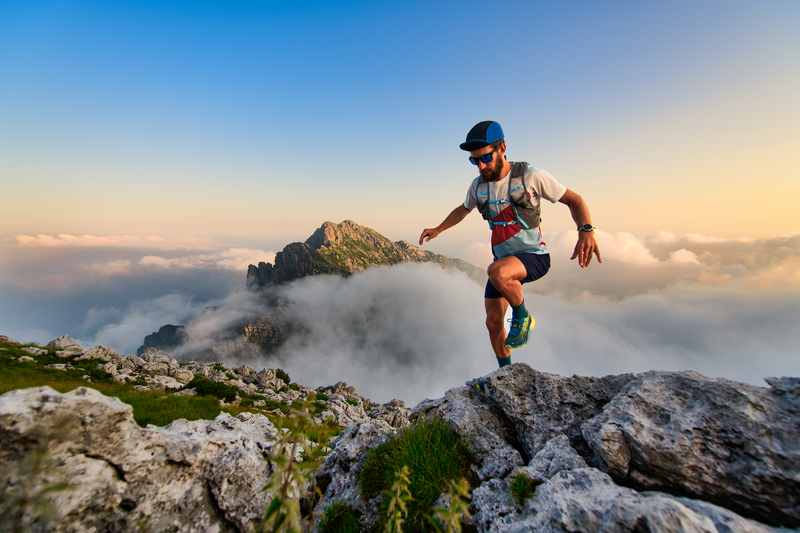
95% of researchers rate our articles as excellent or good
Learn more about the work of our research integrity team to safeguard the quality of each article we publish.
Find out more
ORIGINAL RESEARCH article
Front. Anim. Sci. , 23 January 2024
Sec. Animal Nutrition
Volume 5 - 2024 | https://doi.org/10.3389/fanim.2024.1320075
Introduction: Three in vitro experiments were conducted to evaluate the effects of increasing levels of Enterococcus faecium and Saccharomyces cerevisiae (DFM1) and increasing levels of Bacillus licheniformis and Bacillus subtilis (DFM2) on in vitro ruminal fermentation parameters in three different dietary scenarios.
Methods: For Exp. 1, the basal diet consisted of 25:75 roughage:concentrate ratio (R:C) and was composed by 5 treatments: control (no additive), 2 levels of DFM1 (1X = 1.9 mg and 5X = 9.0 mg), and 2 levels of DFM2 (1X = 3.8 mg and 5X = 19 mg). The Exp. 2 consisted of a 41:59 R:C diet and was composed by 5 treatments: control (no additive) and 2 levels of DFM1 (1X = 3.8 mg and 5 X = 19 mg) and 2 levels of DFM2 (1X = 5.6 mg and 5X = 28 mg). The Exp. 3 consisted of a 100:0 R:C diet [Brachiaria (syn. Urochloa brizantha)] and was composed by the same treatments described in Exp. 1. The DFM1 contained 3.5 × 109 CFU per g of Enterococccus faecium and Saccharomyces cerevisiae, whereas the DFM2 contained Bacillus licheniformis and Bacillus subtilis at 3.2 × 109 CFU per g. In each Exp., an in vitro gas production (GP) system with 43-bottles (AnkomRF) was used in four consecutive 48 or 72-h fermentation batches to evaluate total GP (TGP), kinetics and fermentation profiles, methane (CH4), and carbon dioxide (CO2).
Results: For Exp 1, DFM1 increased quadratically TGP at 24 and 48-h, which reflected in a greater in vitro organic matter digestibility (IVOMD). The concentrations of ammonia-N, CH4, and CO2 (mmol/g of IVOMD) reduced quadratically as DFM1 increased. For Exp. 2, DFM1 inclusion reduced butyrate concentration and acetate to propionate ratio. Regarding GHG emissions, DFM1 and DFM2 quadratically reduced CH4 and CO2 emission per IVOMD (mmol/g of IVOMD). For Exp. 3, DFM1 increased quadratically TGP at 48h with no impact on IVOMD. Otherwise, DFM2 increased linearly TGP at 24 and 48h which reflected in a greater IVOMD. The inclusion of DFM1 increased linearly iso-valerate and branched-chain volatile fatty acids (BCVFA) concentration and DFM2 addition increased BCVFA quadratically.
Discussion: Overall, addition of DFM1 [Enterococccus faecium (5 × 109 CFU per g) + Saccharomyces cerevisiae (5 × 109 CFU per g)] or DFM2 [Bacillus licheniformis + Bacillus subtilis (3.2 × 109 CFU per g)] might enhance the fermentation process in the rumen and decrease greenhouse gas emissions in a dose-dependent manner, though the results are contingent on the specific type of diet.
As potential alternatives to antibiotic feeding, there has been an increasing interest in direct-fed microbials (DFM) for beef and dairy cattle (Krehbiel et al., 2003). Direct-fed microbials must contain live microorganisms (bacteria and/or yeast) that support health (FAO/WHO, 2001) and rumen function of the host. Due to their direct effects on microbial communities and cellular function, DFM were primarily studied regarding their impact on immune modulation in the gastrointestinal tract (Krehbiel et al., 2003; Ballou et al., 2019). However, positive effects of DFM on rumen fermentation have also been reported in the literature (Nocek et al., 2003; Qiao et al., 2010; Lettat et al., 2012; Mamuad et al., 2019; Dias et al., 2022; Wang et al., 2022).
Saccharomyces cerevisiae is among the most studied microorganisms used as DFM for ruminants (Ban and Guan, 2021). Among the beneficial effects of S. cerevisiae supplementation, the supply of growth factors for cellulolytic and amylolytic bacteria, inhibition of lactic acid accumulation in the rumen, improvement of microbial protein synthesis, and modulation of rumen fermentation characteristics are highlighted (Pinloche et al., 2013; Jiang et al., 2017; Dias et al., 2018; Amin and Mao, 2021); however, results may vary depending on dietary forage to concentrate ratio (Williams et al., 1991; Carro et al., 1992; Desnoyers et al., 2009). Enterococci have also been used as a DFM for ruminants (Shridhar et al., 2022), presenting two potential benefits to the rumen environment: 1) adapting microbes to the presence of lactic acid and 2) stimulating the growth of lactic acid-utilizing bacteria (Yoon and Stern, 1995; Seo et al., 2010). Lastly, Bacilli, such as B. licheniformis and B. subtilis, are spore-forming bacteria recognized through their ability to produce enzymes and secondary metabolites (Schallmey et al., 2004; Bernardeau et al., 2017). Moreover, these microbes also have direct inhibitory effects against potentially harmful bacteria (Lim et al., 2021), while also positively impacting rumen nutrient digestibility, volatile fatty acids (VFA) production, and microbial protein synthesis (Qiao et al., 2010; Sun et al., 2013; Pan et al., 2022; Cappellozza et al., 2023).
Altogether, DFM may improve feed efficiency of the herd by reducing energy losses through rumen fermentation towards propionate production (Ban and Guan, 2021; Dias et al., 2022). Because propionate has higher enthalpy than acetate and can be oxidized by the animal, more feed energy is available for productive purposes (Russel and Strobel, 1989). From a sustainability perspective, propionate production in the rumen may also reduce H2 availability for enteric methane production by the methanogenic microbes (Wang et al., 2018). In addition, DFM may stimulate ruminal lactate-utilizing bacteria (Pinloche et al., 2013; Jiang et al., 2017), which compete for H2 in the rumen, decreasing its availability for methanogenesis.
