- 1Departamento de Producción Animal, Facultad de Veterinaria, Universidad Complutense de Madrid (UCM), Madrid, Spain
- 2Kemin Animal Nutrition and Health, Herentals, Belgium
- 3Ivars d’Urgell Cooperativa del Camp, Lleida, Spain
- 4Departamento de Producción Agraria, Escuela Técnica Superior de Ingeniería Agronómica, Alimentaria y de Biosistemas (ETSIAAB), Universidad Politécnica de Madrid (UPM), Madrid, Spain
Introduction: This study investigated the impact of rumen-protected amino acids on beef cattle's growth, fattening performance, and meat quality.
Methods: Two groups of 40 Montbeliard steers (237.8 ± 30 kg body weight) were housed separately and fed, with mono-tunnel feeders, either a control diet (CON) or a diet containing rumen-protected lysine and methionine with 3% less crude protein (APR). Feed consumption and weight were tracked in all animals, and meat quality analysis focused on the longissimus muscle, with 10 steers randomly selected from each treatment.
Results and discussion: Results for overall performance revealed no significant differences in body weight, average daily gain (ADG), and concentrate conversion ratio between the CON and APR diets (p > 0.05). However, during the final 90 days of the trial, the APR group showed significantly higher ADG (p < 0.05) than the CON group, while the concentrate conversion ratio was significantly higher (p < 0.05) in the CON group compared to the APR group. The hot carcass weight, dressing percentage, and carcass conformation did not differ significantly between the CON and APR diets (p > 0.05). There was a slight tendency (p = 0.06) for higher fatness score in the CON group. Regarding meat composition, the diet did not significantly affect proximate composition, but a tendency (p = 0.059) for higher crude protein content in APR cattle's meat compared to the CON group was observed. The APR meat showed slightly lower levels of C17:1 (0.56% vs. 0.72%) and C18:1n9 (31.7% vs. 34%). There was a tendency for C14:0 to be lower (P = 0.07), and for C16:0, C18:2 and polyunsaturated fatty acids (PUFA) to be greater in meat from cattle fed APR compared to CON. However, meat quality attributes like pH, water-holding capacity, color, and texture were similar in both CON and APR groups (p > 0.05). The findings indicate that utilizing rumen-protected amino acids enables formulating diets with reduced crude protein levels while enhancing nitrogen utilization efficiency for protein synthesis in intensively reared steers. Importantly, these dietary improvements do not adversely affect meat quality.
1 Introduction
Ruminant nutrition is influenced by various factors, such as protein quality, fiber content, feed type, rumen microorganisms, digestion, animal welfare, species, life stage, climate, and nutrient source (NRC, 2001). Among these, the quality of protein is more crucial than the quantity of protein for the growth, production, and reproduction of ruminants. The inefficient breakdown of high-protein diets in the rumen can lead to excess nitrogen release into the environment, contributing to the production of gases such as nitrous oxide, nitric oxide, nitrate, and ammonia, which pose environmental concerns (Abbasi et al., 2018). In cattle finishing diets, approximately 10% to 20% of the ingested nitrogen is stored in the animal’s tissues, while a significant portion of 30% to 50% is eliminated through feces. The majority of the remaining 40% to 70% of ingested nitrogen is excreted through urine (Cole and Todd, 2009). The Sustainable Development Goals (SDGs), set by the United Nations in 2015, emphasize the need to address poverty, hunger, and environmental preservation. Livestock farming is pivotal for addressing poverty and hunger (SDG 1 and SDG 2), but it must also align with goals focused on environmental preservation and ecosystem protection (SDG 13 and SDG 15) to ensure sustainable growth in the sector (Wanapat et al., 2015). Reducing crude protein (CP) in ruminants’ rations while maintaining animal performance could be a strategy to reduce nitrogen excretion and feed costs. In fact, dietary protein is the most important on-farm variable that can be controlled relatively easily with a significant effect on ammonia emissions (Agle et al., 2010). In dairy cows, Broderick (2003) observed that as dietary CP increased, there were linear increases in milk urea and urinary nitrogen (N) excretion, accompanied by linear decreases in nitrogen efficiency. Specifically, increasing CP from 15.1% to 18.4% resulted in a reduction in milk nitrogen from 31% to 25% of dietary nitrogen, an increase in urinary nitrogen from 23% to 35% of dietary nitrogen, and a decrease in fecal nitrogen from 45% to 41% of dietary nitrogen. In beef cattle, Todd et al. (2006) estimated that there would be an annual reduction in daily ammonia flux of 28% if CP was reduced from 13% to 11.5% in cattle diets.
The efficiency of metabolizable protein (MP) utilization in ruminants depends on the amino acid (AA) profile, as an inadequacy in any specific AA can restrict the use of others, even if they are present in sufficient quantities (Cole and Van Lunen, 1994; Klemesrud et al., 2000a). When certain AAs are lacking or imbalanced in the diet, the ruminant’s capacity to utilize MP is reduced, leading to decreased growth performance and overall productivity (Harper et al., 1964). Although the limitation of methionine (Met) and lysine (Lys) as the primary AAs in dairy cattle is widely acknowledged and documented (Sinclair et al., 2014; Abbasi et al., 2018), limited information is available for beef cattle. In diets with large proportions of corn, Lys is the first limiting AA for growing beef cattle because corn is low in Lys (Klemesrud et al., 2000a) but not in cattle fed diets containing soybean meal (Hussein and Berger, 1995). Therefore, ensuring that ruminant diets contain an optimal balance of AAs is crucial to promote efficient protein synthesis and maximize growth and productivity. This can be achieved through meticulous dietary management and the incorporation of rumen-protected AAs (RP-AAs) to supplement specific AAs as needed. RP-AAs have the advantage of bypassing the rumen and being absorbed directly in the small intestine, preventing degradation by rumen microbes and increasing their availability for absorption and utilization by the animal. However, there have been relatively few studies evaluating the effects of RP-AA supplementation in growing beef cattle, particularly in feeding systems involving cereal concentrates and ad libitum straw, with different results. Recently, Montaño et al. (2019) reported that supplementation with rumen-protected Met and Lys enhanced average daily gain (ADG) and gain efficiency during the early growing phase in Holstein calves. However, Barido et al. (2020) found that supplementation with Met and Lys had no effect on growth performance and carcass weight and yield in Hanwoo steers. Therefore, more research in this area is needed to better understand the benefits and applications of RP-AAs in beef cattle nutrition.
Building on previously published information, this study was conceived with the hypothesis that integrating protected AAs into a diet for beef cattle with reduced protein levels could potentially yield equivalent growth and meat quality to a diet with standard protein levels. The primary objective of this study was to evaluate the impact of incorporating RP-AAs into a diet with a 3% reduction in CP while increasing the levels of digestible Met and Lys. The study aimed to assess the effects on the growth and fattening performance of beef cattle, along with meat composition and quality.
