- 1School of Veterinary Medicine and Biomedical Sciences, University of Nebraska, Lincoln, NE, United States
- 2Departamento de Producción Agrícola y Animal, División de Ciencias Biologicas y de la Salud (CBS), Universidad Autónoma Metropolitana, Unidad Xochimilco, Ciudad de México, Mexico
In areas where the ambient temperature is above the thermoneutral zone, the economic loss from animals for human consumption, such as pigs, is worrying. Heat Stress (HS) increases body temperature and causes a subsequent reduction in feed intake. This reduction results in the loss of essential nutrients, such as amino acids, which are crucial for growth rate and the activation of vital biochemical mechanisms. However, the effects of HS on reactive oxygen species (ROS) generation, and the activation of mechanisms such as the antioxidant system associated with nuclear factor erythroid-derived 2-like-2 (Nrf2), energy balance, protein synthesis and mitochondrial biogenesis have received limited attention. In this work, we provide a comprehensive review of the existing evidence regarding the significance of nutritional components and their association with HS. We particularly emphasize ROS generation, mitochondrial alterations, and the activation of Nrf2, AMP-activated protein kinase (AMPK), and mammalian target of rapamycin (mTOR) signaling pathways in response to the disruption of homeostasis.
Introduction
Environmental fluctuations associated with climate change occur seasonally in diverse geographic regions (https://www.climate.gov). Heat Stress (HS), in tropical countries and regions with harsh summers plays a significant global role in the productive performance of economically valuable animals (Renaudeau et al., 2011). Each year, the global human population experiences exponential growth. This results in escalating demand for safe, sustainable food consumption to ensure food security. Agricultural products provide approximately 80% of food in developed countries. In poorer and developing countries, the establishment of highly productive animal husbandry industries, especially swine (Figure 1A), is promoted. This has been advocated by the FAO in the 2030 agenda for sustainable development, making swine one of the most affordable proteins worldwide. Regions such as Asia exhibit a significant dominance in the production share of pigs, followed by Europe, the Americas, Africa, and Oceania (Figures 1B, C). Notably, countries such as China and the United States of America take the lead among the top 10 global pork producers (Figure 1D), proving to be an industry with economic viability, even so, the pig industry faces significant annual financial losses, estimated in millions of dollars, attributed to the impacts of climate change at different stages of growth and physiological stage in which they are (St-Pierre et al., 2003; Pollman, 2010; Mayorga et al., 2019; Ramirez et al., 2022).
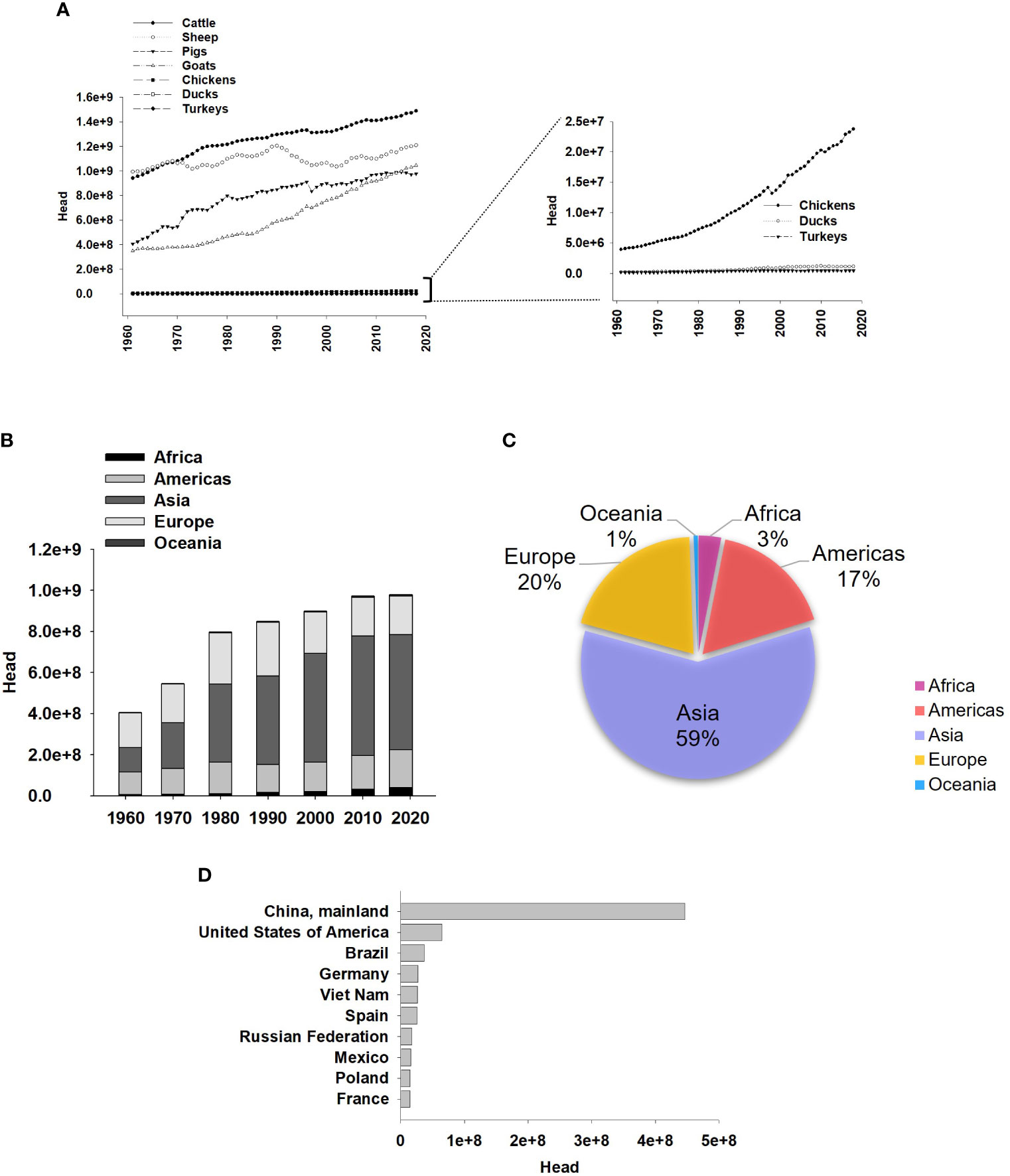
Figure 1 Pig production worldwide. (A) Global Animal Production from 1961-2018, with pigs ranking as the third most produced group of animals. Right graph in A represent the same data, from the corresponding left graphs, but magnified for better visualization. (B) Pig production by year (1961- 2018, every 10 years) and region. (C) Percentage of pig production by region 2000-2018. (D) Top 10 Countries for Pig Production from 2000 to 2018. FAOSTAT.org.
HS occurs when animals are exposed to conditions that exceed their thermoneutral zone. Elevated core temperature during HS leads to a decrease in voluntary feed intake and alters the metabolism of nutrients, resulting in reduced energy availability. Additionally, HS negatively impacts immunity, endocrine functions, electrolyte balance, blood pH, microbiota composition, and intestinal epithelium. Furthermore, it leads to increased circulating levels of cortisol and corticosterone (Morrow-Tesch et al., 1994; Chou et al., 2012; Pearce et al., 2013; Sanz Fernandez et al., 2015; Akbarian et al., 2016). Some of these alterations can lead to disruptions in the reproductive cycle, decreased semen production quality, impaired growth performance due to reduced feed efficiency, altered carcass composition characterized by increased lipid deposition and decreased protein accretion, and an increase in mortality rates (Pollman, 2010; Ross et al., 2015).
While the physiological impacts of HS are well understood, further exploration of the molecular mechanisms activated during thermal stress could contribute to enhancing outcomes in animal production. Recent studies have demonstrated that HS disrupts energy metabolism (Ma et al., 2019) and protein synthesis (Ganesan et al., 2018) in pigs. In this sense, the regulation of energy availability and protein synthesis could be a key answer. AMP-activated protein kinase (AMPK) and mammalian target of rapamycin (mTOR) plays an important role in cell homeostasis. However, one of the initial responses to the disruption of homeostasis is the generation of reactive oxygen species (ROS) and subsequent induction of oxidative stress. This process triggers the activation of various signaling cascades, including nuclear factor erythroid-derived 2-like-2 (Nrf2), AMPK, and mTOR.
In this review, our primary focus is on elucidating the physiological processes elicited by HS in pigs, specifically the perturbations to homeostasis arising from diminished feed intake and restricted nutrient availability. We discuss the generation of reactive ROS, the response of the antioxidant system regulated by Nrf2, mitochondrial alterations, and the activation of metabolic signaling pathways such as AMPK and mTOR.
Effects of heat stress in pigs production
Environmental variations play an important role in the physiological effects of economically important animals. Changes in the physiology of pigs exposed to HS are associated with the loss of the thermoneutral zone that affects multiple life stages, including reproduction, gestation, lactation, and growth efficiency (Christon, 1988; Lucy and Safranski, 2017). In certain instances, the effects of HS are severe enough to result in premature mortality, which represents a substantial economic loss (Ross et al., 2015). HS occurs when the animal’s ability to dissipate body heat is compromised, leading to detrimental effects on its health. The interaction between solar radiation intensity, variations in humidity, and ambient air velocity (Figure 2A) becomes critical in exacerbating the effects of HS (Council, 1981). Pigs, being homeothermic animals, possess a limited ability to dissipate body heat due to the absence of functional sweat glands and the presence of substantial subcutaneous adipose tissue layers (D'Allaire et al., 1996), producing a significant amount of metabolic heat. HS induces a redistribution of blood flow in the pigs’ system, primarily towards the peripheral tissues, resulting in intestinal hypoxia and inflammation. This is followed by a decrease in intestinal functionality and integrity (Pearce et al., 2013). In this context, cytosolic structures and proteins, including tight junctions, occludins, claudins, heat shock proteins (HSP), and hypoxia-inducible factor (HIF), are susceptible to compromise (Pearce et al., 2015). The modification of these complexes is associated to changes in intestinal permeability, leading to elevated levels of blood endotoxemia markers and subsequent immune responses (Hall et al., 2001; Gabler et al., 2018). The effects of HS extend beyond cellular and physiological disruptions that impair animal growth. They also compromise carcass yield, carcass fat deposition, and intramuscular fat content (Pearce et al., 2011). Additionally, HS affects meat quality, as evidenced by changes in pH, water holding capacity, and meat color (Ma et al., 2015), which can lead to decreased consumer acceptance.
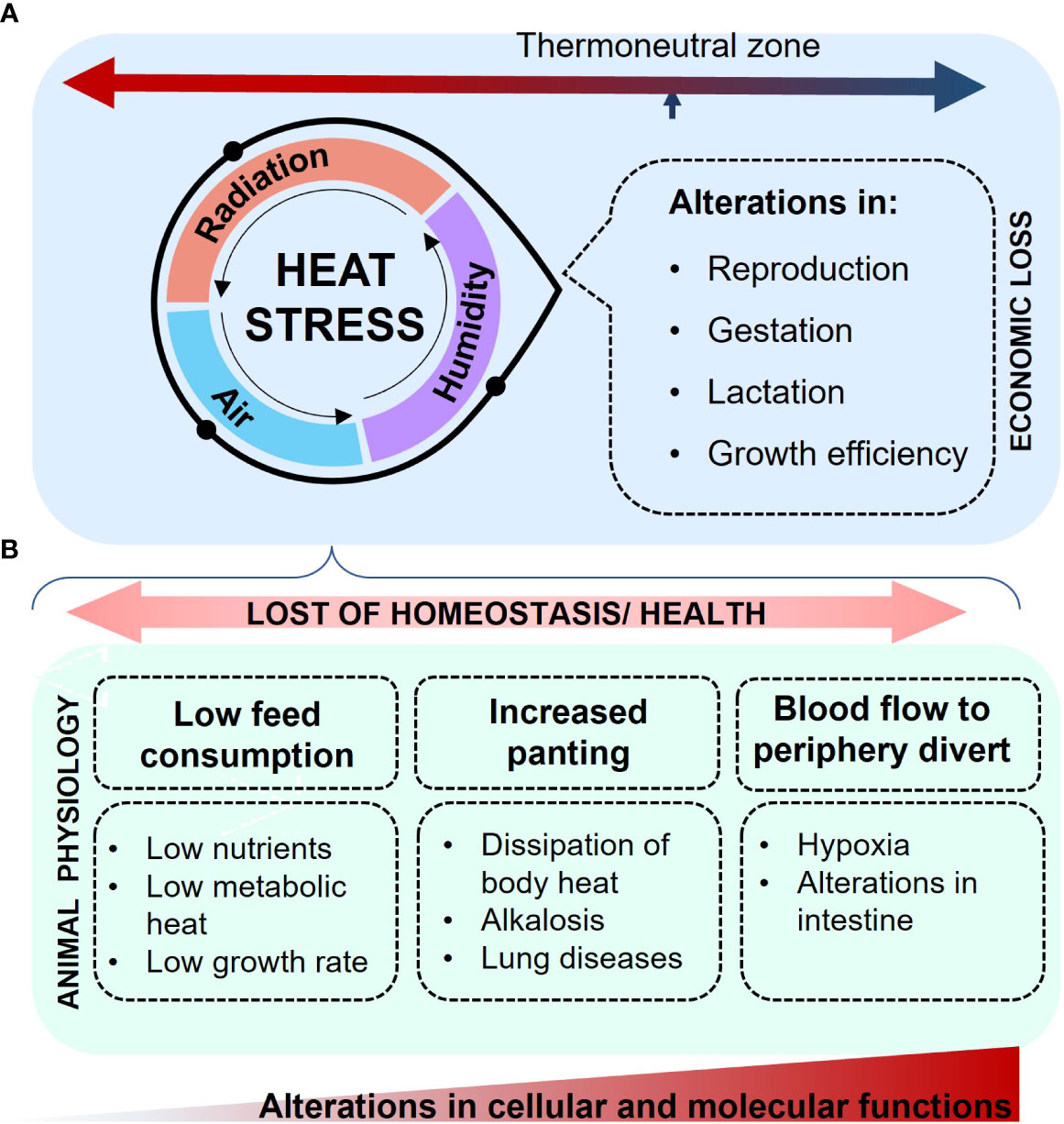
Figure 2 Effects of HS in pigs. (A) The combination of humidity, radiation, and increased air temperature intensifies the state of HS, primarily impacting reproduction, gestation, lactation, and growth efficiency, thereby leading to economic loss. The resultant loss of homeostasis impairs molecular and cellular functions, with effects that become apparent in the overall animal physiology. (B) Decreased feed consumption, alongside peripheral blood diversion and increased panting, leads to insufficient nutrient acquisition, compromised intestinal integrity, and alkalosis.