As few studies have explored the potential of different DFM supplementation on gas production, methane (CH4) and carbon dioxide (CO2) and volatile fatty acids (VFA) profile in the same study of ruminants fed different diets, the objective of the present study was to evaluate different combinations and levels of DFM [(DFM1 (E. faecium + S. cerevisiae) and DFM2 (B. licheniformis + B. subtilis)] on in vitro ruminal fermentation, gas production kinetic, and CH4 and CO2 emissions in high-grain, medium-grain, and forage-based diets. We hypothesized that the individual aforementioned effects of S. cerevisae and E. faecium and the effects of B. licheniformis and B. subtilis may act synergically when blended in a dose-dependent-manner, improving the in vitro ruminal fermentation and gas production kinetic, reducing CH4 and CO2 emissions regardless of the diet.
The experiment was carried out at the Instituto de Zootecnia, Beef Cattle Research Center, located in Sertãozinho, SP, Brazil. The experiment was approved by the Animal Use Ethics Committee of the Instituto de Zootecnia under the protocol n° 363-2022.
Three experiments were conducted to evaluate the effects of two DFM when added in different levels into three different diets. For each of the Experiments reported below, two doses of each DFM (DFM1 and DFM2) were tested independently (1X and 5X). The total amount of each DFM incubated in the 1X diets followed the manufacturer recommendation; however, we hypothesized that increasing this dose by 5 times the response could be better, especially on in vitro studies that for some specific additives require higher dose to be effective, due to the short period on fermentation. In addition, this level of spacing between these doses could improve our power to detect any difference among them, providing us with an accurate answer to be employed on farm. The DFM1 (Probios® Precise; Chr. Hansen A/S, Hørsholm, Denmark) was a combination of three strains of Enterococccus faecium and one strain of Saccharomyces cerevisiae (3.5 × 109 CFU per g), whereas the DFM2 (Bovacillus™; Chr. Hansen A/S) contained Bacillus licheniformis and B. subtilis (3.2 × 109 CFU per g). The viability, identity, and concentration of probiotic strains utilized in the current study were not tested prior to use. However, since all tested DFM products are commercially available, the manufacturer did perform quality assurance on each batch utilized in the present experiments.
In Exp. 1, the basal diet consisted of a typical finishing beef cattle diet (25:75 roughage:concentrate ratio) and was composed by the following treatments: Control (basal diet with no additive; CON), DFM1 at 1X and 5X (1.9 and 9.0 mg, respectively), and DFM2 at 1X and 5X (3.8 and 19 mg, respectively). In the Exp. 2, the basal diet consisted of a typical lactating dairy cow diet (40:60 roughage:concentrate ratio) and was composed by the following treatments: CON (basal diet with no additive), DFM1 at 1X and 5X (3.8 and 19.0 mg, respectively), and DFM2 at 1X and 5X (5.6 and 28 mg, respectively). Finally, in Exp. 3, the basal diet consisted of a forage-based diet (100:0 roughage:concentrate ratio) and was composed by the same treatments as in Exp. 1. In all Exp., the DFM dosages were calculated proportionally considering a rumen capacity of 80 L.
The diet of Exp. 1 was formulated to meet the nutrient requirements of a non-castrated beef steer with an estimated averaged daily gain (ADG) of 1.6 kg (NASEM, 2016). In Exp. 2, the diet was formulated to meet the nutrient requirements of a lactating dairy cow producing 40 kg of milk/d (NASEM, 2021). For the Exp. 3, an exclusive low-quality forage-based diet was utilized with the aim of simulating pasture conditions during the dry season in tropical regions. The nutritional profile of the diets used in Exp. 1, 2, and 3 is reported in Table 1.
For all Exp., a 43-bottle automated in vitro gas production (GP) system (Ankom Technology, Macedon, NY, USA) equipped with wireless pressure sensors connected to a computer was used to evaluate the ruminal fermentation pattern of tested ingredients. Thus, treatments were evaluated at 24-, 48-, or 72-h fermentation incubations to assess the in vitro GP profiles and ruminal fermentation parameters. The experimental units were the fermentation batches (true replicates; n = 4/experiment) and were the mean of four 250-mL bottles that were randomly arranged in the incubator. In addition, 3 flasks were used as blanks (only rumen/mineral/buffer solution), totaling 160 observations. A fermentation batch was performed 1 d after the previous fermentation batch ended.
All ingredients were ground through a 1-mm screen using a Wiley mill (TE 650; Tecnal® Piracicaba, SP, Brazil) prior to incubations and for further analyses. Samples were analyzed for dry matter (DM; method 930.15; AOAC, 1990), ash (method 942.05; AOAC, 1990), crude protein (CP; Dumatherm®; Gerhardt GmbH & Co, Königswinter, Germany; method 990.13; AOAC, 2005) and ether extract (EE; method 2003.05; AOAC, 1990). The organic matter (OM) was calculated as the difference between DM and ash contents. For neutral detergent fiber (NDF), samples were treated with alpha thermo-stable amylase without sodium sulfite (van Soest et al., 1991) and adapted for a Fiber Analyzer (TE 149; Tecnal®).
The rumen fluid from three Nellore steers cannulated in the rumen (average body weight of 640 kg) were mixed prior to use in the incubations. Steers were adapted for 21 d prior to the first batch and maintained during the incubation batches on a total mix diet of 60% corn silage and 40% concentrate (ground corn grain, citrus pulp pellet, soybean meal, and mineral mixture) for Exp. 1 and 2. For Exp., 3 three steers were adapted for 21 d prior to the first batch and maintained during the incubation batches on pasture with brachiaria grass (Syn. Urochloa) the same brachiaria that was used as substrate in Exp. 3. For all Exp., two hours after feeding, 2,000 mL of rumen fluid was collected from each animal, immediately filtered through 4 layers of cheesecloth, placed into pre-warmed (39°C) thermal bottles, and immediately transported to the laboratory (Yáñez-Ruiz et al., 2016). The incubations were performed within 30 min after rumen fluid collection. In addition, a fermentation batch was performed 1 d after the previous fermentation batch ended.
The buffer mineral solutions of all Exp. were prepared according to Menke and Steingass (1988). The buffer solution was kept in a water bath at 39°C and purged continuously with nitrogen (N2) for 30 min. Resazurin was used as color indicator to control the buffer pH and N2 saturation (oxidation-reduction potential). The rumen fluid was mixed with the buffer solution (1:2 v/v) in water bath at 39°C under anaerobic conditions by flushing N2 (Menke and Steingass, 1988).
The AnkomRF system bottle valves were set to be vented. Each bottle (250-mL) was filled with 0.5 g (DM basis) of each diet. Diet samples were hydrated with deionized water to avoid particle dispersion. Bottles were inoculated with 75 mL of rumen/buffer solution keeping the headspace of bottle continuously flushed with N2 for 1 min per bottle. After inoculation, bottles were closed and placed in the air-ventilated shaker incubator (EI-450T, ENGCO, Piracicaba, SP, Brazil) under controlled temperature (39°C) and agitation (83 rpm). The data acquisition software (Gas Pressure Monitor, Ankom Technology, NY, USA) was set to monitor the cumulative pressure every 5 min and data were recorded every 60 min for 48 h in Exp. 1 and 2 and for 72 h for Exp. 3. Valves were set to automatic release the gas when the pressures reached 3.4 kPa (F. Tagliapietra et al., 2010).
The cumulated gas pressures – total GP (TGP) – at 24 and 48 in Exp. 1 and 2, and 24, 48, and 72 h in Exp. 3 were converted into mL according to Tagliapietra et al. (2011) as follows:
GP, mL = (Pc / Po) × Vo,
where Pc is the cumulated pressure change (kPa) in the bottle headspace, Po is the atmospheric pressure read by the equipment at the beginning of the measurement, and Vo is the bottle headspace volume (95 mL).
The bottle final TGP volumes were corrected for inoculum contribution by subtracting the final TGP of the blank bottles. For TGP over time, the cumulative pressure values were adjusted to assess biological values using the following dual-pool model (Schofield et al., 1994):
Vt = [V1 / (1 + e2 + 4 × [K1 × (L - Time)])] + [V2 / (1 + e2 + 4 × [K2 × (L - Time)])],
where: Vt = gas volume produced up to the specific time, mL; V1 and V2 = maximum gas volume achieved from complete digestion of each pool, mL; K1 and K2 = specific rate of digestion of each pool, h-1; L = lag time, h. The solution pH was measured (Accumet™ AP61, Fisher Scientific, Atlanta, GA) at the beginning and at the end of each incubation (48 h - Exp. 1 and 2; 72 h - Exp. 3).