2 Materials and methods
2.1 Animals and experimental diets
Forty Montbéliard steers, all the same age (158.6 days ± 11.9 days) and with an average body weight (BW) of 237.8 kg ± 30 kg, were split into two uniform groups and placed in separate housing. Each housing unit was equipped with a feeding system that allowed for the monitoring of weight and feed consumption of individual calves. To achieve this, each calf was uniquely identified using an electronic ear tag (transponder). The feeding unit was equipped with a radiofrequency identification (RFID) antenna, which detected the calf every time it approached to eat. This technology records data on feed consumption and calves’ weight each time they use the feeding unit. Consequently, although the calves were grouped homogeneously, the records for feed consumption and live weight were maintained for each calf. Each pen, which was naturally ventilated and fully covered, measured 7.0 m × 25.0 m, providing 8.75 m2 per calf. The pen included a mono-tunnel feeder featuring an automatic weighing scale for the animals and a 70-cm-long feed hopper with a 100-kg capacity. The pen was supplied with feed from a metal silo positioned above the hopper. In addition, there was a 15-m-long straw feeder and two 1.0-m-long constant-level drinkers in each enclosure. Twenty calves were randomly placed in each paddock and subjected to an experimental treatment. Ethics approval was not required for this study involving animals, as it complied with local legislation and institutional requirements. The study did not involve invasive procedures on the animals, and it was conducted on production animals.
The trial involved testing two diets: a control diet (CON) and a diet with 3% less CP containing RP-Lys LysiGEM™ and RP-Met KESSENT® MF Arome Dry (Kemin Animal Nutrition and Health, Belgium) (APR). Both diets had the same energy content (1.04 meat forage units; INRA, 2007), but they differed in CP content [13.3% in CON vs. 10.3% in APR; 9.4% of protein digestible in the intestine (PDI) in CON vs. 7.6% of PDI in APR, according to INRA (2007)]. The metabolizable Lys (LysDI) and metabolizable Met (MetDI) values were 5.7% and 1.7% of protein digestible in the intestine with energy limitation (PDIE) in CON (with a Lys-to-Met ratio of 3.35) and 6.0% and 2.6% PDIE in APR (with a Lys-to-Met ratio of 2.31), respectively. Both diets were evaluated using the Cornell Net Carbohydrate and Protein System (CNCPS) model (version 6.55), resulting in percentages of Lys and Met in MP of 6.1% and 2.2%, respectively, in CON (with a Lys-to-Met ratio of 2.78) and 6.9% and 2.7% in APR (with a Lys-to-Met ratio of 2.5). To determine the chemical composition of experimental diets, six samples were taken and analyzed for dry matter (DM), CP, ether extract, crude fiber, and ash using near-infrared spectroscopy (DS2500F; FOSS Barcelona, Spain), with a wavelength range of 850 nm to 2,500 nm. The ingredients and the chemical composition of the diets are shown in Table 1.
Throughout the 202-day trial, the animals were provided with unrestricted access to high-quality water and straw. After a 15-day adaptation period to the experimental diets, the weights (kg) and daily feed consumption (kg FM/day) for each calf were recorded daily through software linked to the feeding unit. Once the concentrate intake and growth rate for each calf were determined, their concentrate conversion ratio (concentrate intake/growth rate) was calculated. The average daily gain, concentrate intake, and concentrate conversion ratio were computed for three distinct periods: days 0 to 112 (period 1), days 113 to slaughter (period 2), and days 0 to slaughter (overall).
2.2 Slaughter measurement and sampling
At the end of the trial, all 40 study calves were transported to a commercial slaughterhouse, located 332 km away in the province of Valencia (Spain), for processing. Hot carcass weight (HCW) was determined immediately after evisceration. Carcasses’ dressing percentage was calculated as the hot carcass weight divided by their live weight 24 h before slaughter.
The carcasses were then assessed for conformation and fatness, following the EUROP classification system. The conformation score ranged from 15 (indicating very good conformation) to 1 (indicating very bad conformation), with corresponding EUROP grades such as E+, E, E−, U+, U, U−, R+, R, R−, O+, O, O−, P+, P, and P−. The fatness score was evaluated on a scale of 1 to 15, with 1 indicating very low fat and 15 indicating very high fat. There was also a 15-point classification, with the equivalence of the scale as follows: 1−, 1, 1+, 2−, 2, 2+, 3−, 3, 3+, 4−, 4, 4+, 5−, 5, and 5+ (Piedrafita et al., 2003). For the meat quality and composition analysis, 10 steers from each treatment were randomly selected. After chilling the carcasses at 2°C for 24 h, the sixth rib on the left side of the carcass was dissected, as described by Prado et al. (2014), ensuring a minimum thickness of 2.5 cm. The longissimus muscle was then separated, placed in aluminum bags, vacuum packaged, and frozen at −21°C until analysis. The samples of the longissimus muscle were freeze-dried using LyoQuest −55 (Telstar, Barcelona, Spain), to determine their proximate, fatty acid, and AA compositions.
Before conducting the meat quality analysis, the samples were thawed at 4°C for 24 h within their bags.
2.3 Meat composition
For the proximate composition, moisture content (AOAC 950.46 method), protein (AOAC 992.15 method), fat (AOAC 960.39 method), and ash (AOAC 920.153 method) were analyzed using official methods from the Association of Official Agricultural Chemists (AOAC; AOAC, 2007).
For the analysis of fatty acids, meat samples were converted into fatty acid methyl esters (FAMES) following the procedure described by Lee et al. (2012). The FAMES were then analyzed using a gas chromatograph (Varian CP-3800; Agilent, USA) equipped with a flame ionization detector and a SP-2560 column (Supelco, Bellefonte, PA, USA). During the analysis, 1.0 µL of the sample was injected in split mode at a 1 : 30 split ratio. The detector and injector oven temperatures were set at 260°C. The oven temperature profile started at 140°C for 5 min and was increased by 4°C per min up to 240°C, and then rapidly increased by 20°C in 1 min up to 260°C, where it was held for 15 min. The identification of FAMES was achieved by comparing them with a standard FAME mixture (Supelco® 37-component FAME Mix; Sigma Aldrich). The results were expressed as a percentage of the total identified FAMES.
The AAs in muscle were analyzed by the Instrumental Techniques Laboratory of the University of Valladolid (UVA, Valladolid, Spain), according to the method proposed by Greene et al. (2009). The samples were hydrolyzed with a microwave in an acid medium (6 N HCl) and an inert atmosphere. Next, the acid medium was removed with a stream of N2. It was redissolved in 0.1 N HCl and filtered through a 0.22-μm nylon membrane into the high-pressure liquid chromatography (HPLC) vials. The AA profile was determined using a Zorbax Eclipse Plus AAA column (Agilent, USA) and UV detector with HPLC (1260 Infinity II; Agilent, USA). The results were expressed in mg/g of dry matter.