One of the initial biological responses that pigs have developed to counteract the effects of HS is a reduction in feed intake by 20-40%, which results in a decrease in metabolic heat production (Huynh et al., 2005; Cervantes et al., 2018; Morales et al., 2014; Morales et al., 2016a), the reduction in feed intake is associated with a decrease in the growth rate (Renaudeau et al., 2010). Additionally, there is an increase in respiration rate as a response to the dissipation of body heat (Vilas Boas Ribeiro et al., 2018), and this response is contingent upon the thermal load and heat dissipation characteristics of the environment (Quiniou et al., 2000; Quiniou et al., 2001). Panting is the primary response for dissipating heat (Fuquay, 1981). However, hyperventilation can induce a decrease in carbon dioxide levels in the blood (Figure 2B), leading to respiratory alkalosis (Bartko et al., 1984). This modification can affect diverse physiological and biochemical processes due to the consequent increase in lactate production (Davies et al., 1986; Lindinger et al., 1990). Respiratory alkalosis and pH alterations can result from a primary physical condition. This alteration can predispose an individual to the entry of other pathological agents. Additionally, it can also be secondary, serving as a compensatory response to an adjacent disease, which may or may not affect the respiratory system (Gordon et al., 1999). Nevertheless, the shorth incorporation of vitamin E in dietary formulations partially alleviated respiratory alkalosis (Liu et al., 2016; Liu et al., 2018).
Nutrient availability in pigs exposed to heat stress
The dietary formulations developed for animals exposed to HS aim to maintain a proper balance of amino acids (AA), proteins, carbohydrates, and lipids (Kerr et al., 2003). Under thermoneutral conditions, proteins in pigs are hydrolyzed by proteolytic enzymes such as stomach pepsin, trypsin, chymotrypsin, pancreatic elastase, and intestinal membranous and cytosolic enzymes (He et al., 2016). This hydrolysis breaks down proteins, releasing AA that are subsequently absorbed through active transport mechanisms in the small intestine (García-Villalobos et al., 2012; Morales et al., 2013; Kiela and Ghishan, 2016). The AA then reach the liver via the portal vein circulation, where metabolic processes begin, involving the synthesis or degradation of proteins and AA (Paulusma et al., 2022). In this complex process, the profile of essential AA (Lys, Met, and Thr) and non-essential AA (Arg, Gly, Cys, Glu, Gln) not only play a critical role in the animal’s diet and metabolic utilization but also have a fundamental impact on intestinal health (Yang and Liao, 2019). Additionally, the association between nutrient availability in diets, metabolism, and the negative effects on intestinal performance under thermoneutral conditions has been reported. Nonetheless, the supplementation of proteins and AA (Lys, Thr, Trp, Met, Ile, Val, and Phe) in diets has improved carbohydrate and lipid metabolism as well as tissue structures in the gastrointestinal tract (Ren et al., 2014). However, certain AA such as Arg, His, Ile, Leu, Met, Phe, Thr, Trp, and Val, have been identified as crucial for metabolism (Wu, 2009). Moreover, the addition of the “HS” factor further impacts intestinal integrity, particularly in the small intestine (Figure 3), leading to alterations in digestive and nutrient absorption capacity and increasing susceptibility to infections (Pearce et al., 2012; Pearce et al., 2014; Gabler and Pearce, 2015). In this regard, the strategy of reducing food consumption to mitigate body/metabolic heat also results in a decrease in the intake of nutrients such as proteins and AA (Baumgard and Rhoads, 2013). Adding extra protein-bound or free AA to the diet can potentially compensate for the reduced AA intake; however, it is important to note that extra protein-bound AA may further increase the body heat load, whereas the addition of free AA does not impact the heat load (Le Bellego et al., 2002; Kerr et al., 2003; Morales et al., 2018). This was corroborated in data analyzed in serum, demonstrating that dietary supplementation with additional free AA (Arg, Ile, Asp, Glu, Gly, Ser) can help rectify the decreased availability of AA and growth rate observed in pigs subjected to HS (24.5-42.6°C, Morales et al., 2019). Similarly, in regions characterized by extreme summers, even minor diurnal ambient temperature variations (30-41°C) can alter the availability of free AA (Cervantes et al., 2017). Consequently, the addition of essential AA has been meticulously calculated to optimize digestibility and maintain nutritional balance, especially when protein content in the diet is reduced (Morales et al., 2019).
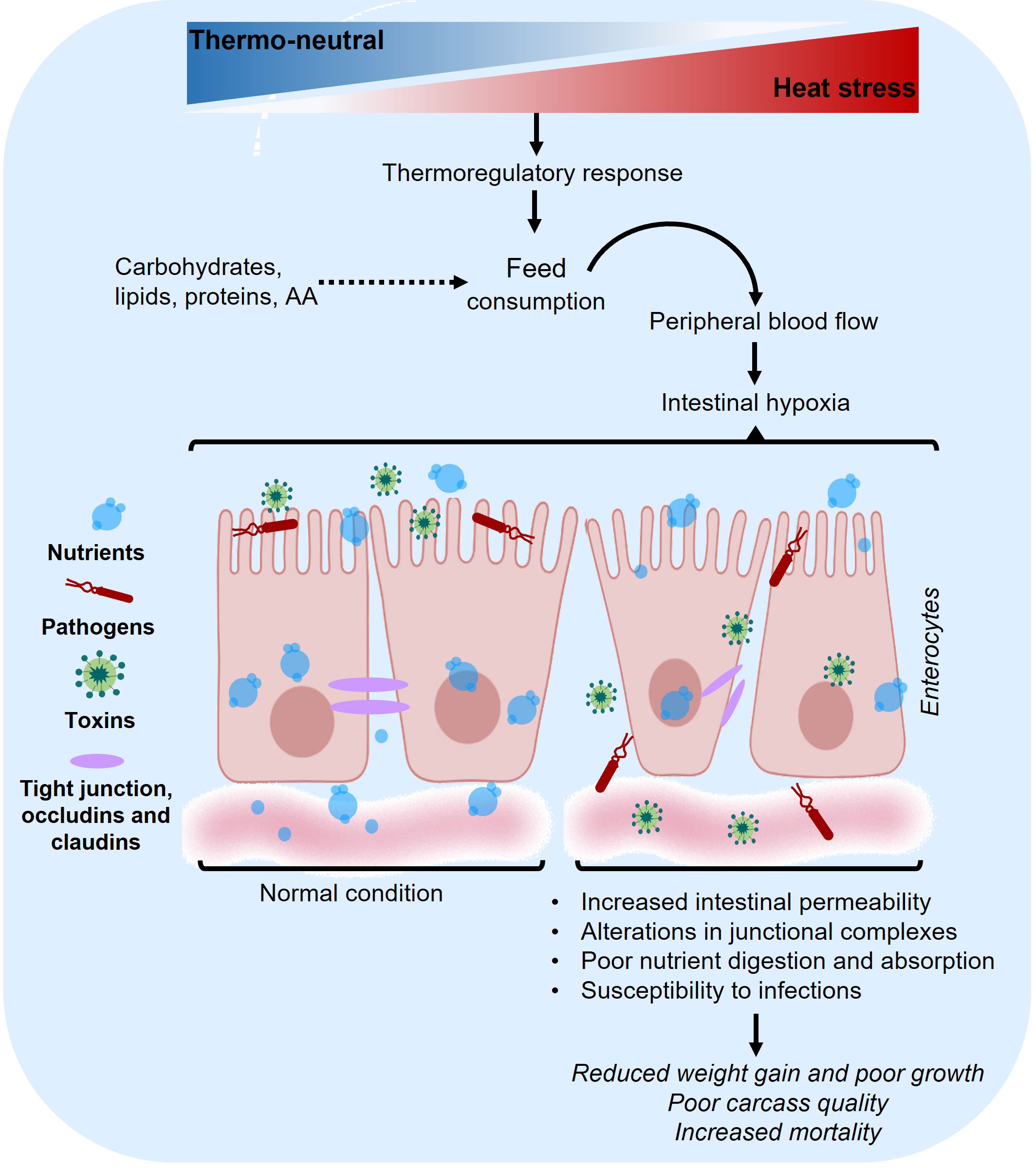
Figure 3 Impacts of heat stress on the small intestine. HS disrupts homeostasis, leading to alterations in molecular and cellular functions. These changes are observable in the overall physiology of the animal. Image of epithelium designed with biorender.com.
Additionally, the reduction in feed intake of up to 40% due to HS has been demonstrated to induce alterations in the surface area of the intestinal epithelium (Pearce et al., 2012). This was observed in Chinese mini pigs exposed to a temperature of 40°C, 5 hours each day over a period of 10 days. The intestinal mucosa, as well as the duodenum and jejunum, began showing signs of damage on the third day of exposure, which gradually repaired over the subsequent six days. This damage was associated with the expression of the Epidermal Growth Factor signaling pathway (Liu et al., 2009). Growing pigs exposed to acute HS conditions (35°C, 24 hours) exhibited a decrease in the integrity of the intestinal tract (specifically the ileum and colon). Additionally, there was an increase in circulating endotoxins as measured in serum, along with an elevation in intestinal glucose transport and blood glucose levels (Pearce et al., 2012). Exposure to HS conditions (31-37°C) in the initial 24 hours can induce increased intestinal cell death, leading to enhanced endogenous intestinal loss of proteins and AA (Arg, Thr) in growing pigs (Morales et al., 2016a). Similarly, growing pigs exposed to HS (24-45°C, 7 days) showed differential effects on the digestive and AA (Arg, His) absorption capacity of the ileum (Morales et al., 2016b).
A healthy gut is crucial for the metabolism of the nutrients, physiological activities, body wellbeing, and production efficiency in different stages of pigs life (Pluske et al., 2018). Thus far, it has been established that feeding pigs subjected to HS with low-protein diets supplemented with free AA mitigates intestinal epithelial damage and enhances nutrient absorption (Morales et al., 2020). The gastrointestinal tract maintains a close relationship with the epithelial mucosa, microbiota, and the nutrients from the pig’s diet. This system represents approximately 20% of the body’s energy expenditure, given that the microbiota hosts around 100 microbial species, is associated with more than 20 hormonal types, and is involved in the digestion and absorption of a vast array of nutrients (Choct, 2009). As of now, the microbiota is recognized as a critical vector for host development, influencing aspects of anatomy, physiology, and metabolism (Sommer and Bäckhed, 2013). Weaning remains one of the most critical stages in animal physiology due to the various biochemical changes the animals undergo. Dietary formulations play a crucial role in ensuring their survival and proper development during this phase (Andretta et al., 2014; Velayudhan et al., 2015). During this stage, and even in growing, gestational, and finishing pigs exposed to HS, alterations in gut microbiota (factors such as epithelial integrity, colonization by pathogenic bacteria, and morphological changes, including variations in villus and microvillus height) composition are observed (Xiong et al., 2020; Patra and Kar, 2021; Xiong et al., 2022). These changes are part of an adaptive mechanism in response to the stress condition (He et al., 2019; Xiong et al., 2020). Several studies have demonstrated that HS effectively alters the fecal microbiota of pigs (He et al., 2019; Xiong et al., 2020; Hu et al., 2022); however, the role this change plays in mitigating the effects of HS has yet to be clearly defined (Le Sciellour et al., 2019). A more comprehensive understanding of the physiological roles of characterized microbial populations could represent a novel strategy to mitigate the effects of HS in pigs.
Molecular response to heat stress
Generally, the physiological changes in pigs exposed to HS have been characterized and described previously. Events such as the generation of oxidative stress have received considerable attention at the molecular and cellular levels due to the activation of various signaling pathways (Liu et al., 2016; Lauridsen, 2019). Consequently, alterations at the intestinal level might constitute an initial response to significant physiological events triggered by an imbalance in homeostasis.
Heat stress and ROS production
Reactive oxygen species (ROS) generation is a typical response to practically all stress factors, including HS. ROS are derived from oxygen metabolism and are by-products of cellular respiration. They can be classified into two groups: free radicals, with one or more unpaired electrons; and non-radicals, where two free radicals share their unpaired electrons (Lushchak, 2015). The high reactivity of ROS is attributed to the unpaired electrons in their structure; these can interact with biologically significant macromolecules, such as nucleic acids, carbohydrates, lipids, and proteins, thus modifying their functions (Cecarini et al., 2007). Under normal conditions, the generation of ROS helps modulate various cellular functions, including cell survival, cell death, differentiation, signaling, metabolism, and inflammation (Halliwell and Whiteman, 2004; Zhang et al., 2016). Minor and transient increases in ROS can be tolerated, but substantial increases are linked with cellular and systemic biological damage, typically as a response to the disruption of homeostasis.
Previous reports have documented the production of ROS under conditions of HS (Flanagan et al., 1998; Vacca et al., 2004). Upon exposure to a temperature of 32°C for eight days, hepatic and skeletal muscle tissues of swine demonstrated physiological modifications in their lipid and carbohydrate metabolic pathways (Sanz Fernandez et al., 2015). These two metabolic pathways are notably vulnerable to disruption due to interactions with ROS. It is well established that the diverse growth stages of pigs and the varying cell types elicit differential responses to HS. Hepatic cells from pigs in the finishing phase, subjected to a temperature of 30°C for three weeks, exhibited an escalated oxidative response. This was associated with immune responses, apoptosis, metabolic alterations, signal transduction, and cytoskeletal changes (Cui et al., 2016). These findings provide evidence that the liver participates in a spectrum of molecular adaptive responses linked to stress events.
At the cellular level, the first response to HS (39-45°C) is the elevation of the production of superoxide anion (O2•-) in the mitochondria, owing to the univalent reduction to superoxide (O2) and hydrogen peroxide [H2O2] (Loschen and Azzi, 1975). H2O2 are produced by dismutation catalyzed by superoxide dismutase (SOD) in animal cells (Mujahid et al., 2005; Mujahid et al., 2007; Azad et al., 2010; Li et al., 2017). As a first response of the antioxidant system, catalase (CAT) can break down H2O2 into oxygen (O2) and water (Deisseroth and Dounce, 1970; Nandi et al., 2019). Glutathione peroxidase (GPx) catalyzes the reduction of H2O2 to H2O, in the oxidation reaction of glutathione reduced (GSH) to glutathione oxidized (GSSG). GSSG is reduced back to GSH by glutathione reductase (GR) which catalyzes the oxidation of nicotinamide adenine dinucleotide phosphate (NADPH) to nicotinamide adenine dinucleotide phosphate (NADP+), forming a redox cycling, Figure 4A (Valko et al., 2007; Lu, 2013). Separately, in the mitochondria, NADH produced during cellular metabolism is oxidized by NADH dehydrogenase (Complex I). Electrons from this reaction then move through the Electron Transport Chain [ETC] (Li et al., 2017), eventually passing through ubiquinol-cytochrome c reductase (Complex III), contributing to the proton gradient used to produce adenosine triphosphate (ATP). In this sense, the redox balance (oxide/reduction) and the antioxidant system responses play a crucial role in the modulation of HS. In pigs, the modulation of this system has already been reported in skeletal muscle at 37°C for short periods of time, specifically, two hours (Volodina et al., 2017).