The in vitro OM digestibility (IVOMD) was calculated according to Menke and Steingass (1988), as follows:
IVOMD (g/kg DM) = 31.55 + 0.8343 × GP200,
where GP200 is the net gas production (mL/200 mg of DM incubated) at 24 and 48 in Exp. 1 and 2, and 24, 48, and 72 h in Exp. 3.
For ammonia-nitrogen (NH3-N) and volatile fatty acids (VFA) analysis, subsamples of 15 mL from the rumen/buffer solution before incubation and from each bottle at 48 h were filtered through four layers of cheesecloth. Then, 0.2 mL of a 500 mL/L of a H2SO4-based solution was added for determination of NH3-N and VFA. Samples were centrifuged for 15 min at 4°C and 10,000 g, then the supernatant was centrifuged for 30 min at 4°C and 20,0000 g (Avanti JXN-26, Beckman Coulter, Nyon, Switzerland). The VFA concentrations were determined using gas chromatography (Nexis GC-2030; Shimadzu, Japan) equipped with a glass capillary (Supelco Nukol™; 30,000 cm × 0.53 mm i.d.) and coupled to a flame detector ionization and N2 was used as a carrier gas. The NH3-N concentration was determined by colorimetry as described by Chaney and Marbach (1962). The total VFA and NH3-N concentrations were calculated by subtracting the values measured on the initial content of the components in the rumen/buffer solution (blank bottles without treatments) from the final concentrations of each bottle (Tagliapietra et al., 2013). The pH of the solution was measured (Accumet™ AP61, Fisher Scientific, Atlanta, GA) at the beginning and at the end of each incubation (48 or 72 h).
For these analyses, the AnkomRF system bottle valves were set to be closed. All other procedures and design were the same as reported for the TGP assay. After inoculation, bottles were sealed and then placed into an air-ventilated shaker incubator (39°C). At the end of each fermentation batch 48 h for Exp. 1 and 2; and 72 h for Exp. 3, CO2 and CH4 production were measured from the headspace using a gas chromatograph (Nexis GC-2030, Shimadzu) equipped with a GS-Carbonplot capillary (Agilent Technologies Inc.; 30,000 cm × 0.32 mm i.d) coupled to a discharge ionization detector and helium (999.9 mL/L) was used as the carrier gas. The CH4 and CO2 production was corrected for inoculum contribution by subtracting the final GP of the blank bottles.
All statistical analyses were performed in SAS version 9.4 (SAS Institute Inc., Cary, NC). All results were assessed for residual normality and variance homogeneity. For all Exp., data were collected and analyzed in a completely randomized design by fitting the data using the GLIMMIX procedure of SAS, considering treatments as fixed factors and bottle (incubation) as a random factor. The average values obtained from the bottles within each incubation was considered as the experimental unit for all analyses reported herein. Non-linear model (NLIN procedure) was used to estimate the fermentation rate and gas pool size. The parameters of the nonlinear functions as well as all other variables were fitted through a generalized mixed model and compared by using the test of Tukey and polynomial linear regressions. The DFM dosages (1X and 5X) were also analyzed for linear and quadratic responses using the following model:
Yij = B0 + B1Xi + B2Xi2 + Pj + eij,
where Yijk is the observed measurement of the ith level of additive in the diet of the jth incubation, and of the kth bottle; i = 1, 2, and 3 (levels of inclusion of additive), B0, B1, B2 = regression parameters of the model; Xi = effect of ith level of fixed quantitative factor (inclusion of additive); Ak(Pj) = random effect of incubation assuming Pj ~ N (0, Pj2); eijk = residual error, assuming eijk ~ N (0, σe2). As DFM doses were non-equidistant, orthogonal contrasts were obtained using the interactive matrix language (IML procedure). For all the analyses, differences detected at P ≤ 0.05 were considered significant, and differences at 0.05< P ≤ 0.10 were considered a tendency toward statistical significance.
The effect of DFM1 and DFM2 inclusion on in vitro rumen fermentation characteristics using a high-grain feedlot diet is presented in Table 2. After 24 h of fermentation, TGP tended to increase (P = 0.09) quadratically with increasing DFM1 dose. Corroborating these results, after 48 h of fermentation, TGP quadratically increased (P = 0.03) in response to DFM1 addition (Table 1). In vitro organic matter digestibility increased quadratically (P = 0.03) according to DFM1 inclusion, reflecting the gas production results. The addition of DFM1 at 1X increased IVOMD by 4.6 and 11.9% IVOMD compared with CON and 5X (P ≤ 0.01), respectively. Ammonia-N concentration tended to decrease quadratically (P = 0.09) by DFM1 inclusion, in which 1X dose decreased NH3-N levels by 50.4% and 27.0% when compared with CON and 5X, respectively. Other rumen fermentation variables such as pH, total VFA, VFA profile, and BCVFA were not affected by DFM1 (P > 0.05). On the other hand, DFM2 doses (P = 0.10) had no impact on any other fermentation variable evaluating a feedlot diet in this present study.
Table 2 Effect of direct-fed microbials (DFM) inclusion on in vitro ruminal fermentation variables1 in gas production system using a high-concentrate feedlot diet (Exp. 1).
The effect of DFM1 and DFM2 inclusion on in vitro rumen fermentation kinetics using a feedlot diet (Exp. 1) is presented in Table 3 and Figure 1. The TGP on the first and second pools (V1 and V2, respectively) had a quadratic increase and a tended to linearly reduction as DFM1 dose increased (P = 0.05 and P = 0.08, respectively). Control, 1X, and 5X of DFM1 reached the maximum gas volume of the first pool 6, 9, and 6 h after the beginning of the fermentation assay, respectively. The DFM1 at 1X had the greatest TGP on the first pool vs. CON and 5X, but on the second pool, lower TGP productions were detected for DFM1 at 1X and 5X vs. CON. Specific rates of digestion for the first and second pools (k1 and k2) reduced quadratically as DFM1 dose increased (P = 0.01 and trend P = 0.08, respectively). Only a linear decrease was detected for DFM2 on k2 specific rate (trend P = 0.07).
Table 3 Effect of additives inclusion on in vitro kinetic variables1 in gas production system using a feedlot diet (Exp. 1).
Figure 1 Effects of additives (DFM1 and DFM2) inclusion on in vitro gas production in Exp. 1 (feedlot diet).
Methane and CO2 production per IVDOM had a quadratic reduction according to DFM1 addition (P = 0.01 and trend P = 0.07, respectively; Figure 2). The DFM1 at 1X dose reduced CH4 production per IVDOM by 9.5 and 12.0% compared with CON and 5X, respectively. Regarding CO2 production per IVDOM, a 3.2 and 10.4% reduction was observed in 1X when compared with CON and 5X. The DFM2 supplementation did not affect GHG emission in Exp. 1.
Figure 2 Effects of additives (DFM1 and DFM2) inclusion on in vitro methane (CH4) and carbon dioxide (CO2) production per digestible organic matter (DOM) in Exp. 1 (feedlot diet). Symbol “Ƚ” indicates linear effect and symbol “Ɋ” indicates quadratic effect for levels of additive inclusion at P ≤ 0.05. Symbol “Ƚ*” indicates linear effect and symbol “Ɋ*” indicates quadratic effect for levels of additive inclusion at 0.05 > P< 0.10. The control treatment was used as having 0 mg level in both polynomial regressions (DFM1 and DFM2).