2.4 Meat quality parameters
The meat’s pH was measured using a Hanna Instruments HI-9025 pH meter (Hanna Instruments S.L., Spain). The calibration was previously carried out with buffers with pH values of 7.0 and 4.0, protected from light, and stored at a low temperature.
Water-holding capacity (WHC) was determined through centrifugation according to Bouton et al. (1971) with modifications. In brief, a 0.3-g sample of the meat was centrifuged at 10,000 rpm for 10 min using centrifugal tubes with an acetate filter (0.2 µm). Two replicates were performed for each sample. The amount of water expelled was determined by weighing the sample before and after centrifugation.
The color coordinates of the longissimus muscle were expressed using the CIELAB system (Commission Internationale de l’Eclairage). The measurements of L* (lightness), a* (redness index), and b* (yellowness index) were obtained with a Minolta CM 2600d reflectometer-colorimeter (I.T.A. Aquateknica, SA, Valencia, Spain) using the following settings: the illuminant D65 and a visual angle of 10°. The chroma [C* = √(a2 + b2)] and hue [h* = arctan (b*/a*)] were calculated using the values of a* (ranging from green to red) and b* (ranging from blue to yellow), respectively.
The meat samples were subjected to texture analysis after being cooked in a water bath until their internal temperature reached 70°C. The analysis was conducted using a TA-XT2 Texture Analyser® (Stable Micro Systems, Surrey, UK) equipped with a Warner–Bräzler device (with a 25-kg load cell and a 2 mm/s crosshead speed). Prisms with a 1 cm × 1 cm cross-section were cut parallel to the muscle fiber direction, and at least eight prisms were used for each sample. The measured parameters included maximum shear force (kg/cm2), shear firmness (kg/s cm2), and total area, as defined as the total work performed to cut the sample or the area under the curve (toughness, kg/s cm2).
2.5 Statistical analysis
The statistical analysis was conducted using SAS software (SAS version 9.0; SAS Institute (2002) Inc., Cary, NC, USA) considering an individual calf as an experimental unit. Prior to the analysis, the normality and homogeneity of variance were assessed for all variables using the Shapiro–Wilk test and the Bartlett test, respectively. Once it was validated that all variables complied with the criteria for an ANOVA, the data were then analyzed using a one-way ANOVA, with diet as a fixed factor. The significant differences were established with p-value < 0.05. Values of p < 0.1 were discussed as trends.
3 Results
3.1 Animal performance and carcass characteristics
There were no significant differences observed in the body weight and concentrate intake between diets (p > 0.10; Table 2). In addition, the treatments did not affect (p > 0.10) the ADG during the first period, from 0 days to 112 days, or overall (0–202 days). However, animals fed the APR diet had a greater ADG than those fed the CON diet from 112 to 202 days (1.55 kg/day vs. 1.33 kg/day; p < 0.05). Likewise, the concentrate conversion ratio was similar for both diets (CON and APR) from 0 days to 112 days and overall. In contrast, from days 112 to 202, cattle fed the CON diet had a higher conversion ratio than those fed the APR diet (7.80 kg/kg vs. 6.53 kg/kg; p < 0.05).
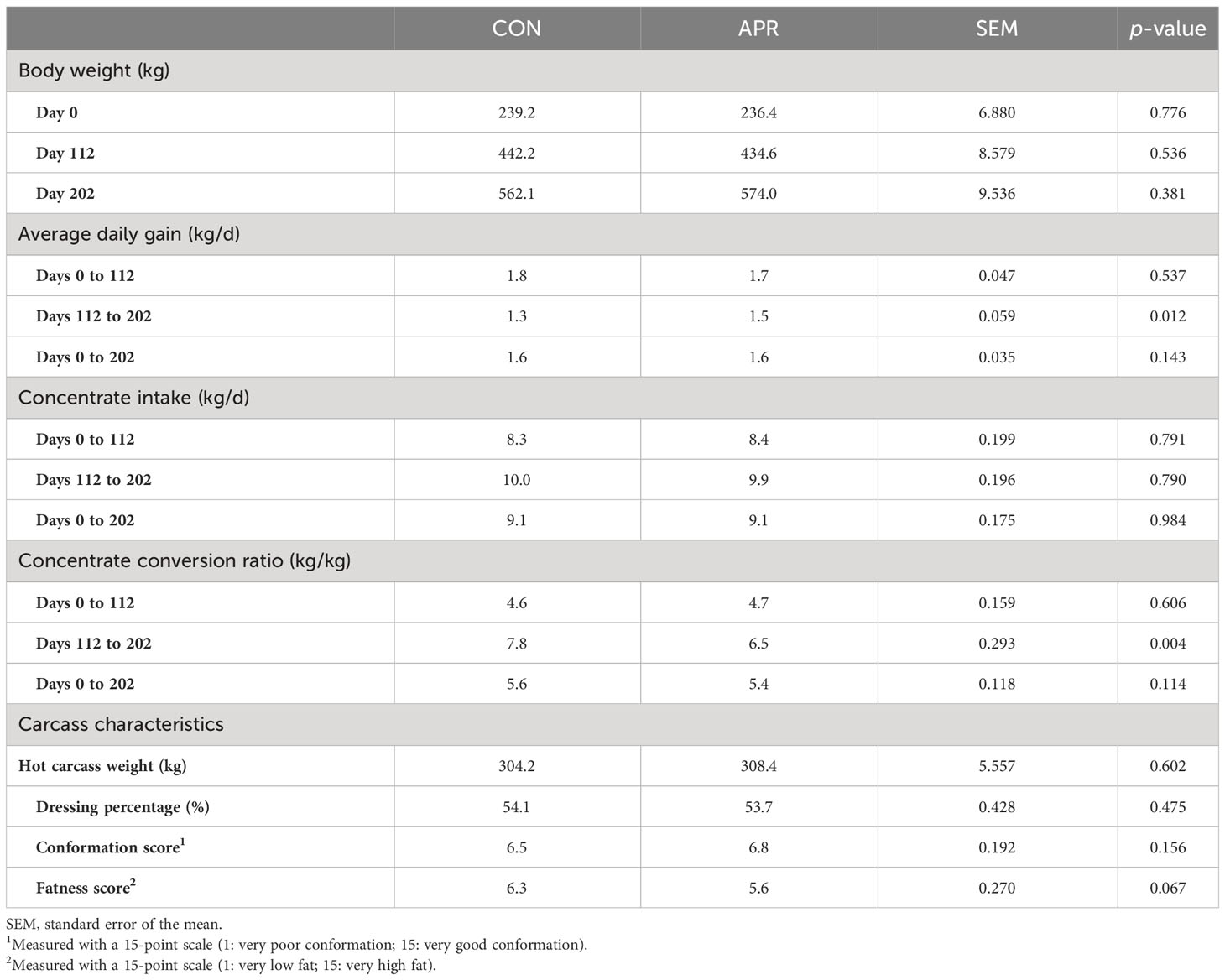
Table 2 Animal performance and carcass characteristics from the cattle fed the control diet (CON) and the diet supplemented with protected amino acids (APR).