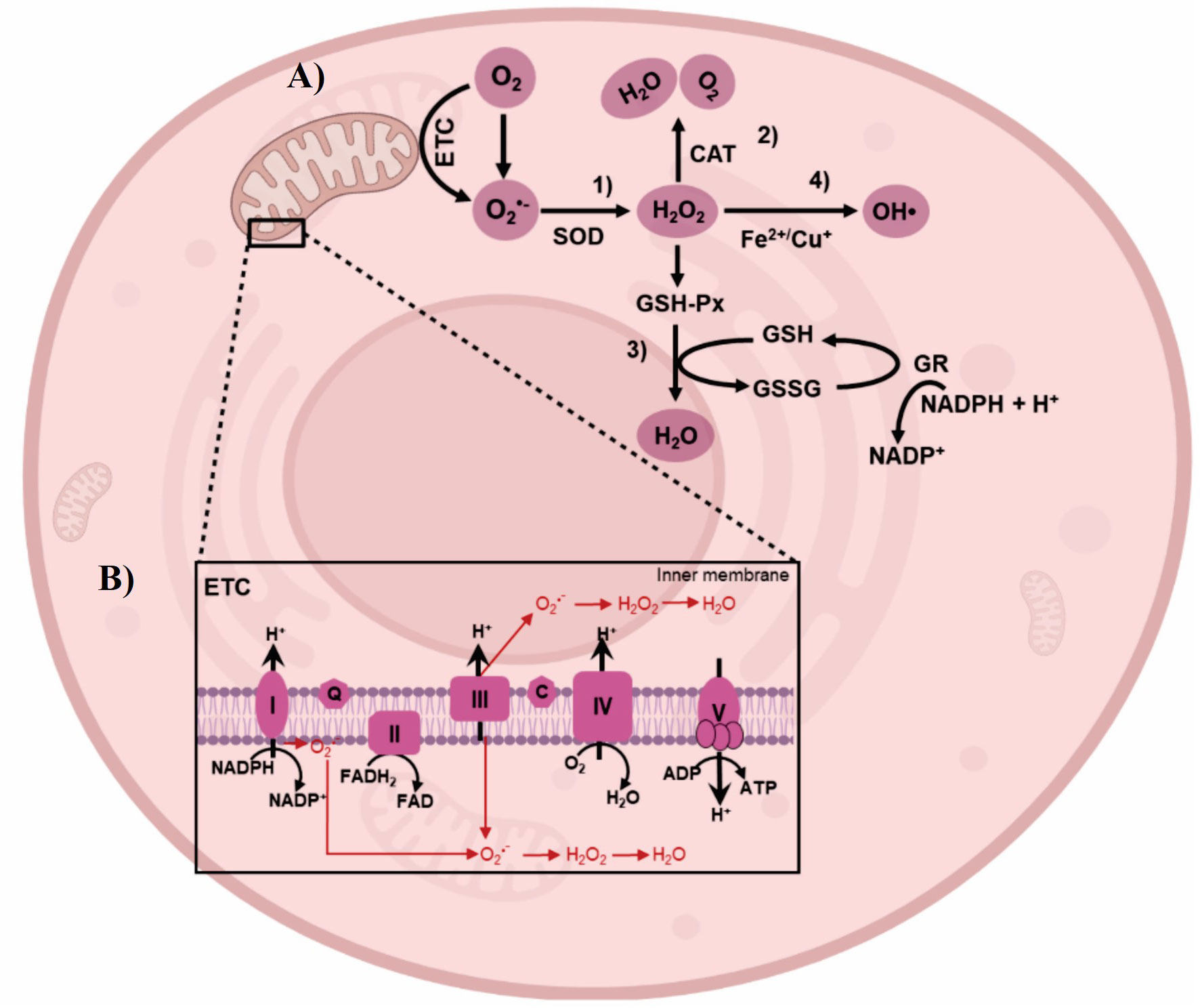
Figure 4 ROS generation by HS and detoxification by antioxidant system. (A) Electrons interact with superoxide (O2) to produce superoxide anion (02•-). 1) Hydrogen peroxide (H2O2) are produced by dismutation catalyzed by superoxide dismutase (SOD). 2) Catalase (CAT) can decompose H2O2 into molecular oxygen (O2) and water (H2O). 3) Glutathione peroxidase (GPx) catalyzes the reduction of H2O2 to H2O, through the oxidation reaction of glutathione reduced (GSH) to glutathione oxidized (GSSG). GSSG is subsequently reduced back to GSH by glutathione reductase (GR) which catalyzes the oxidation of nicotinamide adenine dinucleotide phosphate (NADPH) to nicotinamide adenine dinucleotide phosphate (NADP), thereby forming a redox cycle. 4) H2O2 is converted to hidroxil radical (HO•) by intervention of Fe2+/Cu+. (B) HS leads to ROS generation in the complex I and Ill of Electron Transport Chain (ETC). Image of cell designed with biorender.com.
Free radicals such as O2•- can be easily produced in NADPH oxidase, xanthine oxidase and mitochondria. Mitochondria play a crucial role in cellular energy maintenance through the production of ATP via chemical reactions occurring in the ETC. The complex I and III of the ETC is the primary site of ROS production (Figure 4B), where the electrons interact with O2 to produce O2•-, and spontaneously or enzymatically is converted to H2O2 and hydroxyl radical [HO•] (Murphy, 2009). Increased production of these radicals can lead to mitochondrial dysfunction, associated to a dramatic drop in ATP levels during HS (Patriarca and Maresca, 1990). Few studies have been carried out associated with the composition of the diets of animals exposed to HS and the alterations at the mitochondrial level caused by ROS generation. In Longissimus dorsi muscle, ETC gene expression was differentially affected by dietary phosphorous levels of pigs exposed to 32°C, 24 hours (Weller et al., 2013). These data provide evidence of physiological and biochemical adaptations to HS associated with an increase in ROS.
Research focusing primarily on the “HS” factor appears to be more abundant compared to studies that consider the “diet-HS-oxidative stress” interplay. In this context, mitochondrial alterations appear to be a key mechanism little associated. As shown by proteomic analyses in the intestines of pigs exposed to chronic HS (30°C, three weeks) highlighted the significance of the down-regulation of proteins involved in the tricarboxylic acid cycle and ETC (Cui and Gu, 2015). This study suggests direct alterations in energy metabolism during chronic HS. The findings also indicated that the antioxidant system might control the production of ROS, potentially mitigating the onset of oxidative stress during chronic HS. Moreover, other studies have documented changes in markers of autophagy and mitophagy as a result of ROS production, following exposure to HS (35°C for 1-3 days) in porcine skeletal muscle (Brownstein et al., 2017; Volodina et al., 2017). Along the same lines, pigs exposed to 37°C, 24 hours, have been shown to induce mitochondrial dysfunction and stimulate the antioxidant response, as evidenced by increased expression of SOD and CAT; this HS also triggered apoptosis and autophagic signaling processes in the skeletal muscle (Ganesan et al., 2017). The mitochondrial metabolic flexibility, as indicated by various markers in skeletal muscle, has been found to decrease after exposure to high temperature (35°C) for 7 days, leading to an accumulation of adipose tissue during HS (Zhao et al., 2018). These findings shed light on the multifaceted impact of HS on cellular mechanisms, including energy production, antioxidant defense, and cellular recycling processes.
When the production of ROS exceeds a certain threshold, but before the onset of full-fledged oxidative stress, various signaling pathways within the cell are activated. These pathways function as a protective response, aiming to restore cellular homeostasis and prevent further damage. As observed in skeletal muscle of sows exposed to HS at 37°C, 24 hours (Montilla et al., 2014). In this study, along with the observed increase in ROS production and indications of mitochondrial involvement, the initiation of an antioxidant response (CAT, CuZnSOD and MnSOD) was detected. Additionally, the expression of the nuclear factor-κB (NF-κB), known to be activated by various stimuli, including HS, did not elicit an inflammatory response. These responses are typically more evident in muscle cells due to their high mitochondrial content. Although the observed responses occurred over extended periods, it would be intriguing to investigate how such rapid responses remain active over prolonged periods.
Antioxidant system and thermotolerance
Oxidative stress is defined as the imbalance between an increase in ROS levels and the cell’s ability to neutralize them via the antioxidant system and the repair/turnover mechanism (Birben et al., 2012). Oxidative stress is associated with economic losses due to decreased performance parameters such as feed intake, growth, milk production, and egg laying, as well as increased mortality rates. These detrimental effects are particularly significant in various livestock including dairy cows, dairy heifers, beef cows, finishing cattle (Mirzad et al., 2018; Sigdel et al., 2019), sows and pigs (Cui et al., 2019; Hao et al., 2021), broilers, layers, and turkeys (St-Pierre et al., 2003; Ratriyanto and Mosenthin, 2018). In this sense, the antioxidant system as a mechanism to counter oxidative stress in pigs, has been little studied. Different pathways appear to be particularly relevant mechanisms to study due to their rapid responses to various stressors. Nrf2 it would seem to be the indicated route due to its rapid response to any stress (Glory and Averill-Bates, 2016; Surai et al., 2019). Nrf2 is a master transcription factor that regulate more than 200 genes, mediated expression of phase I and II detoxified and antioxidant enzymes (Nguyen et al., 2009). Nrf2 in the cytoplasm is associated to Keap1 (Kelch like ECH-associated protein 1) protein (Figure 5), where Nrf2 require the decoupling of the complex for the antioxidant system to be activated (Kim and Keum, 2016). The antioxidant system is characterized by the expression of different enzymes, however, SOD, CAT and GSH are the first response molecules. SOD usually are founded in the cytoplasm in three isoforms, manganese (Mn)-SOD in mitochondria, iron (Fe)-SOD in extracellular space and copper-zinc (Cu-Zn)-SOD in cytosol (Canada and Calabrese, 1989; Asakura and Kitahora, 2018). CAT another dismutase enzyme, exists as tetramer composed of 4 identical monomers each one with heme group at the active site (Birben et al., 2012). Is widely expressed in the cytoplasm and peroxisomes (Kehrer et al., 2010). GSH, a tripeptide composed of glycine, cysteine, and glutamic acid, is typically found in the cytoplasm and acts as an endogenous component of cellular metabolism (Gad, 2014). The enzymatic regulation of the GSH redox cycle is critical for maintaining intracellular levels in a reduced state, which is essential for preventing oxidative damage and preserving cellular integrity (Murray et al., 2006). For instance, in a study where Bama miniature pigs were exposed to HS at 40°C for eight days, their liver cells demonstrated a high capacity for heat tolerance. This was accomplished through the activation of the Nrf2 pathway, in addition to an increase in antioxidant enzymes such as SOD, CAT, GSH, and GPx (Chen et al., 2017). Acute exposure to HS conditions (35°C, 1-3 days) in sows has been found to be regulated by the expression of antioxidant enzymes in mitochondria, including Mn-SOD and CAT, and by the activation of the NF-κB and Tumor Necrosis Factor α (TNF-α) pathways in the semitendinosus muscle (Montilla et al., 2014). Furthermore, even short-term exposure to HS (37°C, 2-6 hours) resulted in increased protein abundance and activity of SOD and CAT, which could be linked to skeletal muscle dysfunction (Volodina et al., 2017).
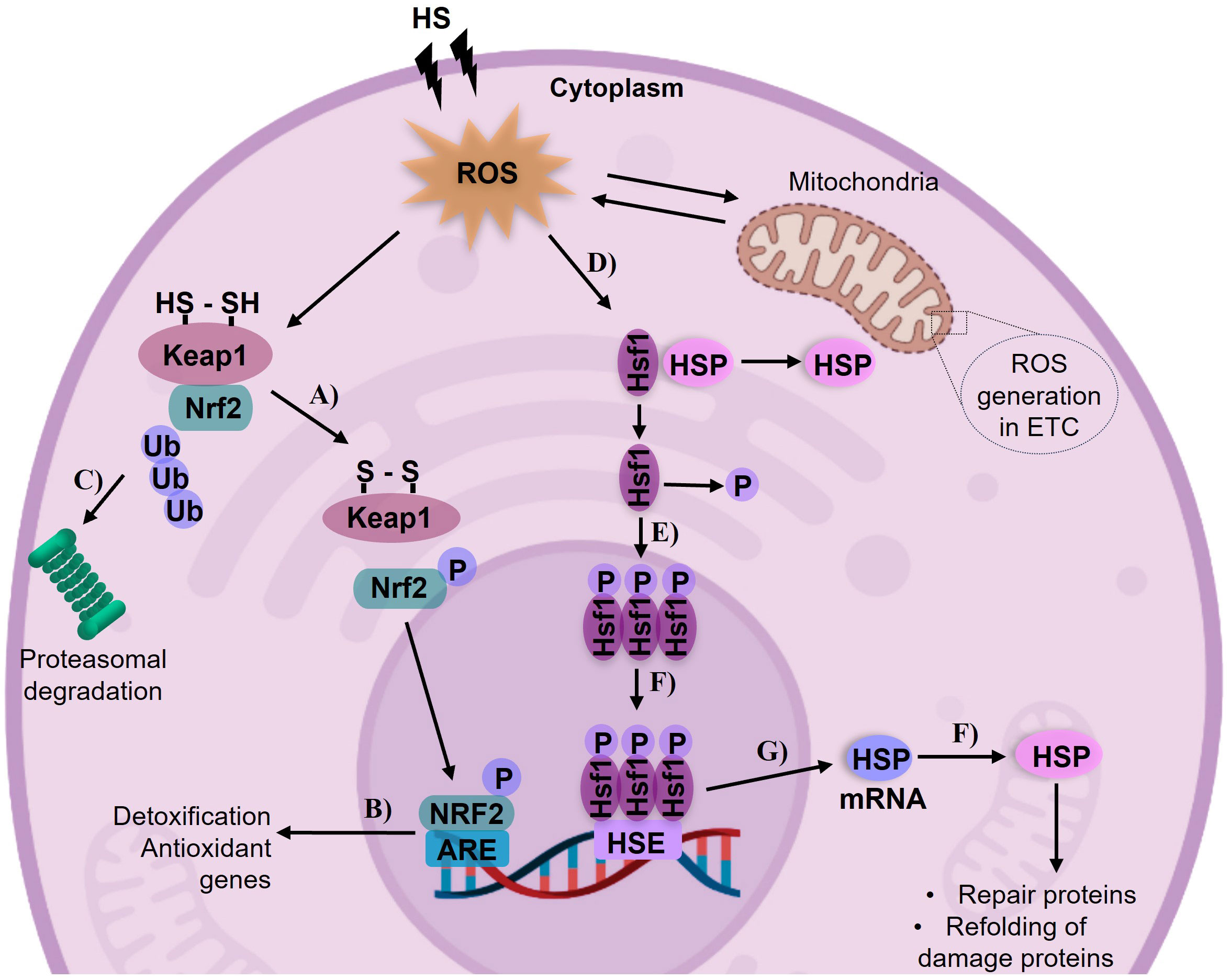
Figure 5 Activation of the antioxidant system by HS and its modulation by the Nrf2 and HSP pathways. Mitochondria is the main source of ROS generation. (A) In the cytoplasm, the Nrf2 transcription factor dissociates from Keapl in response to increased ROS levels. (B) Nrf2 translocate to the nucleus and activates detoxification genes involved in antioxidant defense. (C) ln the absence of dissociation of the Nrf2-Keapl complex, it undergoes degradation in the proteasome. (D) Heat shock factor 1 (Hsfl) forms an inactive complex with Heat Shock Proteins (HSPs), when exposed to HS as a stressor, the Hsfl-HSP complex dissociates. (E) Hsfl undergoes phosphor ylation and forms trimers in the cytosol. (F) The Hsfl trimer enters the nucleus and binds to Heat Shock Elements (HSEs) in the promoter region of HSP genes. (G) This leads to the transcription of HSP mRNA, and then translated into proteins. (H) The synthesized HSPs facilitate the repair and refolding of denatured proteins. Image of cell designed with biorender.com.