The effect of DFM1 and DFM2 inclusion on in vitro rumen fermentation characteristics using a dairy cow diet is presented in Table 4. Total GP at 24 h of incubation tended to increase (P = 0.06) quadratically by adding DFM1. Further, quadratic increases were detected for TGP 48 h and IVOMD (P = 0.03 and P = 0.002, respectively) with increasing doses of DFM1. Butyrate proportion decreased linearly as DFM1 increased (P = 0.05). Ammonia-N concentration had a quadratic decrease (P< 0.0001) according to DFM1 inclusion.
Table 4 Effect of direct-fed microbials (DFM) inclusion on in vitro ruminal fermentation variables1 in gas production system using a dairy cattle diet (Exp. 2).
The TGP at 48 h after incubation increased quadratically (P = 0.03) by DFM2 inclusion. The effect detected for TGP at 48 h reflected on IVOMD results, which had a similar quadratic increase (P = 0.0007). Propionate proportion tended to quadratically increase (P = 0.07) according to DFM2 inclusion, which also reflected the quadratic response on acetate to propionate ratio (P = 0.03). The highest propionate proportion and the lowest acetate to propionate ratio values were detected for DFM2 at 1X. Ammonia-N reduced quadratically (P = 0.0008) according to DFM2 addition, in which the lowest concentration was found with the 1X dose.
The effect of DFM1 and DFM2 inclusion on in vitro rumen fermentation kinetics using a lactating dairy cow diet is presented in Table 5 and Figure 3. The TGP on the first pool (V1) had a linear negative effect as DFM1 inclusion increased (P = 0.05). Control, 1X, and 5X reached the maximum gas volume of the first pool at 6, 8, and 6 h after the beginning of the fermentation, respectively. Although DFM1 at 1X had the greatest TGP, a linear negative effect was significant for these results. The TGP production on the second pool (V2) tended to show a linear negative response following DFM2 addition (P = 0.09).
Table 5 Effects of additive source and level of inclusion on in vitro kinetic variables1 in gas production system using a dairy cattle diet (Exp. 2).
Figure 3 Effects of additives (DFM1 and DFM2) inclusion on in vitro gas production in Exp. 2 (dairy cattle diet).
Methane and CO2 production per digestible organic matter (DOM) had a quadratic reduction following DFM1 (P = 0.01 and trend P = 0.07, respectively) and DFM2 addition (trend P = 0.08 and P = 0.02, respectively; Figure 4). The addition of DFM1 at 1X reduced CH4 production per IVDOM by approximately 15.7 and 6.5% compared with CON and 5X, respectively. Similarly, for CO2 production per DOM, DFM1 at 1X reduced CO2 production per DOM by 13.1 and 5.8% vs. CON and 5X, respectively. The DFM2 at 1X dose reduced CH4 production per IVDOM by 9.8 and 4.9% when compared with CON and 5X, respectively. Regarding CO2 (mmol/g IVDOM), DFM2 at 1X reduced CO2 production by approximately 10.5 and 3.8% when compared with CON and 5X, respectively.
Figure 4 Effects of additives (DFM1 and DFM2) inclusion on in vitro methane (CH4) and carbon dioxide (CO2) production per digestible organic matter (DOM) in Exp. 2 (dairy cattle diet). Symbol “Ƚ” indicates linear effect and symbol “Ɋ” indicates quadratic effect for levels of additive inclusion at P ≤ 0.05. Symbol “Ƚ*” indicates linear effect and symbol “Ɋ*” indicates quadratic effect for levels of additive inclusion at 0.05 > P< 0.10. The control treatment was used as having 0 mg level in both polynomial regressions (DFM1 and DFM2).
The effect of DFM1 and DFM2 inclusion on in vitro rumen fermentation characteristics using a forage-based diet is presented in Table 6. The TGP at 48 h showed a quadratic increase (P = 0.03) according to DFM1 addition; however, this effect was not observed at 72 h for TGP and IVOMD (P = 0.10 and P = 0.11, respectively). The pH value had a quadratic increase (P = 0.002) effect according to DFM1 inclusion, in which the 1X dose presented the highest value compared to CON and the 5X dose. Iso-valerate proportion increased and BCVFA concentration tended to increase linearly by DFM1 addition (P = 0.003 and P = 0.06, respectively). Ammonia-N reduced quadratically (P = 0.001) by increasing DFM1 doses, in which at 1X dose was detected the lowest NH3-N concentration in comparison with CON and 5X.
Table 6 Effects of direct-fed microbials (DFM) inclusion on in vitro ruminal fermentation variables1 in gas production system using a forage-based diet (Exp. 3).
The TGP at 24, 48, and 72 h after incubation had a quadratic increase with increasing doses of DFM2 (P = 0.08, P = 0.03, and P = 0.06, respectively). The TGP findings reflected on the IVOMD results, which also had a tendency of quadratic increase (P = 0.06) according to DFM2 addition. The BCVFA and NH3-N concentration had a tendency of quadratic increase and quadratic reduction, respectively (P = 0.09 and P = 0.06, respectively) with increasing doses of DFM2.
The effect of DFM1 and DFM2 inclusion on in vitro rumen fermentation kinetics using a forage-based diet is presented in Table 7 and Figure 5. There was no effect of either DFM1 or DFM2 inclusion on rumen fermentation kinetic parameters and CH4 and CO2 production (P > 0.10, Figure 6).
Table 7 Effects of additive source and level of inclusion on in vitro kinetic variables1 in gas production system using a forage-based diet (Exp. 3).
Figure 5 Effects of additives (DFM1 and DFM2) inclusion on in vitro gas production in Exp. 3 (forage-based diet).
Figure 6 Effects of additives (DFM1 and DFM2) inclusion on in vitro methane (CH4) and carbon dioxide (CO2) production per digestible organic matter (DOM) in Exp. 3 (forage-based diet). Symbol “Ƚ” indicates linear effect and symbol “Ɋ” indicates quadratic effect for levels of additive inclusion at P ≤ 0.05. Symbol “Ƚ*” indicates linear effect and symbol “Ɋ*” indicates quadratic effect for levels of additive inclusion at 0.05 > P< 0.10. The control treatment was used as having 0 mg level in both polynomial regressions (DFM1 and DFM2).
In recent years, dietary supplementation of cattle with direct-fed microbials (DFM) has emerged as a strategy to improve the efficiency of production, as well as to mitigate some of the environmental impacts of ruminant production systems. Particularly, Bacillus spp., Enterococcus faecium, and Saccharomyces cerevisiae have been shown to improve milk and meat production, reduce CH4 emission, inhibit potentially harmful bacteria, and hence, enhance digestive health in ruminants (Seo et al., 2010). The findings of our in vitro study indicate that DFM1 (E. faecium and S. cerevisiae) and DFM2 (B. licheniformis and B. subtilis) may modulate in vitro rumen fermentation characteristics in a diet-dependent manner. Although in vitro systems have some nuances compared to in vivo studies, such as the lack of VFA translocation through the ruminal epithelium, these systems have shown to be efficient in screening new technologies on ruminant nutrition, such as DFM’s, nutritional additives, and different diets (Vinyard and Faciola, 2022). Further, an in vitro system requires low investment compared to in vivo settings.
To the best of our knowledge, few studies have explored the potential of different DFM on ruminal fermentation traits of ruminants fed different diets based on corn silage, corn finely ground, and soybean meal. Jiao et al. (2021) studied the effects of several proportions of S. cerevisiae and E. faecium on in vitro rumen fermentation and reported different fermentation results according to the diet (high-grain or high-forage; based on barley silage and dry-rolled barley grain proportions) and media pH (5.8 and 6.5). For a high-forage diet, the DFM improved in vitro gas production at pH 6.5, but also increased DM disappearance and VFA concentration regardless of media pH. When a high-grain diet was evaluated, increased acetate along with a reduced propionate proportion was observed following the addition of the live yeast and bacteria combination on a pH 6.5, which, in turn, increased the acetate:propionate ratio. Additionally, for high-grain diets, greater NH3-N was observed when S. cerevisiae and E. faecium was added. Dhakal et al. (2023) reported slight differences in ruminal fermentation pattern when a blend of Lactobacillus animalis, Propionibacterium freudenreichii, B. licheniformis, and B. subtilis was incubated in an in vitro batch culture with corn silage and grass silage. After 3 h of fermentation, the DFM incubation improved total gas production of grass silage, but no differences were observed when a corn silage-based diet was used (Dhakal et al., 2023). Moreover, no DFM effect was observed on VFA and methane production, regardless of the diet (Dhakal et al., 2023).