The hot carcass weight, dressing percentage, and carcass conformation showed no significant difference between the CON and APR diets (p > 0.05; Table 2). However, there was a tendency (p = 0.06) for the fatness score to be slightly higher in the CON group than in the APR group (6.30 vs. 5.58).
3.2 Meat composition
Table 3 shows the proximate composition of meat from cattle fed the control diet (CON) and the diet supplemented with protected AAs (APR). There was a trend (p = 0.059) for CP content, with higher protein content in the meat of APR cattle than CON cattle (18.6% vs. 16.8%). No differences were observed (p > 0.10) for the other meat proximate composition parameters (moisture, fat, and ash).

Table 3 Proximate composition of meat from the cattle fed the control diet (CON) or the diet supplemented with protected amino acids (APR).
The cattle fed the APR diet, supplemented with Met and Lys, produced meat with lower levels of C17:1 (0.56% vs. 0.72%; p = 0.04) and C18:1n9 (31.7% vs. 34%; p = 0.04) (see Table 4) and a higher concentration of C18:1t11 (8.95% vs. 7.77%; p = 0.04) than cattle on the CON diet. Furthermore, the meat from animals fed the APR diet showed a tendency toward lower levels of C14:0 (p = 0.07) and higher levels of C16:0 (p = 0.08), C17:0 (p = 0.07), C18:2 n6c (p = 0.08), and PUFAs (p = 0.06) than cattle on the CON diet. However, the diet did not have a significant impact (p > 0.10) on the total levels of saturated fatty acids (SFAs) and monounsaturated fatty acids (MUFAs).
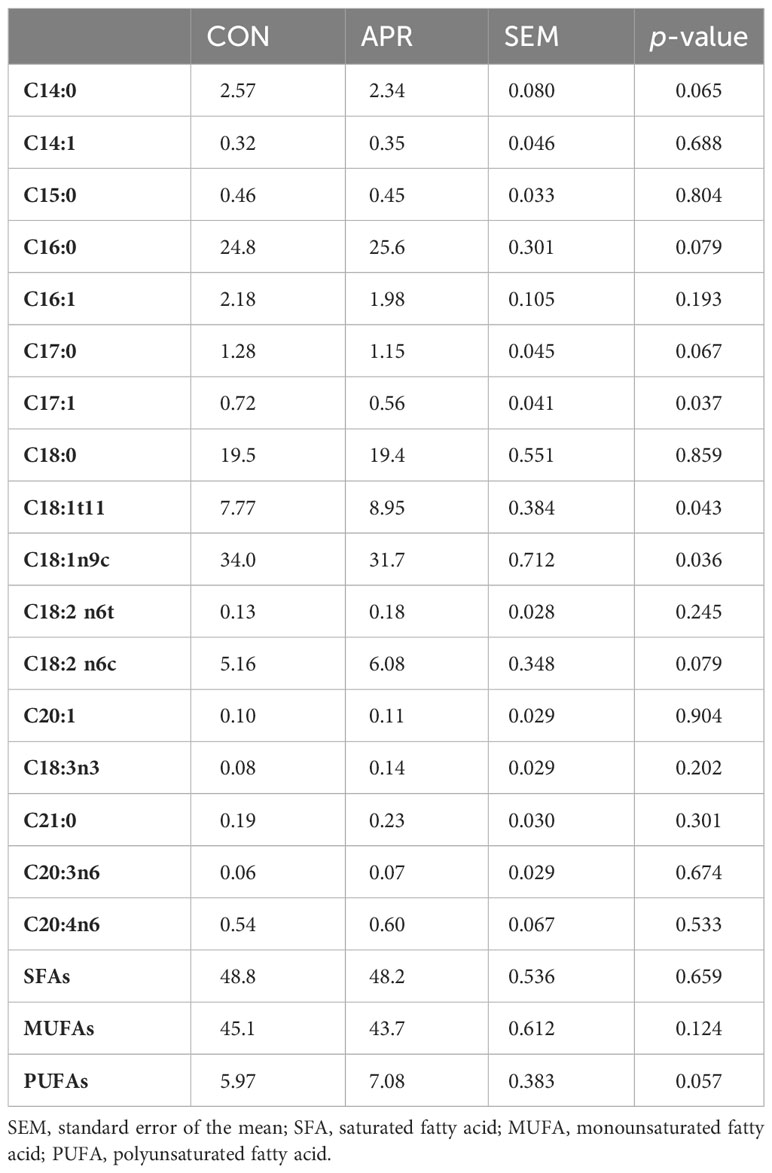
Table 4 Fatty acid composition (%) of meat from the cattle fed the control diet (CON) or the diet supplemented with protected amino acids (APR).
Table 5 displays the AA profiles of the longissimus, and no significant differences (p > 0.05) were observed in both essential and non-essential AAs between the CON and APR diets. Specifically, the Lys content of the meat of the CON cattle was 71.20 mg/g and 70.30 mg/g in the APR cattle. Similarly, the met content in the longissimus was 17.88 mg/g for the CON diet and 17.79 mg/g for the APR diet.