The generation of ROS by HS and the swift activation of the antioxidant system typically initiate numerous reactions, including those linked to thermotolerance. In this process, chaperone proteins play a crucial role in supervising molecular events such as post-translational modifications and transcriptional mechanisms (Kregel, 2002). The reaction of HSP is a ubiquitous phenomenon. Their expression can be triggered rapidly, even by slight increases in HS (Barbe et al., 1988; Ritossa, 1996). HSP expression is implicated in disruptions to metabolic processes and is essential for the synthesis, folding, and assembly of other proteins (Pelham, 1985). Many of the morphological and phenotypic effects of HS can be attributed to protein aggregation (Richter et al., 2010). In the cytoplasm, during HS (acute or chronic), HSP dissociates from Heat Shock Factor (Hsf1) binding, Hsf1 translocates to the nucleus and recognizes the Heat Shock Element a specific sequence in DNA (Figure 5), initiating the assembly of the transcription machinery (Walter and Buchner, 2002), this pathway could be considered one of the first lines of defense (Page et al., 2006). These proteins are upregulated in response to minimizing the accumulation of denatured or abnormal proteins within the cell (Hassan et al., 2019). In cultured porcine muscle satellite cells, HSP70 mRNA expression was reported following 48 hours of exposure to HS at 40.5°C (Kamanga-Sollo et al., 2011). In vivo, a study involving the ileum and colon of pigs, a linear increase in HSP expression was observed between 2-6 hours at 37°C. This response was associated with alterations in feed consumption and intestinal integrity (Pearce et al., 2014). Furthermore, in the liver of growing pigs exposed to HS conditions ranging from 29.5°C to 37.2°C for 21 days, a similar response was observed (Montesinos-Cruz et al., 2019). The response of these molecules has been extensively studied under prolonged times and different formulations in diets (Gan et al., 2013; Wu et al., 2013).
Studies have indicated that the inclusion of synthetic phytochemical antioxidants in swine nutrition might provide a means to counterbalance the consequences of oxidative stress (Lee et al., 2017), inclusive of those induced by HS. Under thermoneutral conditions, selenium-deficient, high-energy swine diets have been observed to suppress the Nrf2 pathway in neutrophils, whilst simultaneously enhancing phagocytosis in cases of chronic oxidative stress (Yang et al., 2017). However, the mechanistic interplay between selenium deficiency and high-energy diet in modulating the activation of the Nrf2 pathway remains unclear. Conversely, the supplementation of resveratrol in sow and piglet diets, also under thermoneutral conditions, has shown to ameliorate the antioxidant system regulated by the Nrf2-Keap1 axis, observed 20 days post-breeding (Meng et al., 2018). The supplementation of L-Met and DL-Met in piglet diets, maintained at temperatures of 27.5 to 25°C over a three week period, yielded analogous outcomes in the expression of the antioxidant Nrf2 system (as evidenced by mRNA levels) in relation to gut morphology (Zeitz et al., 2019). In summary, the link between Nrf2 antioxidant system activation, thermotolerance-HSP, dietary formulations, and heat stress in pigs remains an active research area.
Energy metabolism. AMPK-mTOR role
AMP-activated protein kinase (AMPK), an evolutionarily conserved serine/threonine kinase, serves a critical function as a primary regulator and detector of cellular energy status (Carling et al., 2011). Its activation is involved in the regulation of protein, lipid, and glucose metabolism (Figure 6A), as well as mitochondrial biogenesis (Kahn et al., 2005; Wang et al., 2012). The AMPK complex is heterotrimeric, consisting of a catalytic α subunit and two regulatory subunits, β and γ. It presents two α-subunit isoforms (α1 and α2), two β-subunit isoforms (β1 and β2), and three γ-subunit isoforms (γ1, γ2, γ3); the phosphorylation of the Thr172 residue within the α-subunit is crucial for its activation (Hardie, 2007). The phosphorylation of AMP is a direct activator of AMPK under conditions such as exercise, ischemia, hypoxia, low glucose, HS, ROS, or any form of cellular stress that disrupts or depletes ATP levels (Hardie, 2004; Kahn et al., 2005; Yang et al., 2018; Yu et al., 2018). The phosphorylation or allosteric activation of AMPK results in the downregulation of ATP-consuming anabolic processes and upregulation of catabolic processes that generate ATP, thereby maintaining the AMP : ATP ratio. In other words, AMPK activation plays a positive role in regulating signaling pathways that replenish cellular ATP reserves (such as fatty acid oxidation and autophagy), and conversely inhibits ATP-consuming biosynthetic processes, like gluconeogenesis, lipid synthesis, and protein synthesis (Mihaylova and Shaw, 2011).
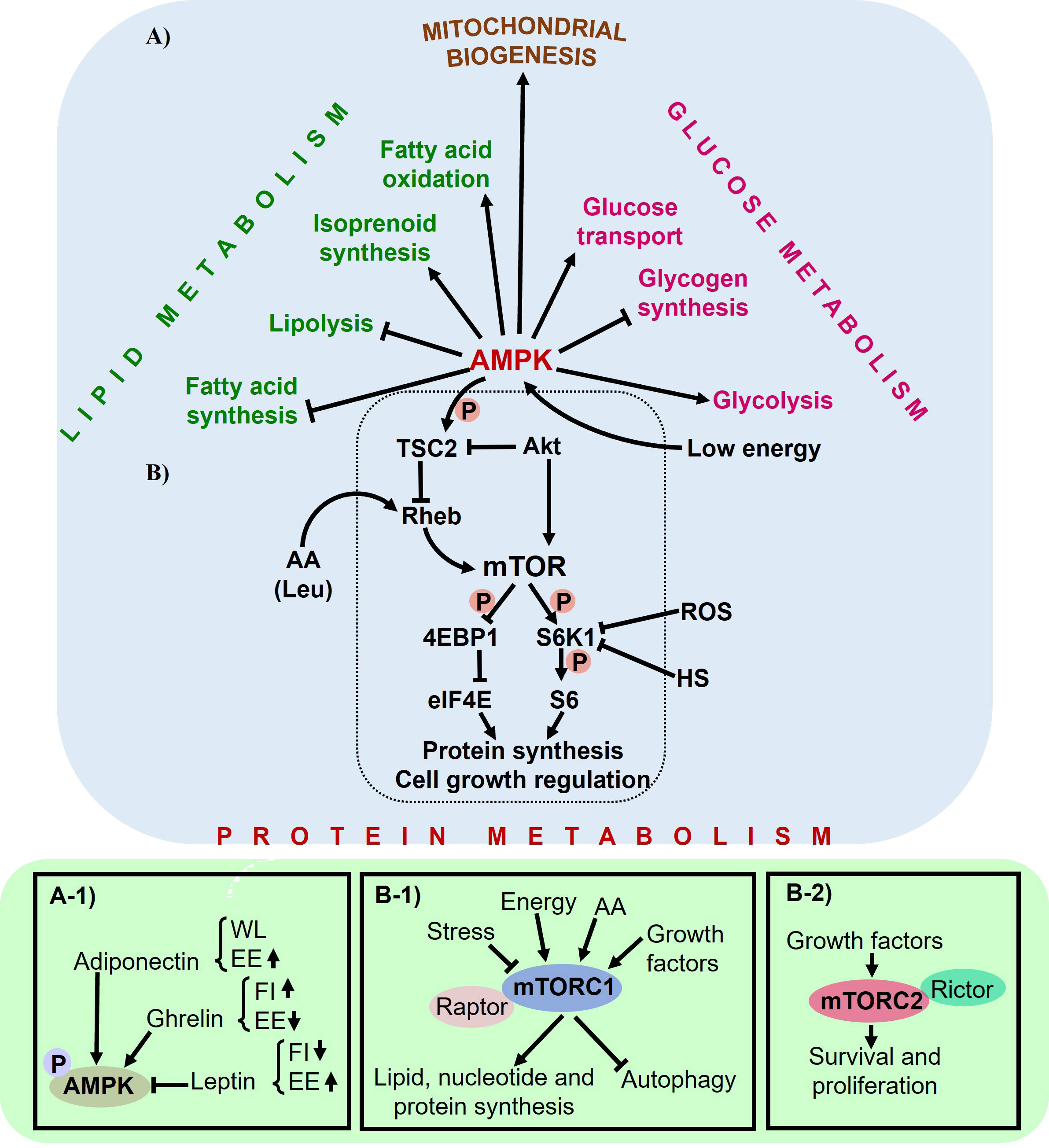
Figure 6 The role of AMPK as a regulator of metabolism. (A) AMPK regulates lipid, glucose, and protein metabolism, as well as mitochondrial biogenesis, through numerous downstream targets. (A-1) AMPK also plays a role in hypothalamic regulation of feed consumption, affecting parameters such as feed intake (Fl), energy expenditure (EE), and weight loss (\VL). (B) Low cellular energy levels activate AMPK, which inhibits TSC2 function. mTOR promotes growth and protein synthesis by regulating S6K and 4EBP1. HS and ROS decrease S6K activity. Leucine (Leu) may enter the mTOR pathway at the level or upstream of Rheb. (B-1) mTORCl and (B-2) mTORC2 act as both inducers and targets in the pathway.
AMPK activation as a response to energy depletion
Feed intake (nutrients), energy balance, and body weight are under the regulation of the central nervous system (CNS). The CNS plays a pivotal role in the control and coordination of energy management in response to peripheral hormonal signals. Its primary function is to maintain homeostasis at the molecular, cellular, and physiological levels through efficient utilization of energy obtained from feed intake (Bray, 2007). In instances of heightened energy intake, the brain modulates feeding behavior by either suppressing it or promoting the storage of excess energy as glycogen in the liver or triglycerides in adipose tissue (Huynh et al., 2016). An energy imbalance triggers the activation of the CNS to initiate the restoration of energy balance. In this context, the neuroendocrine function of AMPK acts as a regulator of metabolic processes orchestrated within the hypothalamus, influenced by changes in glucose, insulin, adiponectin, ghrelin, and leptin levels (Andersson et al., 2004; Minokoshi et al., 2004). In thermoneutral conditions, glucose and these hormones play a critical role in modulating feed intake. When overexpressed, adiponectin functions as a starvation signal. It regulates feeding behavior via the phosphorylation of AMPK, and it can lead to weight loss by increasing energy expenditure and reducing insulin resistance (Chu et al., 2006). Adiponectin triggers AMPK activation in the liver and muscle, leading to enhanced glucose utilization and fatty acid oxidation, while simultaneously inhibiting glucose production in the liver (Yamauchi et al., 2002). Contrarily, ghrelin stimulates hypothalamic AMPK phosphorylation, leading to an increase in food intake and a reduction in energy expenditure. Leptin, meanwhile, inhibits AMPK activity (Figure 6A1), resulting in diminished food intake and heightened energy expenditure (Minokoshi et al., 2002; Yildiz et al., 2004; Kahn et al., 2005). In skeletal muscle, leptin directly enhances AMPK activity, promoting fatty acid oxidation and glucose uptake in response to muscle contraction (Mu et al., 2001; Minokoshi et al., 2002), this is followed by the indirect activation of the hypothalamic-sympathetic nervous system (Friedman and Halaas, 1998; Minokoshi et al., 2002).
Under HS conditions and subsequent caloric restriction, AMPK and the protein silent information regulator T1 (SIRT1) respond to changes in nutrient availability caused by restriction or starvation (Chen et al., 2005; Chen et al., 2008), as well as alterations in energy expenditure (Cantó et al., 2009; Cantó et al., 2010). SIRT1 activates crucial mechanisms to uphold energy and metabolic homeostasis. AMPK and SIRT1 share similar functions in terms of cellular fuel metabolism, inflammation, and mitochondrial function (Ruderman et al., 2010).
The mitochondria, being the primary organelles responsible for cellular energy production, utilize nutrients, including AA, to maintain homeostasis based on cellular demands (Johnson et al., 2014). Supplementation with specific AA, such as Leu, has demonstrated a significant role in activating SIRT1 to prevent mitochondrial dysfunction (Li et al., 2012). Leu has shown beneficial effects on mitochondrial biogenesis and fatty acid oxidation in C2C12 myotubes through the activation of SIRT1 and subsequent activation of AMPK (Liang et al., 2014). Considering that the mitochondria is the primary source of ROS generation during HS or other disruptions in homeostasis, SIRT1 activation could promote mitochondrial biogenesis under conditions of energy deficiency (Tang, 2016). Survival mechanisms associated with the mitochondria, such as autophagy/mitophagy, have become increasingly relevant in economically significant animal species exposed to HS (Brownstein et al., 2017; Ganesan et al., 2017). Autophagy/mitophagy mechanisms serve as a means for cellular nutrient reabsorption by facilitating the recycling of proteins and organelles (Klionsky and Emr, 2000). These processes play a crucial role in promoting cell survival during periods of nutritional stress. In a broader context, the reduced nutrient consumption in response to HS is associated with an increase in the production of ROS, primarily within the mitochondria. This event triggers the activation of signaling pathways such as AMPK, which function to maintain cellular energy homeostasis.
mTOR activation by AA consumption and mitochondrial response
The investigation of the AMPK signaling pathway remains significant owing to its capacity to regulate energy metabolism and serve as a nutrient sensor, attributes that can be easily influenced by environmental conditions. Adequate protein synthesis and high energy demands are closely associated with feed intake. However, when feed consumption decreases, there is a rapid reduction in protein synthesis to conserve cellular energy. In animal production, HS suppresses body protein deposition and promotes increased accumulation of body fat (Ain Baziz et al., 1996). Chronic HS has the capability to suppress RNA transcription, protein synthesis, and muscle protein turnover (Jacob, 1995; Temim et al., 2000). However, in the context of lipid and carbohydrate metabolism, HS decreases fat oxidation in pigs, regardless of nutrient intake (Pearce et al., 2011).
mTOR is activated by various factors, including energy status, growth factors, mechanical stimuli, and notably, AA (Bond, 2016). mTOR primarily regulates protein synthesis (Duan et al., 2018b), and inhibits the induction of autophagy when there are sufficient nutrients available (Klionsky and Emr, 2000). The association between AMPK and mTOR arises from studies examining the phosphorylation and activity of S6 kinase (S6K) and eukaryotic initiation factor E4 binding protein 1 (4EBP1), which are targets of both mTOR and AMPK, demonstrating the convergence of these pathways (Xu et al., 2012). mTOR, belonging to the phosphatidylinositol 3-kinase (Pl3K)-related kinases (PlKK) family, is a highly conserved serine/threonine kinase (Xu et al., 2012). It functions in two complexes: mTOR complex 1 (mTORC1), which associates with the protein Raptor, and mTOR complex 2 (mTORC2), which associates with the protein Rictor (Kim et al., 2002). In the signaling pathway, mTORC1 phosphorylates S6K and 4EBP1, while mTORC2 phosphorylates the serine/threonine protein kinase AKT at Ser473, leading to AKT activation (Xu et al., 2012). mTORC1 plays a crucial role in regulating lipid, nucleotide, and protein synthesis, as well as autophagy (Figures 6B1, 2); on the other hand, mTORC2 is involved in promoting cell survival and proliferation (Saxton and Sabatini, 2017). When energy (in the form of ATP) is depleted due to nutrient deprivation, this triggers a signal from AMPK to the tuberous sclerosis complex (TSC2). This in turn inhibits the Rheb and mTOR signaling pathways, consequently leading to the suppression of protein synthesis (Inoki et al., 2003; Deldicque et al., 2005).