In the present study, considering a feedlot (Exp. 1) and a dairy cow (Exp. 2) diet, a quadratic increase in TGP was detected after 24 and 48 h of fermentation for the combination of S. cerevisiae and E. faecium, at the lowest combination dose. Additionally, in a forage-based diet (Exp. 3), a quadratic increase of TGP was detected after 48 h of fermentation, at the lowest DFM inclusion. The combined effect of S. cerevisiae and E. faecium may have been effective in improving the ruminal environment leading to a better microbial activity and, hence, an improved nutrient degradation in vitro. Saccharomyces cerevisiae supplementation is recognized for positively modulating the rumen environment by supplying soluble growth factors for cellulolytic and amylolytic microbes (amino acids, organic acids, and B vitamins), inhibiting lactic acid accumulation, improving microbial protein synthesis, and increasing VFA concentration (Pinloche et al., 2013; Jiang et al., 2017; Dias et al., 2018; Amin and Mao, 2021). In addition, Enterococci (lactate-producing bacteria) may adapt some microbes to lactic acid as well as stimulate lactic acid-utilizing bacteria (Yoon and Stern, 1995; Seo et al., 2010). Further, Enterococci may change the microbial community abundance and composition of ruminal fluid despite of acting as a fumarate reductase-producing bacteria, in which fumarate that is an intermediate compound in the succinate–propionate pathway, plays an important role sinking H2 in the rumen, producing higher propionate, butyrate, and total VFA concentrations along with reducing CH4 concentration (Kim et al., 2016; Mamuad et al., 2019). Altogether, these effects may justify the findings detected herein for total gas production and DOM estimated from these outcomes. We can hypothesize that DFM1 at a lower dose can modulate the microbial community abundance and composition in the rumen, increasing the efficiency of nutrient use.
Increased fermentability of diets boost ammonia absorption and support increased microbial protein synthesis within the rumen (NASEM, 2016). As NH3-N reduced quadratically as DFM1 increased, we hypothesized that the microbial community may have benefited from DFM1 inclusion by increasing their microbial protein synthesis, improving N bioassimilation either in low, intermediate, or high forage inclusion diets. Therefore, reduced N losses may be expected from DFM1-supplemented ruminants at 1X dose both through urine and feces excretion, decreasing the environmental impact from N compounds. Interestingly, the highest DFM1 dosage was not effective in improving several rumen fermentation variables through different diets. These quadratic effects detected herein are important findings since we described the optimal biological dose of DFM1 that may improve animal performance taking into account the economic factor for its effective use on farms.
Improved nutrient digestibility, total VFA production, and microbial protein synthesis in the rumen have been reported for B. licheniformis and B. subtilis supplementation (Qiao et al., 2010; Sun et al., 2013; Pan et al., 2022; Cappellozza et al., 2023). In our study, DFM2 (B. licheniformis + B. subtilis) supplementation increased quadratically and linearly TGP of both cattle dairy diet and forage-based diet, respectively. Qiao et al. (2010) reported improved ruminal fiber digestion when supplemented B. licheniformis, which the authors justified these findings due to the provision of vitamins, glucose, lactate, malate, formate, succinate, and aspartate once lysis occurs by Bacillus cultures. These facts may explain the absence of DFM2 effect on high-grain diet total gas production since this diet does not depend on fiber digestion to improve its fermentability. Nonetheless, Bacillus spp. also produce several digestive enzymes, including proteases and amylases, that may be corroborated to the greater nutrient digestibility reported herein (Schallmey et al., 2004).
The VFA profile of the feedlot diet (Exp. 1) was not influenced by DFM’s supplementation. Otherwise, butyrate reduced linearly as DFM1 increased in the dairy cattle diet (Exp. 2). In addition, for this same diet, an increased quadratic effect was observed of DFM2 inclusion on propionate proportion along with quadratic reduction on acetate:propionate ratio. We have no explanation for butyrate reductions detected when DFM1 was supplemented in a dairy cattle diet; however, the ruminal fermentative pathway may have been modified by its inclusion through increased lactate-utilizing bacteria populations, in which H2 and CO2 may have been redirected to lactate and, hence propionate formation (Cao et al., 2011). A similar explanation may be given for the positive quadratic effect of DFM2 on propionate proportion in the dairy cattle diet; however, more research is needed to confirm such findings, as we did not measure lactate concentration as well as the microbiome modulation.
Regarding the forage-based diet, DFM1 inclusion increased linearly iso-valerate proportion and BCVFA concentration. In addition, BCVFA concentration had a quadratic positive effect as DFM2 was included. Branched-chained volatile fatty acids occur as a consequence of branch-chained amino acid deamination. Microbes responsible for cellulose and hemicellulose degradation in the rumen have recognized requirements for BCVFA (Andries et al., 1987; Terry et al., 2019). In this present study, DFM1 and DFM2 increased BCVFA, which may have impacted the increased gas production for both DFM’s in this diet scenario. Taking NH3-N and BCVFA concentrations together, it may be hypothesized that BCVFA may have been used as microbial energy source since NH3-N reduced as DFM1 and DFM2 increased.
For Exp. 1 (feedlot diet) the energy expenditure from the first pool (V1) was higher for DFM1 at 1X dose compared to control and DFM1 at 5X dose. But linear reduction was detected in the second pool (V2) as DFM1 increased. The specific rate of digestion reflected the findings of the V1 and V2 for this treatment, in which lower k1 and K2 were detected for DFM1 at 1X dose. These outcomes must be carefully interpreted since a lower rate of digestion does not mean inferior digestion, but the curve takes longer to change its slope, starting the V2 degradation. It seems that DFM1 at 1X improved the efficiency of nutrient extraction of the microbes since a higher TGP 48h was detected for this treatment in all experiments herein reported. It may be explained as earlier mentioned, the effects of S. cerevisae summed to the effects of E. faecium, DFM1 at a lower dose, can modulate the microbial community abundance of the rumen, increasing the efficiency of nutrient use. Regarding the DFM2 outcomes, increased k1 was detected for 1X dose, demonstrating faster slope change from V1 to V2 pool; however, for Exp. 1, no effects were detected on total gas production for this treatment. The DFM1 inclusion in the Exp. 2 (dairy cattle diet) at 1X mg/d dose was effective in improving the energy extraction from V1 compared to control and 5X mg/d. Otherwise, linear reduction was detected for DFM2 inclusion on the V2 pool. The kinetic variables may take the reader to a bias of interpretation since these outcomes must be interpreted as a measure in time. For example, we must interpret the time to change the slope curve from V1 to V2 pool. It cannot be interpreted as a gas production variable by itself, as these variables may not always match data of total gas production.