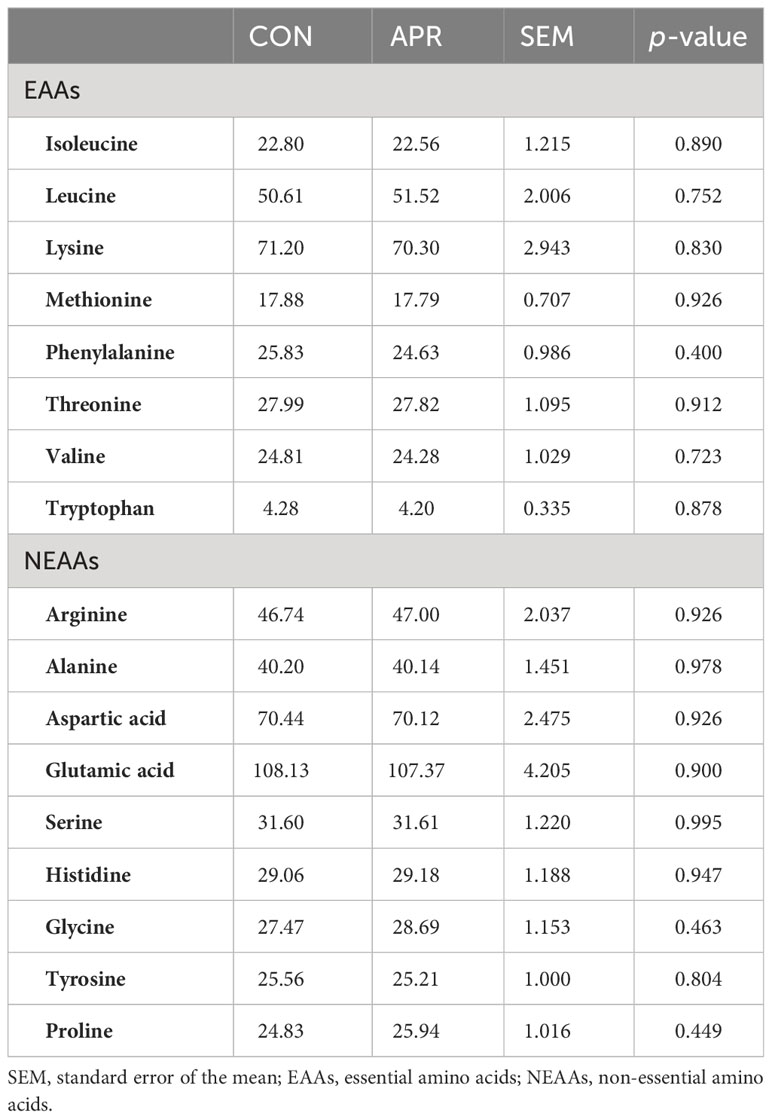
Table 5 Amino acid profiles (mg/g) of meat from the cattle fed the control diet (CON) and the diet supplemented with protected amino acids (APR).
3.3 Meat quality
The diet did not affect pH values (p > 0.05), with a mean pH of 5.60 (Table 6). Similarly, the percentage of expelled juice was similar in meat from CON and APR cattle. The color parameters were not affected by diet, with similar L*, a*, and b* values for CON and APR meat. In relation to texture parameters, meat from CON and APR cattle had similar tenderness, with no differences between groups (p > 0.05) in shear force, total area, or shear firmness.
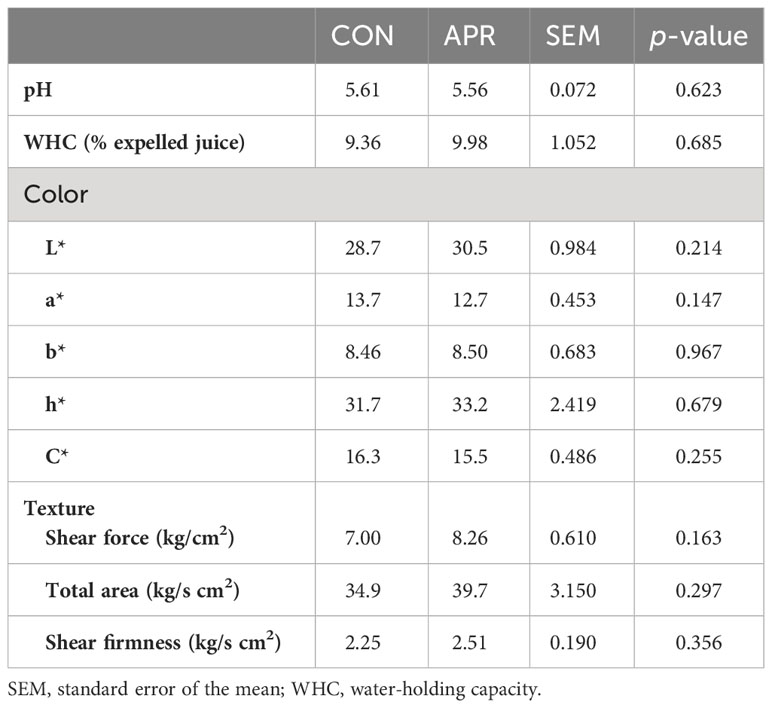
Table 6 Meat quality characteristics from the cattle fed the control diet (CON) or the diet supplemented with protected amino acids (APR).
4 Discussion
The greater ADG in the 90 days of the trial for animals fed the APR diet could be explained by the better utilization of the N provided in the APR diet in the fattening phase, when less growth occurs, which leads to a lower energetic cost of eliminating the N that is not used by the animal. As cattle increase in body weight and level of fatness, the rate of protein deposition decreases, leading to a reduction in their MP requirements (Teixeira et al., 2019). Logically, future research work is required to explain the better performance in animals fed less CP. Similar findings were reported by Prado et al. (2014), who observed no significant differences in animal performance between those with low- and high-protein diets (14.6% CP vs. 16.8% CP) and different Lys-to-Met ratios (3.0 vs. 3.4). However, the growth response to supplementing diets with protected AAs exhibited variability in previous studies (Klemesrud et al., 2000a; Klemesrud et al., 2000b; Schroeder and Titgemeyer, 2008).
Mazinani et al. (2020) suggested that adding protected AAs may have some benefits for these parameters by enhancing rumen undegradable protein. Furthermore, Montaño et al. (2019) found improved daily gains and feed efficiency in Holstein steers fed a rumen-protected Met and Lys diet. In their study, the consumption of these protected AAs increased the availability of Met and Lys in the intestine, post-ruminal segments, and the entire gastrointestinal tract.
According to Weekes et al. (2006), either a deficiency or imbalance in the AA profiles within MP can inhibit the growth performance and feed conversion efficiency of livestock. Heiderscheit and Hansen (2020) conducted an evaluation of supplemental RP-Lys in Angus crossbred steers. Their hypothesis was that steers fed diets formulated to meet Lys requirements would exhibit better feed efficiency and greater gains than those fed a Lys-deficient diet. However, they found that the ADG throughout the entire trial was higher in the control diet, which was deficient in Lys, than the diet formulated to meet Lys requirements using ingredients other than RP-Lys. They suggest that growth performance responses in RP-Lys-fed steers might have been negatively affected by overconsumption of rumen-bypass Lys during the finishing phase. Nevertheless, these authors concluded that diets formulated to meet Lys requirements resulted in positive metabolizable Lys balances during finishing. The increased metabolizable Lys balance may have impacted performance if other AAs were imbalanced. Ma et al. (2021) observed differences in the ADG and feed-to-gain ratio in yaks in a feedlot with four supplementary levels of RP-Lys and Met in the diets. They concluded that growth performance and meat quality could be improved by supplementing RP-AAs in the diets.