The protein kinase AKT not only serves as an upstream and positive regulator of mTOR, but also functions as a key modulator of energy metabolism through its inhibitory action on AMPK (Hahn-Windgassen et al., 2005). Activation of the AKT protein kinase leads to changes in ATP levels, resulting in a reduction of the AMP/ATP ratio and diminished activity of AMPK. AKT phosphorylates and inactivates TSC2, thereby facilitating the activation of mTOR (Potter et al., 2002). Overexpression of TSC2 results in a decrease in phosphorylation of S6K and 4EBP1 in response to nutrients or mitogens (Figure 6B), thereby implying that TSC2 serves as a negative regulator of mTOR signaling pathways (Tee et al., 2002). In this context, the AKT/mTOR/S6K pathway is recognized as a central regulator of protein synthesis and cellular growth (Xu et al., 2012). mTOR, a pleiotropic kinase, is also responsive to HS events (Jurivich et al., 1991) and its interaction with HSF1 can stimulate the expression of HSP (Chou et al., 2012). In the realm of animal production, HS has been demonstrated to exert various effects on the AMPK-mTOR signaling pathways. In pigs exposed to 30°C, for 21 days, a reduction in nutrient absorption due to changes in digestibility impacted AMPK-regulated intracellular energy metabolism, including glycolysis (as seen with alterations in hexokinase and pyruvate kinase activities), and resulted in changes in the enzymatic activities of pyruvate kinase and hexokinase in muscle tissue (Hao et al., 2014). However, for growing pigs subjected to chronic HS (33 ± 2°C), diets supplemented with hydroxy-4-methylselenobutanoic acid demonstrated a reduction in metabolic disorders within the liver (Yan et al., 2020). Investigations such as these, which examine the interplay between AMPK-mTOR, HSP, the antioxidant system, and the regulation of selenoproteins, remain relatively few. As such, these research domains represent promising targets for future studies.
Just as AA deprivation has been demonstrated to inhibit key signaling pathways (Hensen et al., 2012), the addition of certain AA can play a critical role in the activation of mTOR. Nutritional components, such as Leu and glucose positively affects the activation of mTOR (Reiling and Sabatini, 2006). Dietary components, such Leu and glucose, are known to favorably affect mTOR activation (Proud, 2002). Leu might interact with the mTOR pathway at or upstream of Ras homolog enriched in brain (Rheb), and reduced energy levels can trigger the activation of AMPK (Reiling and Sabatini, 2006). However, under thermoneutral conditions, the restriction of Lys intake via reduced food consumption, from piglets through to finishing pigs, impacted AA metabolism, gut microbiota, and inhibited AMPK signaling, though mTOR remained unaltered (Yin et al., 2018). In neonates, treatment with Leu was shown to stimulate muscle protein synthesis by augmenting mTORC1 activation (Suryawan et al., 2008). At the cellular level, supplementation with L-Glutamine (L-Gln) has been shown to stimulate enterocyte growth via mTOR, independently of the AMPK pathway (Yi et al., 2015). In this context, L-Gln demonstrated the ability to regulate genes associated with nutrient transport and energy metabolism. These findings underscore the significant role that dietary formulations play in muscle or organ protein synthesis and suggest that HS factors could modulate (either enhance or diminish) these effects. Numerous studies have proposed a potential interplay between mTOR and mitochondrial function, given the demonstrated involvement of mitochondria in the regulation of lipid metabolism in adipocytes (Ricoult and Manning, 2013; Bradley and Swann, 2019; Zhang et al., 2020). A diet low in protein but high in branched chain AA has been shown to enhance and regulate lipid metabolism in skeletal muscle, via the AMPK-mTOR pathway (Duan et al., 2018a). AA such as Leu are intimately linked to lipid metabolism, energy homeostasis, and the modulation of mitochondrial dysfunction. Leu has been demonstrated to phosphorylate 4EBP1 and activate the mTOR pathway, thereby accelerating fatty acid oxidation, promoting lipolysis, and increasing energy consumption in adipocytes (Fox et al., 1998; Yuan et al., 2015; Han et al., 2018). However, chronic supplementation of Leu at low doses has been observed to reduce the rate of fat production and overall body fat (Jiao et al., 2016). The energy derived from fatty acid oxidation in adipose tissue has been demonstrated to be primarily utilized for protein turnover in muscle tissue (Yan et al., 2017). As a result, Leu has the capacity to decrease fat deposition and reduce body weight. Consequently, Leu exhibits a regulatory impact on both protein synthesis and lipid deposition (Zhang et al., 2020).
The content and function of mitochondria are critical for maintaining robust muscle health, (Valero, 2014). Similarly, studies pointed out that when the metabolism of muscle mitochondria is impaired, particularly in relation to the oxidation of fatty acids, it could lead to an accumulation of fat in the muscles (Gumucio et al., 2019; Kim et al., 2019). Recent studies such as the one conducted by Li et al. (2018) propose that feeding pigs a low protein diet could enhance the quality of their meat. This enhancement is achieved by controlling the level of intramuscular fat, adjusting the composition of fatty acids, modulating the characteristics of muscle fibers, and altering the profile of free AA present in the muscle.
Concluding remarks
As global population grows exponentially, the demand for high-quality animal production also increases, and standards become more stringent. In economically significant sectors, it is crucial to ensure that environmental stressors, such as HS, do not significantly degrade the quality of animal production. A detailed understanding of the molecular, cellular, and physiological processes associated with nutritional regulation is vital. It’s important to understand how stress is generated, how energy is regulated, and how protein synthesis occurs in response to environmental and nutritional events, as these processes appear to play a crucial role in animal health and productivity. Appropriate dietary formulation with specific emphasis on AA supplementation could be key to effectively “turning on or off” crucial metabolic processes in animals during periods of HS. The generation of ROS seems to be effectively countered by energy-consuming antioxidant mechanisms, all while maintaining homeostasis. Certain molecules, such as chaperone proteins, play significant roles in these processes. However, the AMPK-mTOR signaling pathway has emerged as particularly important due to its role in energy balance and its activation through AA supplementation in diets. Despite these advances, research into these complex interplays is just beginning and could have significant implications for improving production performance in the future.
Author contributions
VM-C: Conceptualization, Data curation, Formal Analysis, Funding acquisition, Investigation, Methodology, Project administration, Software, Supervision, Validation, Visualization, Writing – original draft, Writing – review & editing. LPF: Conceptualization, Formal Analysis, Supervision, Writing – review & editing. AGC: Writing –review & editing, Funding acquisition.
Funding
The author(s) declare financial support was received for the research, authorship, and/or publication of this article. Funding: Convocatorias para el Desarrollo Académico 2023, de la Rectoría de Unidad Xochimilco Departamento de Producción Agrícola y Animal. División de CBS. Universidad Autónoma Metropolitana. Unidad Xochimilco. Ciudad de México, México.
Acknowledgments
We appreciate the constructive feedback from our colleagues and reviewers, which significantly improved the manuscript. Additionally, we express our gratitude to Convocatorias para el Desarrollo Académico 2023, de la Rectoría de Unidad Xochimilco for their funding.
Conflict of interest
The authors declare that the research was conducted in the absence of any commercial or financial relationships that could be construed as a potential conflict of interest.
Publisher’s note
All claims expressed in this article are solely those of the authors and do not necessarily represent those of their affiliated organizations, or those of the publisher, the editors and the reviewers. Any product that may be evaluated in this article, or claim that may be made by its manufacturer, is not guaranteed or endorsed by the publisher.
References
Ain Baziz H., Geraert P. A., Padilha J. C., Guillaumin S. (1996). Chronic heat exposure enhances fat deposition and modifies muscle and fat partition in broiler carcasses. Poult Sci. 75 (4), 505–513. doi: 10.3382/ps.0750505
Akbarian A., Michiels J., Degroote J., Majdeddin M., Golian A., De Smet S. (2016). Association between heat stress and oxidative stress in poultry; mitochondrial dysfunction and dietary interventions with phytochemicals. J. Anim. Sci. Biotechnol. 7, 37–37. doi: 10.1186/s40104-016-0097-5
Andersson U., Filipsson K., Abbott C. R., Woods A., Smith K., Bloom S. R., et al. (2004). AMP-activated protein kinase plays a role in the control of food intake. J. Biol. Chem. 279 (13), 12005–12008. doi: 10.1074/jbc.C300557200
Andretta I., Pomar C., Rivest J., Pomar J., Lovatto P. A., Radünz Neto J. (2014). The impact of feeding growing-finishing pigs with daily tailored diets using precision feeding techniques on animal performance, nutrient utilization, and body and carcass composition. J. Anim. Sci. 92 (9), 3925–3936. doi: 10.2527/jas.2014-7643
Asakura H., Kitahora T. (2018). “). Chapter 23 - antioxidants and polyphenols in inflammatory bowel disease: ulcerative colitis and crohn disease,” in Polyphenols: Prevention and Treatment of Human Disease (Second Edition). Eds. Watson R. R., Preedy V. R., Zibadi S., 279–292. doi: 10.1016/B978-0-12-813008-7.00023-0
Azad M. A., Kikusato M., Sudo S., Amo T., Toyomizu M. (2010). Time course of ROS production in skeletal muscle mitochondria from chronic heat-exposed broiler chicken. Comp. Biochem. Physiol. A Mol. Integr. Physiol. 157 (3), 266–271. doi: 10.1016/j.cbpa.2010.07.011
Barbe M., Tytell M., Gower D., Welch W. (1988). Hyperthermia protects against light damage in the rat retina. Science 241 (4874), 1817–1820. doi: 10.1126/science.3175623
Bartko P., Vrzgula L., Paulíková I., Reichel P. (1984). The effect of heat stress on acid-base homeostasis in pigs. Vet. Med. (Praha) 29 (6), 337–344.
Baumgard L. H., Rhoads R. P. Jr (2013). Effects of heat stress on postabsorptive metabolism and energetics. Annu. Rev. Anim. Biosci. 1 (1), 311–337. doi: 10.1146/annurev-animal-031412-103644
Birben E., Sahiner U. M., Sackesen C., Erzurum S., Kalayci O. (2012). Oxidative stress and antioxidant defense. World Allergy Organ. J. 5 (1), 9–19. doi: 10.1097/WOX.0b013e3182439613
Bond P. (2016). Regulation of mTORC1 by growth factors, energy status, amino acids and mechanical stimuli at a glance. J. Int. Soc. Sports Nutr. 13 (1), 8. doi: 10.1186/s12970-016-0118-y
Bradley J., Swann K. (2019). Mitochondria and lipid metabolism in mammalian oocytes and early embryos. Int. J. Dev. Biol. 63 (3-4-5), 93–103. doi: 10.1387/ijdb.180355ks
Bray G. A. (2007). Regulation of energy balanc: studies on gnetic, hypothalamic and dietary obesity. Proc. Nutr. Soc. 41 (2), 95–108. doi: 10.1079/PNS19820018
Brownstein A. J., Ganesan S., Summers C. M., Pearce S., Hale B. J., Ross J. W., et al. (2017). Heat stress causes dysfunctional autophagy in oxidative skeletal muscle. Physiol. Rep. 5 (12), e13317. doi: 10.14814/phy2.13317
Canada A. T., Calabrese E. J. (1989). Superoxide dismutase: Its role in xenobiotic detoxification. Pharmacol. Ther. 44 (2), 285–295. doi: 10.1016/0163-7258(89)90068-5
Cantó C., Gerhart-Hines Z., Feige J. N., Lagouge M., Noriega L., Milne J. C., et al. (2009). AMPK regulates energy expenditure by modulating NAD+ metabolism and SIRT1 activity. Nature 458 (7241), 1056–1060. doi: 10.1038/nature07813
Cantó C., Jiang L. Q., Deshmukh A. S., Mataki C., Coste A., Lagouge M., et al. (2010). Interdependence of AMPK and SIRT1 for metabolic adaptation to fasting and exercise in skeletal muscle. Cell Metab. 11 (3), 213–219. doi: 10.1016/j.cmet.2010.02.006
Carling D., Mayer F. V., Sanders M. J., Gamblin S. J. (2011). AMP-activated protein kinase: nature's energy sensor. Nat. Chem. Biol. 7 (8), 512–518. doi: 10.1038/nchembio.610
Cecarini V., Gee J., Fioretti E., Amici M., Angeletti M., Eleuteri A. M., et al. (2007). Protein oxidation and cellular homeostasis: Emphasis on metabolism. Biochim. Biophys. Acta (BBA) - Mol. Cell Res. 1773 (2), 93–104. doi: 10.1016/j.bbamcr.2006.08.039
Cervantes M., Antoine D., Valle J. A., Vásquez N., Camacho R. L., Bernal H., et al. (2018). Effect of feed intake level on the body temperature of pigs exposed to heat stress conditions. J. Thermal Biol. 76, 1–7. doi: 10.1016/j.jtherbio.2018.06.010
Cervantes M., Ibarra N., Vásquez N., Reyes F., Avelar E., Espinoza S., et al. (2017). Serum concentrations of free amino acids in growing pigs exposed to diurnal heat stress fluctuations. J. Therm Biol. 69, 69–75. doi: 10.1016/j.jtherbio.2017.06.008
Chen D., Bruno J., Easlon E., Lin S. J., Cheng H. L., Alt F. W., et al. (2008). Tissue-specific regulation of SIRT1 by calorie restriction. Genes Dev. 22 (13), 1753–1757. doi: 10.1101/gad.1650608
Chen D., Steele A. D., Lindquist S., Guarente L. (2005). Increase in activity during calorie restriction requires Sirt1. Science 310 (5754), 1641. doi: 10.1126/science.1118357
Chen J., Wang F., Zhou X., Cao Y., Li Y., Li C. (2017). Bama miniature pigs' liver possess great heat tolerance through upregulation of Nrf2-mediated antioxidative enzymes J Therm Biol 67, 15–21. doi: 10.1016/j.jtherbio.2017.04.012
Choct M. (2009). Managing gut health through nutrition. Br. Poult Sci. 50 (1), 9–15. doi: 10.1080/00071660802538632
Chou S.-D., Prince T., Gong J., Calderwood S. K. (2012). mTOR is essential for the proteotoxic stress response, HSF1 activation and heat shock protein synthesis. PloS One 7 (6), e39679–e39679. doi: 10.1371/journal.pone.0039679
Christon R. (1988). The effect of tropical ambient temperature on growth and metabolism in pigs. J. Anim. Sci. 66 (12), 3112–3123. doi: 10.2527/jas1988.66123112x
Chu M. C., Cosper P., Nakhuda G. S., Lobo R. A. (2006). A comparison of oral and transdermal short-term estrogen therapy in postmenopausal women with metabolic syndrome. Fertil Steril 86 (6), 1669–1675. doi: 10.1016/j.fertnstert.2006.04.043
Council N. R. (1981). Effect of environment on nutrient requirements of domestic animals. Natl. Academies Press. doi: 10.17226/4963
Cui Y., Gu X. (2015). Proteomic changes of the porcine small intestine in response to chronic heat stress. J. Mol. Endocrinol. 55 (3), 277. doi: 10.1530/jme-15-0161
Cui Y., Hao Y., Li J., Bao W., Li G., Gao Y., et al. (2016). Chronic heat stress induces immune response, oxidative stress response, and apoptosis of finishing pig liver: A proteomic approach. Int. J. Mol. Sci. 17 (5), 1–24. doi: 10.3390/ijms17050393
Cui Y., Wang C., Hao Y., Gu X., Wang H. (2019). Chronic heat stress induces acute phase responses and serum metabolome changes in finishing pigs. Anim. (Basel) 9 (7), 1–13. doi: 10.3390/ani9070395
D'Allaire S., Drolet R., Brodeur D. (1996). Sow mortality associated with high ambient temperatures. Can. veterinary J. = La Rev. veterinaire Can. 37 (4), 237–239.