For Exp. 1 (feedlot diet), DFM1 inclusion reduced quadratically CH4 and CO2 emissions by DOM incubated. The same results were detected for the intermediate-grain diet. In addition, in the Exp. 2 (dairy cattle diet), DFM2 inclusion reduced quadratically CH4 and CO2 emissions by DOM incubated. The stoichiometric math involved in propionate formation through H2 available in the rumen cannot be used to explain the reduced CH4 detected for DFM1 inclusion for both high and intermediate-grain diets (Exp. 1 and 2). It is because propionate proportion was not influenced in these treatments. However, some studies compiled by Doyle et al. (2019) reported reduced CH4 emission through direct effect of lactate acid bacteria on methanogenesis through bacteriocins or due to the reduction of substrates available to methanogenesis provided by rumen microbes. Additionally, yeast cultures may reduce ruminal CH4 production by reducing protozoa numbers; by increasing butyrate or propionate production, and by promoting acetogenesis (Newbold et al., 1998). Corroborating, Chaucheyras et al. (1995) in an in vitro study demonstrated that Saccharomyces cerevisiae extracts increased hydrogen utilization by acetogens and decreased CH4 emission. However, as a weakness of this present study, the findings presented should be interpreted with caution because we did not evaluate several variables, reported herein from the literature, used to support our discussion.
Otherwise, the effect of DFM2 on CH4 emissions in the dairy cattle diet (Exp. 2) may be explained partially by the slight increase in propionate proportion detected. The production of propionate in the rumen competes with the production of methane for the hydrogen (H2) available. As a result, when propionate formation increases, methane production may decrease in a proportional manner (Pereira et al., 2022). As herein reported, this leads to a reduction in the ratio of acetate to propionate in the rumen (Krehbiel et al., 2003; Seo et al., 2010). The effect of Bacillus on rumen microbe modulation may be attributed to their bacitracin production, which is a broad-spectrum cyclopeptide antibiotic complex originally isolated from B. licheniformis and B. subtilis. Bacitracin exhibits a wide range of antimicrobial activity, rapid excretion rate, limited absorption in livestock, and the ability to block the cell wall synthesis of numerous gram-positive bacteria as well as certain gram-negative bacteria (Zhu et al., 2023). Russell and Strobel (1988) reported that the addition of bacitracin to an in vitro fermentation system using corn as the substrate led to an increase in propionate production. The study also found that bacitracin had similar effects to monensin on ruminal fermentation in vitro. Therefore, the changes in rumen fermentation observed in this study may be attributed to alterations in the microbial population of the rumen. Certain types of bacteria that produce lactate, butyrate, formate, and H2 were found to be susceptible to these compounds, while others involved in succinate production and lactate fermentation were not affected. As a result, the microbial community exhibited reduced production of acetate, butyrate, and methane, but increased production of propionate (Chen and Wolin, 1979; Dennis et al., 1981). The CO2 emission followed a similar pattern effect detected for CH4 emission, demonstrating a redirecting on metabolic pathways increasing other metabolites (propionate, for example) or just reducing its production according to DFM1 or DFM2 supplementation in the different diet types herein studied. Again, as a weakness of this present study, the findings presented should be interpreted with caution because we did not evaluate several variables reported herein from the literature, such as bacitracin production and microbial community profile among treatments, which were used to support our discussion.
Considering a feedlot diet (25% corn silage) or a dairy cattle diet (40.9% corn silage), the treatment DFM1 at dose 1X was effective in increasing TGP after 24 and 48 of fermentation process, which reflected in increased IVOMD. However, in a forage-based diet (100% grass hay), the increased TGP was only detected after 48h of fermentation process for the DFM1 treatment, which did not reflect on IVOMD. Otherwise, DFM2 increased TGP and IVOMD in both intermediate-grain (at 1X dose) and forage-based diets (at 5X dose). Regarding emission of greenhouse gases, DFM1 reduced CH4 and CO2 emission by DOM in both feedlot and dairy cattle diets; however, DFM1 inclusion did not affect CH4 and CO2 in a forage-based diet. Moreover, DFM2 only reduced CH4 and CO2 emission in a dairy cattle diet. Overall, DFM1 [Enterococccus faecium (5 × 109 CFU per g) + Saccharomyces cerevisiae (5 × 109 CFU per g)] and DFM2 [Bacillus licheniformis + Bacillus subtilis (3.2 × 109 CFU per g)] supplementation may improve ruminal fermentation and reduce GHG emissions in a dose-dependent manner, but the outcomes are dependent upon diet type.
The raw data supporting the conclusions of this article will be made available by the authors, without undue reservation.
The animal study was approved by Animal Use Ethics Committee of the Instituto de Zootecnia. The study was conducted in accordance with the local legislation and institutional requirements.
THS: Data curation, Formal analysis, Investigation, Methodology, Visualization, Writing – original draft, Writing – review & editing. BRA: Data curation, Formal analysis, Investigation, Methodology, Writing – review & editing. EM: Data curation, Formal analysis, Investigation, Methodology, Supervision, Validation, Visualization, Writing – review & editing. GWM: Formal analysis, Investigation, Methodology, Visualization, Writing – review & editing. HGR: Formal analysis, Investigation, Methodology, Visualization, Writing – review & editing. TT: Formal analysis, Investigation, Methodology, Visualization, Writing – review & editing. BIC: Resources, Writing – review & editing. RB: Conceptualization, Formal analysis, Funding acquisition, Project administration, Resources, Supervision, Validation, Visualization, Writing – review & editing, Data curation, Investigation, Methodology. EMP: Conceptualization, Data curation, Formal analysis, Funding acquisition, Investigation, Methodology, Project administration, Resources, Supervision, Validation, Visualization, Writing – original draft, Writing – review & editing.
The author(s) declare financial support was received for the research, authorship, and/or publication of this article. The authors gratefully acknowledge the Chr. Hansen A/S (Horsholm, Denmark) and the Sao Paulo Research Foundation (FAPESP; Grant# 2018/19743-7) for the funding support. And the Sao Paulo Research Foundation for providing a scholarship for the first author (FAPESP, Grant # 2022/11769-2).
Author BC was employed by the company Chr. Hansen A/S.
The remaining authors declare that the research was conducted in the absence of any commercial or financial relationships that could be construed as a potential conflict of interest.
The authors declare that this study received funding from Chr. Hansen A/S Horsholm, Denmark. The funder had the following involvement in the study: resources, writting (review and editing), visualization, and funding acquisition.
All claims expressed in this article are solely those of the authors and do not necessarily represent those of their affiliated organizations, or those of the publisher, the editors and the reviewers. Any product that may be evaluated in this article, or claim that may be made by its manufacturer, is not guaranteed or endorsed by the publisher.