Teixeira et al. (2019) also observed that steers fed a supplemental RP-Lys diet did not experience changes in dressing percentage, but did produce carcasses with reduced fat thickness and increased longissimus muscle area. These findings led them to conclude that the RP-Lys diet improved nitrogen utilization, indicating the enhanced utilization of AAs for anabolic purposes and increased protein accretion and muscle synthesis, as we observed in the APR group, which increased protein content in the meat. Heiderscheit and Hansen (2020) reported no differences in the carcass characteristics of Angus crossbred steers fed supplemental RP-Lys diets. Similarly, Ma et al. (2021), in their study with different levels of protected Lys and Met, found no differences in carcass characteristics. In diets with varying levels of CP and Lys-to-Met ratios, Prado et al. (2014) did not observe significant differences in carcass conformation and fatness score. In addition, Barido et al. (2020) found that meat from cattle supplemented with Met and Lys had higher protein content than meat from non-supplemented cattle. Similarly, Teixeira et al. (2019) conducted a study on Angus × Simmental steers that were fed diets with RP-AAs, and they suggested that Lys supplementation increased protein accretion and muscle synthesis. These observations made them deduce that the RP-Lys regimen boosted nitrogen assimilation, pointing to an improved uptake of AAs for growth activities and heightened protein deposition and muscle development.
The fatty acid composition of cattle meat can be influenced by various factors, including breed, weight, sex, age, and diet (De la Fuente et al., 2009). However, in the context of diet, the fatty acid profile of ruminant meat is relatively unaffected due to ruminal modifications, but it can still reflect differences in the composition of dietary lipids (Díaz et al., 2005). In addition, lipid metabolism can be influenced by the balance of AAs in the diet (Martínez et al., 2017). Research has demonstrated that restricting essential AAs in the diet, such as Met, can result in substantial alterations in lipid metabolism within adipose and hepatic tissues. These changes trigger significant transcriptional and metabolic responses, including a reduction in transcriptional initiation and the expression of the enzyme fatty acid synthase (Anthony et al., 2013), which is responsible for the de novo biosynthesis of fatty acids. Published literature on the effects of RP-AAs on the fatty acid composition of ruminant meat is scarce and presents highly variable results. For instance, Barido et al. (2020) observed only minor effects of dietary Met and Lys supplementation on intramuscular fatty acid composition in Hanwoo cattle, with C14:0 being the only fatty acid affected. The present study found that the effect of diet on the intramuscular fatty acid profile was limited, and, therefore, cattle fed the APR diet, supplemented with Met and Lys, produced meat with slightly lower levels of C18:1n9 and greater C16:0 than the CON meat. For example, Třináctý et al. (2006) observed a reduced proportion of C18:1 in milk fat from cows that were supplemented with RP-AAs (Lys, Met, and histidine) compared with cows that were not supplemented. This finding suggests that Met may influence the de novo synthesis of fatty acids, potentially increasing the production of short- and medium-chain fatty acids within the mammary gland (Pisulewski et al., 1996). In this context, C16:0 emerges as the predominant end product of de novo synthesis by fatty acid synthase (Smith et al., 2003). In our study, the lower proportion of C18:1n9 and greater C16:0 in cattle fed the APR diet could be attributed to Met supplementation, but it might also be influenced by the different composition of the diets provided. Further research needs to be conducted to better understand the mechanism of the AAs’ effect on meat fatty acid composition. Other researchers have ascribed a partial effect of AA lipid coating on the fatty acid composition of milk (Sevi et al., 1998). In our case, the surface coating used for Met protection was palmitic acid, which could partly explain the tendency toward a higher proportion of C16:0 in the APR meat. In the case of growing lambs, Dong et al. (2020) demonstrated that betaine, a choline metabolite closely related to Met, supplementation increased the PUFA content of the gluteus muscles, similar to the results of our study, with near to 1% more PUFAs in APR than in CON meat. In this study, the inclusion of RP-AAs and the reduction of CP levels did not have a significant effect on the AA content of the longissimus muscle. Similarly, Ma et al. (2021) found no significant differences in the AA profile of the longissimus muscle in feedlot yaks with various levels of supplementary RP-AAs in the diet. Similarly, Xue et al. (2010) found no differences in the meat AA profile of Limousin-crossbred calves fed RP-Lys. Teixeira et al. (2019) noticed a tendency for Lys levels in serum to decrease when arginine was added to the diet, suggesting that excessive arginine intake might hinder Lys absorption from the small intestine. However, they reported no notable variations in plasma Lys levels among calves consuming diets containing RP-Lys.
The ultimate pH values were in accordance with those obtained by Thénard et al. (2006) in Montbéliard cattle, and no differences were observed in the meat from cattle fed the CON diet and the APR diet. The pH values were below 5.6, indicating that the animals were not stressed at slaughter (Prado et al., 2014). Similar results were obtained by Barido et al. (2020), who reported no effect of dietary supplementation with rumen-protected Met/Lys on pH at 0 h of longissimus lumborum muscle from Hanwoo steers. In yak meat, Ma et al. (2021) obtained ultimate pH values of 5.44, with no differences due to the supplementary level of RP-AAs. Likewise, the WHC was not affected by diet, with a similar percentage of expelled juice in meat from cattle fed the CON diet and the APR diet supplemented with Met and Lys, in accordance with similar ultimate pH values found in the CON and APR meat. The WHC indicates variations in the charges and structure of muscle proteins, and is closely related to pH (Bouton et al., 1971).
In ruminants, the papers published about the impact of RP-AAs on meat quality are scarce and present variable results. Some authors have observed an effect of Met supplementation on ruminant meat color (Dong et al., 2020; Ma et al., 2021). Ma et al. (2021) noted an impact of dietary supplementation with RP-AAs (Met and Lys) in feedlot yaks, resulting in changes in meat color. They reported increased a* values and decreased L* and b* values in the supplemented groups compared with the control group. In the case of growing lambs, Dong et al. (2020) observed a linear decrease in the b* value of the longissimus muscle with increasing doses of rumen-protected betaine. However, our study did not show any significant effect on meat color, as we observed similar L*, a*, and b* values in both the CON and the APR meat samples. It has been reported that some AAs, including Met, have antioxidant activity (Levine et al., 2000; Lund et al., 2011), improving muscular antioxidant status and enhancing meats’ stability against oxidation. Myoglobin oxidation during storage affects the extent of meat discoloration depend on the length of the storage period, packaging conditions, and storage temperature (Olivera et al., 2013). In the present study, meat samples were stored through vacuum packaging and at congelation temperature. These conditions minimize oxidation (Vieira et al., 2009). This could partially explain the lack of effect of diet on meat color.
Despite the differences in protein content between the CON and APR meat, no significant effect of diet was recorded for texture parameters. According to Koohmaraie et al. (2002), changes in muscle protein synthesis do not affect meat tenderness; however, changes in muscle protein degradation affect meat tenderization/tenderness. Similarly, Prado et al. (2014) did not report differences in texture parameters (shear force) due to diet protein level or Lys-to-Met ratio. However, they reported lower values of shear force (4.63 kg) than in our study. The differences between studies could be due to the different breeds used, which imply differences in the quantity and solubility of collagen, fatness, and calpain and calpastatin activity (Monsón et al., 2005), which affect tenderness, but are also due to differences in aging time (Vieira et al., 2009) between studies.