Davies S. F., Iber C., Keene S. A., McArthur C. D., Path M. J. (1986). Effect of respiratory alkalosis during exercise on blood lactate. J. Appl. Physiol. (1985) 61 (3), 948–952. doi: 10.1152/jappl.1986.61.3.948
Deisseroth A., Dounce A. L. (1970). Catalase: Physical and chemical properties, mechanism of catalysis, and physiological role. Physiol. Rev. 50 (3), 319–375. doi: 10.1152/physrev.1970.50.3.319
Deldicque L., Theisen D., Francaux M. (2005). Regulation of mTOR by amino acids and resistance exercise in skeletal muscle. Eur. J. Appl. Physiol. 94 (1-2), 1–10. doi: 10.1007/s00421-004-1255-6
Duan Y., Li F., Guo Q., Wang W., Zhang L., Wen C., et al. (2018a). Branched-chain amino acid ratios modulate lipid metabolism in adipose tissues of growing pigs. J. Funct. Foods 40, 614–624. doi: 10.1016/j.jff.2017.12.004
Duan Y., Zhang L., Li F., Guo Q., Long C., Yin Y., et al. (2018b). β-Hydroxy-β-methylbutyrate modulates lipid metabolism in adipose tissues of growing pigs. Food Funct. 9 (9), 4836–4846. doi: 10.1039/c8fo00898a
Flanagan S. W., Moseley P. L., Buettner G. R. (1998). Increased flux of free radicals in cells subjected to hyperthermia: detection by electron paramagnetic resonance spin trapping. FEBS Lett. 431 (2), 285–286. doi: 10.1016/S0014-5793(98)00779-0
Fox H. L., Pham P. T., Kimball S. R., Jefferson L. S., Lynch C. J. (1998). Amino acid effects on translational repressor 4E-BP1 are mediated primarily by L-leucine in isolated adipocytes. Am. J. Physiol. 275 (5), C1232–C1238. doi: 10.1152/ajpcell.1998.275.5.C1232
Friedman J. M., Halaas J. L. (1998). Leptin and the regulation of body weight in mammals. Nature 395 (6704), 763–770. doi: 10.1038/27376
Fuquay J. W. (1981). Heat stress as it affects animal production. J. Anim. Sci. 52 (1), 164–174. doi: 10.2527/jas1981.521164x
Gabler N., Pearce S. (2015). The impact of heat stress on intestinal function and productivity in grow-finish pigs. Anim. Production Sci. 55, 1403–1410. doi: 10.1071/AN15280
Gabler N. K., Koltes D., Schaumberger S., Murugesan G. R., Reisinger N. (2018). Diurnal heat stress reduces pig intestinal integrity and increases endotoxin translocation. Trans. Anim. Sci. 2 (1), 1–10. doi: 10.1093/tas/txx003
Gad S. C. (2014). “Glutathione,” in Encyclopedia of Toxicology (Third Edition). Ed. Wexler P., 751. doi: 10.1016/B978-0-12-386454-3.00850-2
Gan F., Ren F., Chen X., Lv C., Pan C., Ye G., et al. (2013). Effects of Selenium-Enriched Probiotics on Heat Shock Protein mRNA Levels in Piglet under Heat Stress Conditions. J. Agric. Food Chem. 61 (10), 2385–2391. doi: 10.1021/jf300249j
Ganesan S., Summers C. M., Pearce S. C., Gabler N. K., Valentine R. J., Baumgard L. H., et al. (2017). Short-term heat stress causes altered intracellular signaling in oxidative skeletal muscle. J. Anim. Sci. 95 (6), 2438–2451. doi: 10.2527/jas.2016.1233
Ganesan S., Summers C. M., Pearce S. C., Gabler N. K., Valentine R. J., Baumgard L. H., et al. (2018). Short-term heat stress altered metabolism and insulin signaling in skeletal muscle. J. Anim. Sci. 96 (1), 154–167. doi: 10.1093/jas/skx083
García-Villalobos H., Morales-Trejo A., Araiza-Piña B. A., Htoo J. K., Cervantes-Ramírez M. (2012). Effects of dietary protein and amino acid levels on the expression of selected cationic amino acid transporters and serum amino acid concentration in growing pigs. Arch. Anim. Nutr. 66 (4), 257–270. doi: 10.1080/1745039x.2012.697351
Glory A., Averill-Bates D. A. (2016). The antioxidant transcription factor Nrf2 contributes to the protective effect of mild thermotolerance (40°C) against heat shock-induced apoptosis. Free Radic. Biol. Med. 99, 485–497. doi: 10.1016/j.freeradbiomed.2016.08.032
Gordon J. B., Rehorst-Paea L. A., Hoffman G. M., Nelin L. D. (1999). Pulmonary vascular responses during acute and sustained respiratory alkalosis or acidosis in intact newborn piglets. Pediatr. Res. 46 (6), 735–741. doi: 10.1203/00006450-199912000-00013
Gumucio J. P., Qasawa A. H., Ferrara P. J., Malik A. N., Funai K., McDonagh B., et al. (2019). Reduced mitochondrial lipid oxidation leads to fat accumulation in myosteatosis. FASEB J. 33 (7), 7863–7881. doi: 10.1096/fj.201802457RR
Hahn-Windgassen A., Nogueira V., Chen C. C., Skeen J. E., Sonenberg N., Hay N. (2005). Akt activates the mammalian target of rapamycin by regulating cellular ATP level and AMPK activity. J. Biol. Chem. 280 (37), 32081–32089. doi: 10.1074/jbc.M502876200
Hall D. M., Buettner G. R., Oberley L. W., Xu L., Matthes R. D., Gisolfi C. V. (2001). Mechanisms of circulatory and intestinal barrier dysfunction during whole body hyperthermia. Am. J. Physiology-Heart Circulatory Physiol. 280 (2), H509–H521. doi: 10.1152/ajpheart.2001.280.2.H509
Halliwell B., Whiteman M. (2004). Measuring reactive species and oxidative damage in vivo and in cell culture: how should you do it and what do the results mean? Br. J. Pharmacol. 142 (2), 231–255. doi: 10.1038/sj.bjp.0705776
Han G., Yang H., Bungo T., Ikeda H., Wang Y., Nguyen L. T. N., et al. (2018). In ovoL-leucine administration stimulates lipid metabolisms in heat-exposed male, but not female, chicks to afford thermotolerance. J. Therm Biol. 71, 74–82. doi: 10.1016/j.jtherbio.2017.10.020
Hao Y., Feng Y., Yang P., Feng J., Lin H., Gu X. (2014). Nutritional and physiological responses of finishing pigs exposed to a permanent heat exposure during three weeks. Arch. Anim. Nutr. 68 (4), 296–308. doi: 10.1080/1745039x.2014.931522
Hao Y., Xing M., Gu X. (2021). Research progress on oxidative stress and its nutritional regulation strategies in pigs. Anim. (Basel) 11 (5), 1–21. doi: 10.3390/ani11051384
Hardie D. G. (2004). The AMP-activated protein kinase pathway – new players upstream and downstream. J. Cell Sci. 117 (23), 5479. doi: 10.1242/jcs.01540
Hardie D. G. (2007). AMP-activated/SNF1 protein kinases: conserved guardians of cellular energy. Nat. Rev. Mol. Cell Biol. 8 (10), 774–785. doi: 10.1038/nrm2249
Hassan F.-u., Nawaz A., Rehman M. S., Ali M. A., Dilshad S. M. R., Yang C. (2019). Prospects of HSP70 as a genetic marker for thermo-tolerance and immuno-modulation in animals under climate change scenario. Anim. Nutr. 5 (4), 340–350. doi: 10.1016/j.aninu.2019.06.005
He J., Guo H., Zheng W., Xue Y., Zhao R., Yao W. (2019). Heat stress affects fecal microbial and metabolic alterations of primiparous sows during late gestation. J. Anim. Sci. Biotechnol. 10, 84. doi: 10.1186/s40104-019-0391-0
He L., Wu L., Xu Z., Li T., Yao K., Cui Z., et al. (2016). Low-protein diets affect ileal amino acid digestibility and gene expression of digestive enzymes in growing and finishing pigs. Amino Acids 48 (1), 21–30. doi: 10.1007/s00726-015-2059-1
Hensen S. M., Heldens L., van Enckevort C. M., van Genesen S. T., Pruijn G. J., Lubsen N. H. (2012). Heat shock factor 1 is inactivated by amino acid deprivation. Cell Stress chaperones 17 (6), 743–755. doi: 10.1007/s12192-012-0347-1
Hu C., Patil Y., Gong D., Yu T., Li J., Wu L., et al. (2022). Heat stress-induced dysbiosis of porcine colon microbiota plays a role in intestinal damage: A fecal microbiota profile. Front. veterinary Sci. 9. doi: 10.3389/fvets.2022.686902
Huynh T. T., Aarnink A. J., Verstegen M. W., Gerrits W. J., Heetkamp M. J., Kemp B., et al. (2005). Effects of increasing temperatures on physiological changes in pigs at different relative humidities. J. Anim. Sci. 83 (6), 1385–1396. doi: 10.2527/2005.8361385x
Huynh M. K. Q., Kinyua A. W., Yang D. J., Kim K. W. (2016). Hypothalamic AMPK as a regulator of energy homeostasis. Neural plasticity 2016, 2754078–2754078. doi: 10.1155/2016/2754078
Inoki K., Zhu T., Guan K. L. (2003). TSC2 mediates cellular energy response to control cell growth and survival. Cell 115 (5), 577–590. doi: 10.1016/s0092-8674(03)00929-2
Jacob S. T. (1995). Regulation of ribosomal gene transcription. Biochem. J. 306, 617–626. doi: 10.1042/bj3060617
Jiao J., Han S. F., Zhang W., Xu J. Y., Tong X., Yin X. B., et al. (2016). Chronic leucine supplementation improves lipid metabolism in C57BL/6J mice fed with a high-fat/cholesterol diet. Food Nutr. Res. 60, 31304. doi: 10.3402/fnr.v60.31304
Johnson M. A., Vidoni S., Durigon R., Pearce S. F., Rorbach J., He J., et al. (2014). Amino acid starvation has opposite effects on mitochondrial and cytosolic protein synthesis. PloS One 9 (4), e93597. doi: 10.1371/journal.pone.0093597
Jurivich D. A., Chung J., Blenis J. (1991). Heat shock induces two distinct S6 protein kinase activities in quiescent mammalian fibroblasts. J. Cell Physiol. 148 (2), 252–259. doi: 10.1002/jcp.1041480210
Kahn B. B., Alquier T., Carling D., Hardie D. G. (2005). AMP-activated protein kinase: ancient energy gauge provides clues to modern understanding of metabolism. Cell Metab. 1 (1), 15–25. doi: 10.1016/j.cmet.2004.12.003
Kamanga-Sollo E., Pampusch M. S., White M. E., Hathaway M. R., Dayton W. R. (2011). Effects of heat stress on proliferation, protein turnover, and abundance of heat shock protein messenger ribonucleic acid in cultured porcine muscle satellite cells. J. Anim. Sci. 89 (11), 3473–3480. doi: 10.2527/jas.2011-4123
Kehrer J. P., Robertson J. D., Smith C. V. (2010). “1.14 - free radicals and reactive oxygen species,” in Comprehensive Toxicology (Second Edition). Ed. McQueen C. A., 277–307. doi: 10.1016/B978-0-08-046884-6.00114-7
Kerr B. J., Yen J. T., Nienaber J. A., Easter R. A. (2003). Influences of dietary protein level, amino acid supplementation and environmental temperature on performance, body composition, organ weights and total heat production of growing pigs. J. Anim. Sci. 81 (8), 1998–2007. doi: 10.2527/2003.8181998x
Kiela P. R., Ghishan F. K. (2016). Physiology of intestinal absorption and secretion. Best Pract. Res. Clin. Gastroenterol. 30 (2), 145–159. doi: 10.1016/j.bpg.2016.02.007
Kim J., Keum Y.-S. (2016). NRF2, a key regulator of antioxidants with two faces towards cancer. Oxid. Med. Cell. Longevity 2016, 2746457–2746457. doi: 10.1155/2016/2746457
Kim E. J., Lee M., Kim D. Y., Kim K. I., Yi J. Y. (2019). Mechanisms of energy metabolism in skeletal muscle mitochondria following radiation exposure. Cells 8 (9), 1–18. doi: 10.3390/cells8090950
Kim D. H., Sarbassov D. D., Ali S. M., King J. E., Latek R. R., Erdjument-Bromage H., et al. (2002). mTOR interacts with raptor to form a nutrient-sensitive complex that signals to the cell growth machinery. Cell 110 (2), 163–175. doi: 10.1016/s0092-8674(02)00808-5
Klionsky D. J., Emr S. D. (2000). Autophagy as a regulated pathway of cellular degradation. Science 290 (5497), 1717–1721. doi: 10.1126/science.290.5497.1717
Kregel K. C. (2002). Invited Review: Heat shock proteins: modifying factors in physiological stress responses and acquired thermotolerance. J. Appl. Physiol. 92 (5), 2177–2186. doi: 10.1152/japplphysiol.01267.2001
Lauridsen C. (2019). From oxidative stress to inflammation: redox balance and immune system. Poult Sci. 98 (10), 4240–4246. doi: 10.3382/ps/pey407
Le Bellego L., van Milgen J., Noblet J. (2002). Effect of high temperature and low-protein diets on the performance of growing-finishing pigs. J. Anim. Sci. 80 (3), 691–701. doi: 10.2527/2002.803691x
Lee M. T., Lin W. C., Yu B., Lee T. T. (2017). Antioxidant capacity of phytochemicals and their potential effects on oxidative status in animals — A review. Asian-Australas J. Anim. Sci. 30 (3), 299–308. doi: 10.5713/ajas.16.0438
Le Sciellour M., Zemb O., Hochu I., Riquet J., Gilbert H., Giorgi M., et al. (2019). Effect of chronic and acute heat challenges on fecal microbiota composition, production, and thermoregulation traits in growing pigs1,2. J. Anim. Sci. 97 (9), 3845–3858. doi: 10.1093/jas/skz222
Li Y. H., Li F. N., Duan Y. H., Guo Q. P., Wen C. Y., Wang W. L., et al. (2018). Low-protein diet improves meat quality of growing and finishing pigs through changing lipid metabolism, fiber characteristics, and free amino acid profile of the muscle. J. Anim. Sci. 96 (8), 3221–3232. doi: 10.1093/jas/sky116
Li L., Tan H., Yang H., Li F., He X., Gu Z., et al. (2017). Reactive oxygen species mediate heat stress-induced apoptosis via ERK dephosphorylation and Bcl-2 ubiquitination in human umbilical vein endothelial cells. Oncotarget 8 (8), 12902–12916. doi: 10.18632/oncotarget.14186
Li H., Xu M., Lee J., He C., Xie Z. (2012). Leucine supplementation increases SIRT1 expression and prevents mitochondrial dysfunction and metabolic disorders in high-fat diet-induced obese mice. Am. J. Physiol. Endocrinol. Metab. 303 (10), E1234–E1244. doi: 10.1152/ajpendo.00198.2012
Liang C., Curry B. J., Brown P. L., Zemel M. B. (2014). Leucine modulates mitochondrial biogenesis and SIRT1-AMPK signaling in C2C12 myotubes. J. Nutr. Metab. 2014, 1–11. doi: 10.1155/2014/239750
Lindinger M. I., Heigenhauser G. J., Spriet L. L. (1990). Effects of alkalosis on muscle ions at rest and with intense exercise. Can. J. Physiol. Pharmacol. 68 (7), 820–829. doi: 10.1139/y90-125
Liu F., Celi P., Chauhan S. S., Cottrell J. J., Leury B. J., Dunshea F. R. (2018). A short-term supranutritional vitamin E supplementation alleviated respiratory alkalosis but did not reduce oxidative stress in heat stressed pigs. Asian-Australas J. Anim. Sci. 31 (2), 263–269. doi: 10.5713/ajas.17.0256
Liu F., Cottrell J. J., Furness J. B., Rivera L. R., Kelly F. W., Wijesiriwardana U., et al. (2016). Selenium and vitamin E together improve intestinal epithelial barrier function and alleviate oxidative stress in heat-stressed pigs. Exp. Physiol. 101 (7), 801–810. doi: 10.1113/ep085746
Liu F., Yin J., Du M., Yan P., Xu J., Zhu X., et al. (2009). Heat-stress-induced damage to porcine small intestinal epithelium associated with downregulation of epithelial growth factor signaling. J. Anim. Sci. 87 (6), 1941–1949. doi: 10.2527/jas.2008-1624
Loschen G., Azzi A. (1975). On the formation of hydrogen peroxide and oxygen radicals in heart mitochondria. Recent Adv. Stud. Cardiac Struct. Metab. 7, 3–12.