Amin A. B., Mao S. (2021). Influence of yeast on rumen fermentation, growth performance and quality of products in ruminants: A review. Anim. Nutr. 7 (1), 31–415. doi: 10.1016/j.aninu.2020.10.005
Andries J. I., Buysse F. X., De Brabander D. L., Cottyn B. G. (1987). Isoacids in ruminant nutrition: their role in ruminal and intermediary metabolism and possible influences on performances - A review. Anim. Feed Sci. Technol. 18 (3), 169–180. doi: 10.1016/0377-8401(87)90069-1
AOAC (1990). “Official methods os analysis,” in Official Methods of Analysis, 15th editi. Ed. Helrich K. (Washington DC: Association of Official Analytical Chemists, Inc). doi: 10.7312/seir17116-004
Ballou M. A., Davis E. M., Kasl B. A. (2019). Nutraceuticals: an alternative strategy for the use of antimicrobials. Veterinary Clinics North America - Food Anim. Pract. 35 (3), 507–345. doi: 10.1016/j.cvfa.2019.08.004
Ban Y., Guan Le L. (2021). Implication and challenges of direct-fed microbial supplementation to improve ruminant production and health. J. Anim. Sci. Biotechnol. 12 (1), 1–225. doi: 10.1186/s40104-021-00630-x
Bernardeau M., Lehtinen M. J., Forssten S. D., Nurminen P. (2017). Importance of the gastrointestinal life cycle of bacillus for probiotic functionality. J. Food Sci. Technol. 54 (8), 2570–2584. doi: 10.1007/s13197-017-2688-3
Cao Y., Cai Y., Takahashi T., Yoshida N., Tohno M., Uegaki R., et al. (2011). Effect of lactic acid bacteria inoculant and beet pulp addition on fermentation characteristics and in vitro ruminal digestion of vegetable residue silage. J. Dairy Sci. 94 (8), 3902–3912. doi: 10.3168/jds.2010-3623
Cappellozza B. I., Joergensen J. N., Copani G., Bryan K. A., Fantinati P., Bodin J. C., et al. (2023). Evaluation of a Bacillus-based direct-fed microbial probiotic on in vitro rumen gas production and nutrient digestibility of different feedstuffs and total mixed rations. Trans. Anim. Sci. 7 (1), 1–8. doi: 10.1093/tas/txad044
Carro M. D., Lebzien P., Rohr K. (1992). Influence of yeast culture on the in vitro fermentation (Rusitec) of diets containing variable portions of concentrates. Anim. Feed Sci. Technol. 37 (3–4), 209–220. doi: 10.1016/0377-8401(92)90005-Q
Chaney A. L., Marbach E. P. (1962). Modified reagents for determination of urea and ammonia. Clin. Chem. 8, 130–132. doi: 10.1093/clinchem/8.2.130
Chaucheyras F., Fonty G., Bertin G., Gouet P. (1995). In vitro H2 utilization by a ruminal acetogenic bacterium cultivated alone or in association with an archaea methanogen is stimulated by a probiotic strain of saccharomyces cerevisiae. Appl. Environ. Microbiol. 61 (9), 3466–3467. doi: 10.1128/aem.61.9.3466-3467.1995
Chen M., Wolin M. J. (1979). Effect of monensin and lasalocid-sodium on the growth of methanogenic and rumen saccharolytic bacteria. Appl. Environ. Microbiol. 38 (1), 72–77. doi: 10.1128/aem.38.1.72-77.1979
Dennis S. M., Nagaraja T. G., Bartley E. E. (1981). Effects of lasalocid or monensin on lactate-producing or -using rumen bacteria. J. Anim. Sci. 52 (2), 418–426. doi: 10.2527/jas1981.522418x
Desnoyers M., Giger-Reverdin S., Bertin G., Duvaux-Ponter C., Sauvant D. (2009). Meta-analysis of the influence of saccharomyces cerevisiae supplementation on ruminal parameters and milk production of ruminants. J. Dairy Sci. 92, 1620 –11632. doi: 10.3168/jds.2008-1414
Dhakal R., Copani G., Cappellozza B. I., Milora N., Hansen H. H. (2023). The effect of direct-fed microbials on in-vitro rumen fermentation of grass or maize silage. Fermentation 9 (4). doi: 10.3390/fermentation9040347
Desnoyers M., Giger-Reverdin S., Bertin G., Duvaux-Ponter C., Sauvant D. (2009). Meta-analysis of the influence of Saccharomyces cerevisiae supplementation on ruminal parameters and milk production of ruminants. J. Dairy Sci. 92, 1620–1632. doi: 10.3168/jds.2008-1414
Dias A. L. G., Freitas J. A., Micai B., Azevedo R. A., Greco L. F., Santos J. E. P. (2018). Effect of supplemental yeast culture and dietary starch content on rumen fermentation and digestion in dairy cows. J. Dairy Sci. 101 (1), 201–221. doi: 10.3168/jds.2017-13241
Dias B. G. C., Santos F. A. P., Meschiatti M., Brixner B. M., Almeida A. A., Queiroz O., et al. (2022). Effects of feeding different probiotic types on metabolic, performance, and carcass responses of bos indicus feedlot cattle offered a high-concentrate diet. J. Anim. Sci. 100, skac289. doi: 10.1093/jas/skac289
Doyle N., Mbandlwa P., Kelly W. J., Attwood G., Li Y., Ross R.P., et al. (2019). Use of lactic acid bacteria to reduce methane production in ruminants, a critical review. Front. Microbiol. 10 (October). doi: 10.3389/fmicb.2019.02207
Food and Agricultural Organization of the United Nations and World Health Organization (FAO/WHO) (2001). Probiotics in food: health and nutritional properties of probiotics in food including powder milk with live lactic acid bacteria. World Health Organization. Available at: https://www.fao.org/3/a0512e/a0512e.pdf.
Jiang Y., Ogunade I. M., Qi S., Hackmann T. J., Staples C. R., Adesogan A. T. (2017). Effects of the dose and viability of saccharomyces cerevisiae. 1. Diversity of ruminal microbes as analyzed by illumina miSeq sequencing and quantitative PCR. J. Dairy Sci. 100 (1), 325–342. doi: 10.3168/jds.2016-11263
Jiao P. X., Ma F. C., Beauchemin K. A., Alzahal O., Xie X. L., Yang W. Z. (2021). Effect of mixed live yeast and lactic acid bacteria on in vitro fermentation with varying media Ph using a high-grain or high-forage diet. Can. J. Anim. Sci. 101 (2), 370–380. doi: 10.1139/cjas-2020-0138
Kim S. Ho, Mamuad L. L., Kim D. W., Kim S. Ki, Lee S. S. (2016). Fumarate reductase-producing enterococci reduce methane production in rumen fermentation in vitro. J. Microbiol. Biotechnol. 26 (3), 558–665. doi: 10.4014/jmb.1512.12008
Krehbiel C. R., Rust S. R., Zhang G., Gilliland S. E. (2003). Bacterial direct-fed microbials in ruminant diets : performance response and mode of action. J. Anim. Sci. 81 (October 2014), 120–132. doi: 10.2527/2003.8114_suppl_2E120x
Lettat A., Nozière P., Silberberg M., Morgavi D. P., Berger C., Martin Cécile (2012). Rumen microbial and fermentation characteristics are affected differently by bacterial probiotic supplementation during induced lactic and subacute acidosis in sheep. BMC Microbiol. 12. doi: 10.1186/1471-2180-12-142
Lim H., Oh S., Yu S., Kim M. (2021). Isolation and characterization of probiotic bacillus subtilis Mkhj 1-1 possessing l-asparaginase activity. Appl. Sci. (Switzerland) 11 (10), 1–105. doi: 10.3390/app11104466
Mamuad L. L., Kim S. Ho, Biswas A. A., Yu Z., Cho K. K., Kim S. B., et al. (2019). Rumen fermentation and microbial community composition influenced by live enterococcus faecium supplementation. AMB Express 9 (1). doi: 10.1186/s13568-019-0848-8
Menke K. H., Steingass H. (1988). Estimation of the energetic feed value obtained from chemical analysis and in vitro gas production using rumen fluid. Anim. Res. Dev. 28, 7–55.