5 Conclusion
The findings of this study demonstrate that the inclusion of RP-AAs in cattle fattening diets offers several benefits. It allows for a reduction in CP content without compromising productive yields or the quality of the meat produced. Moreover, it may enhance the efficiency of the utilization of nitrogen from the diet, thereby potentially reducing environmental pollution. In summary, incorporating RP-AAs in cattle diets presents a promising approach for beef producers in southern Europe. It may not only mitigate the challenges associated with volatile raw material prices and deforestation but also offer environmental benefits while maintaining cattle productivity and the quality of the meat produced.
Data availability statement
The raw data supporting the conclusions of this article will be made available by the authors, without undue reservation.
Ethics statement
Ethics approval was not necessary, as the study was carried out on production animals, and no invasive procedures were performed on them.
Author contributions
AC: Conceptualization, Data curation, Formal analysis, Investigation, Methodology, Project administration, Resources, Software, Supervision, Validation, Visualization, Writing – original draft, Writing – review & editing. JD: Data curation, Formal analysis, Investigation, Writing – review & editing, Validation, Writing – original draft. MD: Data curation, Formal analysis, Methodology, Software, Writing – original draft, Writing – review & editing. RB: Data curation, Formal analysis, Software, Visualization, Writing – original draft, Writing – review & editing. Dd: Funding acquisition, Investigation, Writing – original draft, Writing – review & editing. JM: Funding acquisition, Investigation, Writing – original draft, Writing – review & editing. NL: Data curation, Resources, Writing – original draft, Writing – review & editing. VJ: Conceptualization, Funding acquisition, Investigation, Methodology, Project administration, Writing – original draft, Writing – review & editing.
Funding
The author(s) declare that financial support was received for the research, authorship, and/or publication of this article. This project was financed by Kemin-Fundación Premio Arce (ETSIAAB-UPM), project FPA-220000PA1511.
Conflict of interest
The authors declare that the research was conducted in the absence of any commercial or financial relationships that could be construed as a potential conflict of interest.
Publisher’s note
All claims expressed in this article are solely those of the authors and do not necessarily represent those of their affiliated organizations, or those of the publisher, the editors and the reviewers. Any product that may be evaluated in this article, or claim that may be made by its manufacturer, is not guaranteed or endorsed by the publisher.
References
Abbasi I. H. R., Abbasi F., Abd El-Hack M. E., Abdel-Latif M. A., Soomro R. N., Hayat K., et al. (2018). Critical analysis of excessive utilization of crude protein in ruminants ration: impact on environmental ecosystem and opportunities of supplementation of limiting amino acids—a review. Environ. Sci. pollut. 25, 181–190. doi: 10.1007/s11356-017-0555-4
Agle M., Hristov A. N., Zaman S., Schneider C., Ndegwa P., Vaddella V. K. (2010). The effects of ruminally degraded protein on rumen fermentation and ammonia losses from manure in dairy cows. J. Dairy Sci. 93 (4), 1625–1637. doi: 10.3168/jds.2009-2579
Anthony T. G., Morrison C. D., Gettys T. W. (2013). Remodeling of lipid metabolism by dietary restriction of essential amino acids. Diabetes 62, 2635–2644. doi: 10.2337/db12-1613
AOAC. (2007). Association of official analytical chemists. official methods of analysis, 18nd (Arlington, VA, USA: AOAC).
Barido F. H., Utama D. T., Jeong H. S., Kim J., Lee C. W., Park Y. S., et al. (2020). The effect of finishing diet supplemented with methionine/lysine and methionine/α-tocopherol on performance, carcass traits and meat quality of Hanwoo steers. Asian-australas. J. Anim. Sci. 33, 69–78. doi: 10.5713/ajas.19.0209
Bouton P. E., Harris P. T., Shorthose W. R. (1971). Effect of ultimate pH upon the water-holding capacity and tenderness of mutton. J. Food Sci. 36, 435–439. doi: 10.1111/j.1365-2621.1971.tb06382.x
Broderick G. A. (2003). Effects of varying dietary protein and energy levels on the production of lactating dairy cows. J. Dairy Sci. 86 (4), 1370–1381. doi: 10.3168/jds.S0022-0302(03)73721-7
Cole N. A., Todd R. W. (2009). “Nitrogen and phosphorus balance of beef cattle feedyards.” in Proceedings of the Texas animal manure management issues conference. (Round Rock, TX, USA: U.S. Department of Agriculture), 17–24.
Cole D. J. A., Van Lunen T. A. (1994). “(Chapter 5) Ideal amino acid patterns,” in Amino Acids in Farm Animal Nutrition. Ed. D’ Mello J. F. P. (Wallingford, Oxon, UK: CAB International).
De la Fuente J., Díaz M. T., Alvarez I., Oliver M. A., Font I Furnols M., Sañudo C., et al. (2009). Fatty acid and vitamin E composition of intramuscular fat in cattle reared in different production systems. Meat Sci. 82, 331–337. doi: 10.1016/j.meatsci.2009.02.002
Díaz M. T., Alvarez I., de la Fuente J., Sañudo C., Campo M. M., Oliver M. A., et al. (2005). Fatty acid composition of meat from typical lamb production systems of Spain, United Kingdom, Germany and Uruguay. Meat Sci. 71, 256–263. doi: 10.1016/j.meatsci.2005.03.020
Dong L., Zhong Z. X., Cui H. H., Wang S. N., Luo Y., Yu L. H., et al. (2020). Effects of rumen-protected betaine supplementation on meat quality and the composition of fatty and amino acids in growing lambs. Animal 14, 435–444. doi: 10.1017/S1751731119002258
Greene J., Henderson J. W. Jr., Wikswo J. P. (2009). Rapid and precise determination of cellular amino acid flux rates using HPLC with automated derivatization with absorbance detection. Agilent Technol., 1–8.
Harper A. E., Leung P., Yoshida A., Rogers Q. R. (1964). Some new thoughts on amino acid imbalance. Fed. Proc. 23, 1087–1092.
Heiderscheit K. J., Hansen S. L. (2020). Effect of rumen-protected lysine on growth performance, carcass characteristics, and plasma amino acid profile in feedlot steers. Transl. Anim. Sci. 4, 1–9. doi: 10.1093/tas/txaa128
Hussein H. S., Berger L. L. (1995). Feedlot performance and carcass characteristics of Holstein steers as affected by source of dietary protein and level of ruminally protected lysine and methionine. J. Anim. Sci. 73 (12), 3503–3509. doi: 10.2527/1995.73123503x
INRA. (2007). Alimentation des bovins, ovins et caprins-besoins des animaux –Valeurs des Aliments – Tables INRA 2007 (Versailles, France: Editions Quae).