Lu S. C. (2013). Glutathione synthesis. Biochim. Biophys. Acta 1830 (5), 3143–3153. doi: 10.1016/j.bbagen.2012.09.008
Lucy M. C., Safranski T. J. (2017). Heat stress in pregnant sows: Thermal responses and subsequent performance of sows and their offspring. Mol. Reprod. Dev. 84 (9), 946–956. doi: 10.1002/mrd.22844
Lushchak V. (2015). Free radicals, reactive oxygen species, oxidative stresses and their classifications. Ukrainian Biochem. J. 87, 11–18. doi: 10.15407/ubj87.06.011
Ma X., Jiang Z., Zheng C., hu Y., Wang L. (2015). Nutritional regulation for meat quality and nutrient metabolism of pigs exposed to high temperature environment. J. Nutr. Food Sci. 05. doi: 10.4172/2155-9600.1000420
Ma X., Wang L., Shi Z., Chen W., Yang X., Hu Y., et al. (2019). Mechanism of continuous high temperature affecting growth performance, meat quality, and muscle biochemical properties of finishing pigs. Genes Nutr. 14 (1), 23. doi: 10.1186/s12263-019-0643-9
Mayorga E. J., Renaudeau D., Ramirez B. C., Ross J. W., Baumgard L. H. (2019). Heat stress adaptations in pigs. Anim. Front. 9 (1), 54–61. doi: 10.1093/af/vfy035
Meng Q., Guo T., Li G., Sun S., He S., Cheng B., et al. (2018). Dietary resveratrol improves antioxidant status of sows and piglets and regulates antioxidant gene expression in placenta by Keap1-Nrf2 pathway and Sirt1. Journal of animal science and biotechnology 9, 34–34. doi: 10.1186/s40104-018-0248-y
Mihaylova M. M., Shaw R. J. (2011). The AMPK signalling pathway coordinates cell growth, autophagy and metabolism. Nat. Cell Biol. 13 (9), 1016–1023. doi: 10.1038/ncb2329
Minokoshi Y., Alquier T., Furukawa N., Kim Y. B., Lee A., Xue B., et al. (2004). AMP-kinase regulates food intake by responding to hormonal and nutrient signals in the hypothalamus. Nature 428 (6982), 569–574. doi: 10.1038/nature02440
Minokoshi Y., Kim Y.-B., Peroni O. D., Fryer L. G. D., Müller C., Carling D., et al. (2002). Leptin stimulates fatty-acid oxidation by activating AMP-activated protein kinase. Nature 415 (6869), 339–343. doi: 10.1038/415339a
Mirzad A. N., Tada T., Ano H., Kobayashi I., Yamauchi T., Katamoto H. (2018). Seasonal changes in serum oxidative stress biomarkers in dairy and beef cows in a daytime grazing system. J. Vet. Med. Sci. 80 (1), 20–27. doi: 10.1292/jvms.17-0321
Montesinos-Cruz V., Cota M., Buenabad L., Cervantes M., Morales A. (2019). Effect of heat stress on the expression of HSP70, UCP3 and CYP450 genes in liver; longissimus dorsi and semitendinosus muscle of growing pigs. Am. J. Anim. Veterinary Sci. 14 (4), 221–230. doi: 10.3844/ajavsp.2019.221.230
Montilla S. I., Johnson T. P., Pearce S. C., Gardan-Salmon D., Gabler N. K., Ross J. W., et al. (2014). Heat stress causes oxidative stress but not inflammatory signaling in porcine skeletal muscle. Temperature (Austin) 1 (1), 42–50. doi: 10.4161/temp.28844
Morales A., Chávez M., Vásquez N., Camacho L., Avelar E., Arce N., et al. (2019). Extra dietary protein-bound or free amino acids differently affect the serum concentrations of free amino acids in heat-stressed pigs. J. Anim. Sci. 97 (4), 1734–1744. doi: 10.1093/jas/skz037
Morales A., Chávez M., Vásquez N., Htoo J. K., Buenabad L., Espinoza S., et al. (2018). Increased dietary protein or free amino acids supply for heat stress pigs: effect on performance and carcass traits. J. Anim. Sci. 96 (4), 1419–1429. doi: 10.1093/jas/sky044
Morales A., Gómez T., Villalobos Y. D., Bernal H., Htoo J. K., González-Vega J. C., et al. (2020). Dietary protein-bound or free amino acids differently affect intestinal morphology, gene expression of amino acid transporters, and serum amino acids of pigs exposed to heat stress. J. Anim. Sci. 98 (3), 1–9. doi: 10.1093/jas/skaa056
Morales A., Grageola F., García H., Araiza A., Zijlstra R. T., Cervantes M. (2013). Expression of cationic amino acid transporters, carcass traits, and performance of growing pigs fed low-protein amino acid-supplemented versus high protein diets. Genet. Mol. Res. 12 (4), 4712–4722. doi: 10.4238/2013.October.18.9
Morales A., Grageola F., García H., Arce N., Araiza B., Yáñez J., et al. (2014). Performance, serum amino acid concentrations and expression of selected genes in pair-fed growing pigs exposed to high ambient temperatures. J. Anim. Physiol. Anim. Nutr. 98 (5), 928–935. doi: 10.1111/jpn.12161
Morales A., Hernández L., Buenabad L., Avelar E., Bernal H., Baumgard L. H., et al. (2016a). Effect of heat stress on the endogenous intestinal loss of amino acids in growing pigs. J. Anim. Sci. 94 (1), 165–172. doi: 10.2527/jas.2015-9393
Morales A., Pérez M., Castro P., Ibarra N., Bernal H., Baumgard L. H., et al. (2016b). Heat stress affects the apparent and standardized ileal digestibilities of amino acids in growing pigs. J. Anim. Sci. 94 (8), 3362–3369. doi: 10.2527/jas.2016-0571
Morrow-Tesch J. L., McGlone J. J., Salak-Johnson J. L. (1994). Heat and social stress effects on pig immune measures. J. Anim. Sci. 72 (10), 2599–2609. doi: 10.2527/1994.72102599x
Mu J., Brozinick J. T. Jr., Valladares O., Bucan M., Birnbaum M. J. (2001). A role for AMP-activated protein kinase in contraction- and hypoxia-regulated glucose transport in skeletal muscle. Mol. Cell 7 (5), 1085–1094. doi: 10.1016/s1097-2765(01)00251-9
Mujahid A., Akiba Y., Warden C. H., Toyomizu M. (2007). Sequential changes in superoxide production, anion carriers and substrate oxidation in skeletal muscle mitochondria of heat-stressed chickens. FEBS Lett. 581 (18), 3461–3467. doi: 10.1016/j.febslet.2007.06.051
Mujahid A., Yoshiki Y., Akiba Y., Toyomizu M. (2005). Superoxide radical production in chicken skeletal muscle induced by acute heat stress. Poult Sci. 84 (2), 307–314. doi: 10.1093/ps/84.2.307
Murphy M. P. (2009). How mitochondria produce reactive oxygen species. Biochem. J. 417 (1), 1–13. doi: 10.1042/BJ20081386
Murray K. F., Messner D. J., Kowdley K. V. (2006). “CHAPTER 58 - mechanisms of hepatocyte detoxification,” in Physiology of the Gastrointestinal Tract (Fourth Edition). Ed. Johnson L. R., 1483–1504. doi: 10.1016/B978-012088394-3/50061-1
Nandi A., Yan L.-J., Jana C. K., Das N. (2019). Role of catalase in oxidative stress- and age-associated degenerative diseases. Oxid. Med. Cell. Longevity 2019, 9613090–9613090. doi: 10.1155/2019/9613090
Nguyen T., Nioi P., Pickett C. B. (2009). The Nrf2-antioxidant response element signaling pathway and its activation by oxidative stress. J. Biol. Chem. 284 (20), 13291–13295. doi: 10.1074/jbc.R900010200
Page T. J., Sikder D., Yang L., Pluta L., Wolfinger R. D., Kodadek T., et al. (2006). Genome-wide analysis of human HSF1 signaling reveals a transcriptional program linked to cellular adaptation and survival. Mol. Biosyst. 2 (12), 627–639. doi: 10.1039/b606129j
Patra A. K., Kar I. (2021). Heat stress on microbiota composition, barrier integrity, and nutrient transport in gut, production performance, and its amelioration in farm animals. J. Anim. Sci. Technol. 63 (2), 211–247. doi: 10.5187/jast.2021.e48
Patriarca E. J., Maresca B. (1990). Acquired thermotolerance following heat shock protein synthesis prevents impairment of mitochondrial ATPase activity at elevated temperatures in Saccharomyces cerevisiae. Exp. Cell Res. 190 (1), 57–64. doi: 10.1016/0014-4827(90)90143-x
Paulusma C. C., Lamers W. H., Broer S., van de Graaf S. F. J. (2022). Amino acid metabolism, transport and signalling in the liver revisited. Biochem. Pharmacol. 201, 115074. doi: 10.1016/j.bcp.2022.115074
Pearce S. C., Gabler N. K., Ross J. W., Escobar J., Patience J. F., Rhoads R. P., et al. (2013). The effects of heat stress and plane of nutrition on metabolism in growing pigs. J. Anim. Sci. 91 (5), 2108–2118. doi: 10.2527/jas.2012-5738
Pearce S. C., Lonergan S. M., Huff-Lonergan E., Baumgard L. H., Gabler N. K. (2015). Acute heat stress and reduced nutrient intake alter intestinal proteomic profile and gene expression in pigs. PloS One 10 (11), e0143099. doi: 10.1371/journal.pone.0143099
Pearce S., Mani V., Boddicker R., Johnson J., Weber T., Ross J., et al. (2012). Heat stress reduces barrier function and alters intestinal metabolism in growing pigs. J. Anim. Sci. 90, 257–259. doi: 10.2527/jas52339
Pearce S. C., Sanz-Fernandez M. V., Hollis J. H., Baumgard L. H., Gabler N. K. (2014). Short-term exposure to heat stress attenuates appetite and intestinal integrity in growing pigs. J. Anim. Sci. 92 (12), 5444–5454. doi: 10.2527/jas.2014-8407
Pearce S. C., Upah N. C., Harris A., Gabler N. K., Ross J. W., Rhoads R. P., et al. (2011). Effects of heat stress on energetic metabolism in growing pigs. FASEB J. 25 (S1), 1052.1055–1052.1055. doi: 10.1096/fasebj.25.1_supplement.1052.5
Pelham H. (1985). Activation of heat-shock genes in eukaryotes. Trends Genet. 1, 31–35. doi: 10.1016/0168-9525(85)90012-5
Pluske J. R., Turpin D. L., Kim J. C. (2018). Gastrointestinal tract (gut) health in the young pig. Anim. Nutr. 4 (2), 187–196. doi: 10.1016/j.aninu.2017.12.004
Pollman D. (2010). Seasonal effects on sow herds: industry experience and management strategies. J. Anim. Sci. 88, 9.