National Academies of Sciences, Engineering, and Medicine (NASEM). (2021). Nutrient Requirements of Dairy Cattle: Eighth Revised Edition. Washington, DC: The National Academies Press. doi: 10.17226/25806
National Academies of Sciences, Engineering, and Medicine (2016). Nutrient Requirements of Beef Cattle: Eighth Revised Edition. (Washington, DC: National Academies Press). doi: 10.17226/19014
National Academies of Sciences, Engineering, and Medicine (2021). Nutrient requirements of dairy cattle: Eighth revised edition (Washington, DC: The National Academies Press). doi: 10.17226/25806
Newbold C. J., McIntosh F. M., Wallace R. J. (1998). Changes in the microbial population of a rumen-simulating fermenter in response to yeast culture. Can. J. Anim. Sci. 78 (2), 241–244. doi: 10.4141/A97-086
Nocek J. E., Kautz W. P., Leedle J. A. Z., Block E. (2003). Direct-fed microbial supplementation of dairy cattle during the transition period. J. Dairy Sci. 86 (1), 331–335. doi: 10.3168/jds.S0022-0302(03)73610-8
Pan L., Harper K., Queiroz O., Copani G., Cappellozza B. I. (2022). Effects of a bacillus-based direct-fed microbial on in vitro nutrient digestibility of forage and high-starch concentrate substrates. Trans. Anim. Sci. 6 (2), 1–95. doi: 10.1093/tas/txac067
Pereira A. M., Dapkevicius M. de L. N., Borba A. E.S. (2022). Alternative pathways for hydrogen sink originated from the ruminal fermentation of carbohydrates: which microorganisms are involved in lowering methane emission? Anim. Microbiome 4 (1), 1–125. doi: 10.1186/s42523-021-00153-w
Pinloche E., McEwan N., Marden J. P., Bayourthe C., Auclair E., Newbold C. J. (2013). The effects of a probiotic yeast on the bacterial diversity and population structure in the rumen of cattle. PLoS One 8 (7). doi: 10.1371/journal.pone.0067824
Qiao G. H., Shan A. S., Ma N., Ma Q. Q., Sun Z. W. (2010). Effect of supplemental bacillus cultures on rumen fermentation and milk yield in chinese holstein cows. J. Anim. Physiol. Anim. Nutr. 94 (4), 429–436. doi: 10.1111/j.1439-0396.2009.00926.x
Russel J. B., Strobel H. J. (1989). Effect of lonophores on ruminal fermentation. Appl. Environ. Microbiol. 55 (1), 1–65. doi: 10.1128/aem.55.1.1-6.1989
Russell J. B., Strobel H. J. (1988). Effects of additives on in vitro ruminal fermentation: A comparison of monensin and bacitracin, another gram-positive antibiotic. J. Anim. Sci. 66 (2), 552–558. doi: 10.2527/jas1988.662552x
Schallmey M., Singh A., Ward O. P. (2004). Developments in the use of bacillus species for industrial production. Can. J. Microbiol. 50 (1), 1–175. doi: 10.1139/w03-076
Schofield P., Pitt R. E., Pell A. N. (1994). Kinetics of fiber digestion from in vitro gas production. J. Anim. Sci. 72 (11), 2980–2991. doi: 10.2527/1994.72112980x
Seo Ja K., Kim S.-w., Kim M. H., Upadhaya S. D., Kam D. K., Ha J. K. (2010). Direct-fed microbials for ruminant animals. Asian-Australasian J. Anim. Sci. 23 (12), 1657–1675. doi: 10.5713/ajas.2010.r.08
Shridhar P. B., Amachawadi R. G., Tokach M., Patel I., Gangiredla J., Mammel M., et al. (2022). Whole genome sequence analyses-based assessment of virulence potential and antimicrobial susceptibilities and resistance of enterococcus faecium strains isolated from commercial swine and cattle probiotic products. J. Anim. Sci. 100 (3), 1–105. doi: 10.1093/jas/skac030
Sun P., Wang J. Q., Deng L. F. (2013). Effects of bacillus subtilis natto on milk production, rumen fermentation and ruminal microbiome of dairy cows. Animal 7 (2), 216–222. doi: 10.1017/S1751731112001188
Tagliapietra F., Cattani M., Bailoni L., Schiavon S. (2010). In vitro rumen fermentation: effect of headspace pressure on the gas production kinetics of corn meal and meadow hay. Anim. Feed Sci. Technol. 158 (3–4), 197–201. doi: 10.1016/j.anifeedsci.2010.04.003
Tagliapietra F., Cattani M., Hansen H. H., Bittante G., Schiavon S. (2013). High doses of vitamin E and vitamin C influence in vitro rumen microbial activity. Anim. Feed Sci. Technol. 183 (3–4), 210–214. doi: 10.1016/j.anifeedsci.2013.05.010
Tagliapietra F., Cattani M., Hansen H. H., Hindrichsen I. K., Bailoni L., Schiavon S. (2011). Metabolizable energy content of feeds based on 24 or 48h in situ NDF digestibility and on in vitro 24h gas production methods. Anim. Feed Sci. Technol. 170 (3–4), 182–191. doi: 10.1016/j.anifeedsci.2011.09.008
Terry S. A., Badhan A., Wang Y., Chaves A. V., McAllister T. A. (2019). Fibre digestion by rumen microbiota — a review of recent metagenomic and metatranscriptomic studies. Can. J. Anim. Sci. 99 (4), 678–925. doi: 10.1139/cjas-2019-0024
van Soest P. J., Robertson J. B., Lewis B. A. (1991). Methods for dietary fiber, neutral detergent fiber, and nonstarch polysaccharides in relation to animal nutrition. J. Dairy Sci. 74 (10), 3583–3975. doi: 10.3168/jds.S0022-0302(91)78551-2
Vinyard J. R., Faciola A. P. (2022). Unraveling the pros and cons of various in vitro methodologies for ruminant nutrition: A review”. Trans. Anim. Sci. 6 (4), 1–95. doi: 10.1093/tas/txac130
Wang K., Nan X., Chu K., Tong J., Yang L., Zheng S., et al. (2018). Shifts of hydrogen metabolism from methanogenesis to propionate production in response to replacement of forage fiber with non-forage fiber sources in diets in vitro. Front. Microbiol. 9 (NOV). doi: 10.3389/fmicb.2018.02764
Wang H., Yu Z., Gao Z., Li Q., Qiu X., Wu F., et al. (2022). Effects of compound probiotics on growth performance, rumen fermentation, blood parameters, and health status of neonatal holstein calves. J. Dairy Sci. 105 (3), 2190–22005. doi: 10.3168/jds.2021-20721
Williams P. E., Tait C. A., Innes G. M., Newbold C. J. (1991). Effects of the inclusion of yeast culture (Saccharomyces cerevisiae plus growth medium) in the diet of dairy cows on milk yield and forage degradation and fermentation patterns in the rumen of steers. J. Anim. Sci. 69 (7), 3016–3026. doi: 10.2527/1991.6973016x
Yáñez-Ruiz D. R., Bannink A., Dijkstra J., Kebreab E., Morgavi D. P., O’Kiely P., et al. (2016). Design, implementation and interpretation of in vitro batch culture experiments to assess enteric methane mitigation in ruminants-a review. Anim. Feed Sci. Technol. 216, 1–18. doi: 10.1016/j.anifeedsci.2016.03.016
Yoon I. K., Stern M. D. (1995). Influence of DFM on ruminal fermentation adn performance of ruminants.Pdf. AJAS 8 (6), 533–555. Available at: https://www.animbiosci.org/upload/pdf/8-80.pdf.
Keywords: digestibility, direct-fed microbials, methane, ruminant, sustainability
Citation: Silva TH, Amâncio BR, Magnani E, Meurer GW, Reolon HG, Timm TG, Cappellozza BI, Branco RH and Paula EM (2024) Evaluation of direct-fed microbials on in vitro ruminal fermentation, gas production kinetic, and greenhouse gas emissions in different ruminants’ diet. Front. Anim. Sci. 5:1320075. doi: 10.3389/fanim.2024.1320075
Received: 11 October 2023; Accepted: 02 January 2024;
Published: 23 January 2024.
Edited by:
James Levi Klotz, United States Department of Agriculture, United StatesReviewed by:
Brittany Davis, Agricultural Research Service (USDA), United StatesCopyright © 2024 Silva, Amâncio, Magnani, Meurer, Reolon, Timm, Cappellozza, Branco and Paula. This is an open-access article distributed under the terms of the Creative Commons Attribution License (CC BY). The use, distribution or reproduction in other forums is permitted, provided the original author(s) and the copyright owner(s) are credited and that the original publication in this journal is cited, in accordance with accepted academic practice. No use, distribution or reproduction is permitted which does not comply with these terms.
*Correspondence: Eduardo M. Paula, emarostegandepaula@gmail.com
Disclaimer: All claims expressed in this article are solely those of the authors and do not necessarily represent those of their affiliated organizations, or those of the publisher, the editors and the reviewers. Any product that may be evaluated in this article or claim that may be made by its manufacturer is not guaranteed or endorsed by the publisher.
Research integrity at Frontiers
Learn more about the work of our research integrity team to safeguard the quality of each article we publish.