Potter C. J., Pedraza L. G., Xu T. (2002). Akt regulates growth by directly phosphorylating Tsc2. Nat. Cell Biol. 4 (9), 658–665. doi: 10.1038/ncb840
Proud C. G. (2002). Regulation of mammalian translation factors by nutrients. Eur. J. Biochem. 269 (22), 5338–5349. doi: 10.1046/j.1432-1033.2002.03292.x
Quiniou N., Dubois S., Noblet J. (2000). Voluntary feed intake and feeding behavior of grouphoused growing pigs are affected by ambient temperature and body weight. Livestock Production Sci. 63, 245–253. doi: 10.1016/S0301-6226(99)00135-9
Quiniou N., Noblet J., van Milgen J., Dubois S. (2001). Modelling heat production and energy balance in group-housed growing pigs exposed to low or high ambient temperatures. Br. J. Nutr. 85 (1), 97–106. doi: 10.1079/bjn2000217
Ramirez B. C., Hoff S. J., Hayes M. D., Brown-Brandl T., Harmon J. D., Rohrer G. A. (2022). A review of swine heat production: 2003 to 2020 [Systematic Review]. Front. Anim. Sci. 3. doi: 10.3389/fanim.2022.908434
Ratriyanto A., Mosenthin R. (2018). Osmoregulatory function of betaine in alleviating heat stress in poultry. J. Anim. Physiol. Anim. Nutr. 102 (6), 1634–1650. doi: 10.1111/jpn.12990
Reiling J. H., Sabatini D. M. (2006). Stress and mTORture signaling. Oncogene 25 (48), 6373–6383. doi: 10.1038/sj.onc.1209889
Ren M., Liu C., Zeng X., Yue L., Mao X., Qiao S., et al. (2014). Amino acids modulates the intestinal proteome associated with immune and stress response in weaning pig. Mol. Biol. Rep. 41 (6), 3611–3620. doi: 10.1007/s11033-014-3225-3
Renaudeau D., Anais C., Tel L., Gourdine J. L. (2010). Effect of temperature on thermal acclimation in growing pigs estimated using a nonlinear function. J. Anim. Sci. 88 (11), 3715–3724. doi: 10.2527/jas.2009-2169
Renaudeau D., Collin A., Yahav S., de Basilio V., Gourdine J. L., Collier R. J. (2011). Adaptation to hot climate and strategies to alleviate heat stress in livestock production. Animal 6 (5), 707–728. doi: 10.1017/S1751731111002448
Richter K., Haslbeck M., Buchner J. (2010). The heat shock response: life on the verge of death. Mol. Cell 40 (2), 253–266. doi: 10.1016/j.molcel.2010.10.006
Ricoult S. J., Manning B. D. (2013). The multifaceted role of mTORC1 in the control of lipid metabolism. EMBO Rep. 14 (3), 242–251. doi: 10.1038/embor.2013.5
Ritossa F. (1996). Discovery of the heat shock response. Cell Stress chaperones 1 (2), 97–98. doi: 10.1379/1466-1268(1996)001<0097:dothsr>2.3.co;2
Ross J. W., Hale B. J., Gabler N. K., Rhoads R. P., Keating A. F., Baumgard L. H. (2015). Physiological consequences of heat stress in pigs. Anim. Production Sci. 55 (12), 1381–1390. doi: 10.1071/AN15267
Ruderman N. B., Xu X. J., Nelson L., Cacicedo J. M., Saha A. K., Lan F., et al. (2010). AMPK and SIRT1: a long-standing partnership? Am. J. Physiology-Endocrinology Metab. 298 (4), E751–E760. doi: 10.1152/ajpendo.00745.2009
Sanz Fernandez V. M., Johnson J. S., Abuajamieh M., Stoakes S. K., Seibert J. T., Cox L., et al. (2015). Effects of heat stress on carbohydrate and lipid metabolism in growing pigs. Physiol. Rep. 3 (2), e12315. doi: 10.14814/phy2.12315
Saxton R. A., Sabatini D. M. (2017). mTOR signaling in growth, metabolism, and disease. Cell 168 (6), 960–976. doi: 10.1016/j.cell.2017.02.004
Sigdel A., Abdollahi-Arpanahi R., Aguilar I., Peñagaricano F. (2019). Whole genome mapping reveals novel genes and pathways involved in milk production under heat stress in US Holstein cows. Front Genet. 10 928, 1–10. doi: 10.3389/fgene.2019.00928
Sommer F., Bäckhed F. (2013). The gut microbiota–masters of host development and physiology. Nat. Rev. Microbiol. 11 (4), 227–238. doi: 10.1038/nrmicro2974
St-Pierre N., Cobanov B., Schnitkey G. (2003). Economic losses from heat stress by US livestock industries. J. Dairy Sci. - J. DAIRY Sci. 86, 52–77. doi: 10.3168/jds.S0022-0302(03)74040-5
Surai P. F., Kochish I. I., Fisinin V. I., Kidd M. T. (2019). Antioxidant defence systems and oxidative stress in poultry biology: an update. Antioxidants (Basel Switzerland) 8 (7), 235. doi: 10.3390/antiox8070235
Suryawan A., Jeyapalan A. S., Orellana R. A., Wilson F. A., Nguyen H. V., Davis T. A. (2008). Leucine stimulates protein synthesis in skeletal muscle of neonatal pigs by enhancing mTORC1 activation. Am. J. Physiology-Endocrinology Metab. 295 (4), E868–E875. doi: 10.1152/ajpendo.90314.2008
Tang B. L. (2016). Sirt1 and the mitochondria. Mol. Cells 39 (2), 87–95. doi: 10.14348/molcells.2016.2318
Tee A. R., Fingar D. C., Manning B. D., Kwiatkowski D. J., Cantley L. C., Blenis J. (2002). Tuberous sclerosis complex-1 and -2 gene products function together to inhibit mammalian target of rapamycin (mTOR)-mediated downstream signaling. Proc. Natl. Acad. Sci. U.S.A. 99 (21), 13571–13576. doi: 10.1073/pnas.202476899
Temim S., Chagneau A. M., Peresson R., Tesseraud S. (2000). Chronic heat exposure alters protein turnover of three different skeletal muscles in finishing broiler chickens fed 20 or 25% protein diets. J. Nutr. 130 (4), 813–819. doi: 10.1093/jn/130.4.813
Vacca R. A., de Pinto M. C., Valenti D., Passarella S., Marra E., De Gara L. (2004). Production of reactive oxygen species, alteration of cytosolic ascorbate peroxidase, and impairment of mitochondrial metabolism are early events in heat shock-induced programmed cell death in tobacco Bright-Yellow 2 cells. Plant Physiol. 134 (3), 1100–1112. doi: 10.1104/pp.103.035956
Valero T. (2014). Mitochondrial biogenesis: pharmacological approaches. Curr. Pharm. Des. 20 (35), 5507–5509. doi: 10.2174/138161282035140911142118
Valko M., Leibfritz D., Moncol J., Cronin M. T., Mazur M., Telser J. (2007). Free radicals and antioxidants in normal physiological functions and human disease. Int. J. Biochem. Cell Biol. 39 (1), 44–84. doi: 10.1016/j.biocel.2006.07.001
Velayudhan D. E., Kim I. H., Nyachoti C. M. (2015). Characterization of dietary energy in Swine feed and feed ingredients: a review of recent research results. Asian-Australas J. Anim. Sci. 28 (1), 1–13. doi: 10.5713/ajas.14.0001R
Vilas Boas Ribeiro B. P., Lanferdini E., Palencia J. Y. P., Lemes M. A. G., Teixeira de Abreu M. L., de Souza Cantarelli V., et al. (2018). Heat negatively affects lactating swine: A meta-analysis. J. Therm Biol. 74, 325–330. doi: 10.1016/j.jtherbio.2018.04.015
Volodina O., Ganesan S., Pearce S. C., Gabler N. K., Baumgard L. H., Rhoads R. P., et al. (2017). Short-term heat stress alters redox balance in porcine skeletal muscle. Physiol. Rep. 5 (8), e13267. doi: 10.14814/phy2.13267
Walter S., Buchner J. (2002). Molecular chaperones–cellular machines for protein folding. Angew Chem. Int. Ed Engl. 41 (7), 1098–1113. doi: 10.1002/1521-3773(20020402)41:7<1098::aid-anie1098>3.0.co;2-9
Wang S., Song P., Zou M. H. (2012). AMP-activated protein kinase, stress responses and cardiovascular diseases. Clin. Sci. (Lond) 122 (12), 555–573. doi: 10.1042/cs20110625
Weller M. M. D. C. A., Alebrante L., Campos P. H. R. F., Saraiva A., Silva B. A. N., Donzele J. L., et al. (2013). Effect of heat stress and feeding phosphorus levels on pig electron transport chain gene expression. Animal 7 (12), 1985–1993. doi: 10.1017/S1751731113001535
Wu G. (2009). Amino acids: metabolism, functions, and nutrition. Amino Acids 37 (1), 1–17. doi: 10.1007/s00726-009-0269-0
Wu X., Xie C., Yin Y., Li F., Li T., Huang R., et al. (2013). Effect of L-arginine on HSP70 expression in liver in weanling piglets. BMC Veterinary Res. 9 (1), 63. doi: 10.1186/1746-6148-9-63
Xiong Y., Cao S., Xiao H., Wu Q., Yi H., Jiang Z., et al. (2022). Alterations in intestinal microbiota composition coincide with impaired intestinal morphology and dysfunctional ileal immune response in growing-finishing pigs under constant chronic heat stress. J. Anim. Sci. Biotechnol. 13 (1), 1. doi: 10.1186/s40104-021-00651-6
Xiong Y., Yi H., Wu Q., Jiang Z., Wang L. (2020). Effects of acute heat stress on intestinal microbiota in grow-finishing pigs, and associations with feed intake and serum profile. J. Appl. Microbiol. 128 (3), 840–852. doi: 10.1111/jam.14504
Xu J., Ji J., Yan X. H. (2012). Cross-talk between AMPK and mTOR in regulating energy balance. Crit. Rev. Food Sci. Nutr. 52 (5), 373–381. doi: 10.1080/10408398.2010.500245
Yamauchi T., Kamon J., Minokoshi Y., Ito Y., Waki H., Uchida S., et al. (2002). Adiponectin stimulates glucose utilization and fatty-acid oxidation by activating AMP-activated protein kinase. Nat. Med. 8 (11), 1288–1295. doi: 10.1038/nm788
Yan L., Jiayong T., Ying H., Gang J., Guangmang L., Gang T., et al. (2020). Selenogenome and AMPK signal insight into the protective effect of dietary Se on chronic heat-stress induced hepatic metabolic disorder in growing pigs. J. Anim. Sci. Biotechnol. doi: 10.21203/rs.3.rs-130240/v1
Yan G., Li X., Peng Y., Long B., Fan Q., Wang Z., et al. (2017). The fatty acid β-oxidation pathway is activated by leucine deprivation in hepG2 cells: A comparative proteomics study. Sci. Rep. 7 (1), 1914–1914. doi: 10.1038/s41598-017-02131-2
Yang Z., Liao S. F. (2019). Physiological effects of dietary amino acids on gut health and functions of swine. Front. veterinary Sci. 6. doi: 10.3389/fvets.2019.00169
Yang W. R., Liao T. T., Bao Z. Q., Zhou C. Q., Luo H. Y., Lu C., et al. (2018). Role of AMPK in the expression of tight junction proteins in heat-treated porcine Sertoli cells. Theriogenology 121, 42–52. doi: 10.1016/j.theriogenology.2018.08.005
Yang T., Zhao Z., Liu T., Zhang Z., Wang P., Xu S., et al. (2017). Oxidative stress induced by Se-deficient high-energy diet implicates neutrophil dysfunction via Nrf2 pathway suppression in swine. Oncotarget 8 (8), 13428–13439. doi: 10.18632/oncotarget.14550
Yi D., Hou Y., Wang L., Ouyang W., Long M., Zhao D., et al. (2015). L-Glutamine enhances enterocyte growth via activation of the mTOR signaling pathway independently of AMPK. Amino Acids 47 (1), 65–78. doi: 10.1007/s00726-014-1842-8
Yildiz B. O., Suchard M. A., Wong M. L., McCann S. M., Licinio J. (2004). Alterations in the dynamics of circulating ghrelin, adiponectin, and leptin in human obesity. Proc. Natl. Acad. Sci. U.S.A. 101 (28), 10434–10439. doi: 10.1073/pnas.0403465101
Yin J., Li Y., Han H., Liu Z., Zeng X., Li T., et al. (2018). Long-term effects of lysine concentration on growth performance, intestinal microbiome, and metabolic profiles in a pig model. Food Funct. 9 (8), 4153–4163. doi: 10.1039/c8fo00973b
Yu C. L., Guan J. Y., Ding J., Huang S., Lian Y., Luo H. Y., et al. (2018). AMP-activated protein kinase negatively regulates heat treatment-induced lactate secretion in cultured boar sertoli cells. Theriogenology 121, 35–41. doi: 10.1016/j.theriogenology.2018.07.039
Yuan X. W., Han S. F., Zhang J. W., Xu J. Y., Qin L. Q. (2015). Leucine supplementation improves leptin sensitivity in high-fat diet fed rats. Food Nutr. Res. 59, 27373. doi: 10.3402/fnr.v59.27373
Zeitz J. O., Kaltenböck S., Most E., Eder K. (2019). Effects of L-methionine on performance, gut morphology and antioxidant status in gut and liver of piglets in relation to DL-methionine. J. Anim. Physiol. Anim. Nutr. 103 (1), 242–250. doi: 10.1111/jpn.13000
Zhang L., Li F., Guo Q., Duan Y., Wang W., Zhong Y., et al. (2020). Leucine supplementation: A novel strategy for modulating lipid metabolism and energy homeostasis. Nutrients 12 (5), 1–20. doi: 10.3390/nu12051299
Zhang J., Wang X., Vikash V., Ye Q., Wu D., Liu Y., et al. (2016). ROS and ROS-mediated cellular signaling. Oxid. Med. Cell. Longevity 2016, 4350965. doi: 10.1155/2016/4350965
Keywords: heat stress, pigs, ROS, oxidative stress, Nrf2, AMPK, mTOR, mitochondria
Citation: Montesinos-Cruz V, Parra Forero LY and García Contreras AdC (2023) Alterations in energy balance and the antioxidant system due to heat stress: focus on animal production. Front. Anim. Sci. 4:1275290. doi: 10.3389/fanim.2023.1275290
Received: 09 August 2023; Accepted: 15 November 2023;
Published: 01 December 2023.
Edited by:
Mayra A. D. Saleh, University of the Azores, PortugalReviewed by:
Ramona Rodriguez Gonzales, São Paulo State University, BrazilSarah C. Pearce, United States Department of Agriculture, United States
Copyright © 2023 Montesinos-Cruz, Parra Forero and García Contreras. This is an open-access article distributed under the terms of the Creative Commons Attribution License (CC BY). The use, distribution or reproduction in other forums is permitted, provided the original author(s) and the copyright owner(s) are credited and that the original publication in this journal is cited, in accordance with accepted academic practice. No use, distribution or reproduction is permitted which does not comply with these terms.
*Correspondence: Veronica Montesinos-Cruz, dm1vbnRlc2lub3NjcnV6M0B1bmwuZWR1