Klemesrud M. J., Klopfenstein T. J., Lewis A. J. (2000b). Metabolizable methionine and lysine requirements of growing cattle. J. Anim. Sci. 78, 199–206. doi: 10.2527/2000.781199x
Klemesrud M. J., Klopfenstein T. J., Stock R. A., Lewis A. J., Herold D. W. (2000a). Effect of dietary concentration of metabolizable lysine on finishing cattle performance. J. Anim. Sci. 78, 1060–1066. doi: 10.2527/2000.7841060x
Koohmaraie M., Kent M. P., Shackelford S. D., Veiseth E., Wheeler T. L. (2002). Meat tenderness and muscle growth: is there any relationship? Meat Sci. 62, 345–352. doi: 10.1016/S0309-1740(02)00127-4
Lee M. R. F., Tweed J. K. S., Kim E. J., Scollan N. D. (2012). Beef, chicken and lamb fatty acid analysis—A simplified direct bimethylation procedure using freeze-dried material. Meat Sci. 92 (4), 863–866. doi: 10.1016/j.meatsci.2012.06.013
Levine R. L., Moskovitz J., Stadtman E. R. (2000). Oxidation of methionine in proteins: roles in antioxidant defense and cellular regulation. IUBMB Life 50, 301–307. doi: 10.1080/713803735
Lund M. N., Heinonen M., Baron C. P., Estévez M. (2011). Protein oxidation in muscle foods: a review. Mol. Nutr. Food Res. 55, 83–95. doi: 10.1002/mnfr.201000453
Ma Z., Zhao Z., Wang H., Zhou J., Zhang C. (2021). Effect of supplementary levels of rumen-protected lysine and methionine on growth performance, carcass traits, and meat quality in feedlot Yaks (Bos grunniens). Animals 11, 3384. doi: 10.3390/ani11123384
Martínez Y., Li X., Liu G., Bin P., Yan W., Más D., et al. (2017). The role of methionine on metabolism, oxidative stress, and diseases. Amino Acids 49, 2091–2098. doi: 10.1007/s00726-017-2494-2
Mazinani M., Naserian A. A., Rude B. J., Tahmasbi A. M., Valizadeh R. (2020). Effects of feeding rumen–protected amino acids on the performance of feedlot calves. J. Adv. Vet. Anim. 7 (2), 229–233. doi: 10.5455/javar.2020.g414
Monsón F., Sañudo C., Sierra I. (2005). Influence of breed and ageing time on the sensory meat quality and consumer acceptability in intensively reared beef. Meat Sci. 71, 471–479. doi: 10.1016/j.meatsci.2005.04.026
Montaño M. F., Chirino J. O., Latack B. C., Salinas-Chavira J., Zinn R. A. (2019). Influence of supplementation of growing diets enriched with rumen-protected methionine and lysine on feedlot performance and characteristics of digestion in Holstein steer calves. Appl. Anim. Sci. 35 (3), 318–324. doi: 10.15232/aas.2019-01843
NRC (2001). National Research Council. Nutrient Requirements of Dairy Cattle. 7th rev. ed (Washington, DC: Natl. Acad. Sci.).
Olivera D. F., Bambicha R., Laporte G., Cárdenas F. C., Mestorino N. (2013). Kinetics of colour and texture changes of beef during storage. J. Food Sci. Technol. 50, 821–825. doi: 10.1007/s13197-012-0885-7
Piedrafita J., Quintanilla R., Sañudo C., Olleta J. L., Campo M. M., Panea B., et al. (2003). Carcass quality of 10 beef cattle breeds of the Southwest of Europe in their typical production systems. Livest. Prod. Sci. 82 (1), 1–13. doi: 10.1016/S0301-6226(03)00006-X
Pisulewski P. M., Rulquin H., Peyraud J. L., Verite R. (1996). Lactational and systemic responses of dairy cows to postruminal infusions of increasing amounts of methionine. J. dairy Sci. 79 (10), 1781–1791. doi: 10.3168/jds.S0022-0302(96)76546-3
Prado I. N., Campo M. M., Muela E., Valero M. V., Catalan O., Olleta J. L., et al. (2014). Effects of castration age, dietary protein level and lysine/methionine ratio on animal performance, carcass and meat quality of Friesian steers intensively reared. Animal 8 (9), 1561–1568. doi: 10.1017/S1751731114001591
Schroeder G. F., Titgemeyer E. C. (2008). Interaction between protein and energy supply on protein utilization in growing cattle: a review. Livestock Sci. 114, 1–10. doi: 10.1016/j.livsci.2007.12.008
Sevi A., Rotunno T., Di Caterina R., Muscio A. (1998). Rumen-protected methionine or lysine supplementation of Comisana ewes' diets: Effects on milk fatty acid composition. J. Dairy Res. 65, 413–422. doi: 10.1017/S0022029998002945
Sinclair K. D., Garnsworthy P. C., Mann G. E., Sinclair L. A. (2014). Reducing dietary protein in dairy cow diets: implications for nitrogen utilization, milk production, welfare and fertility. Animal 8 (2), 262–274. doi: 10.1017/S1751731113002139
Smith S., Witkowski A., Joshi A. K. (2003). Structural and functional organization of the animal fatty acid synthase. Prog. Lipid Res. 42 (4), 289–317. doi: 10.1016/s0163-7827(02)00067-x
Teixeira P. D., Tekippe J. A., Rodrigues L. M., Ladeira M. M., Pukrop J. R., Kim Y. B., et al. (2019). Effect of ruminally protected arginine and lysine supplementation on serum amino acids, performance, and carcass traits of feedlot steers. J. Anim. Sci. 97 (8), 3511–3522. doi: 10.1093/jas/skz191
Thénard V., Dumont R., Grosse M., Trommenschlager J. M., Fiorelli J. L., Roux M. (2006). Grass steer production system to improve carcass and meat quality. Livest. Sci. 105, 185–197. doi: 10.1016/j.livsci.2006.06.008
Todd R. W., Cole N. A., Clark R. N. (2006). Reducing crude protein in beef cattle diet reduces ammonia emissions from artificial feedyard surfaces. J. Environ. Qual. 35 (2), 404–411. doi: 10.2134/jeq2005.0045
Třináctý J., Krizova L., Hadrová S., Hanuš O., Janštová B., Vorlová L., et al. (2006). Effect of rumen-protected protein supplemented with three amino acids on milk yield, composition and fatty acid profile in dairy cows. J. Anim. Feed Sci. 15, 3–15. doi: 10.22358/jafs/66834/2006
Vieira C., Diaz M. T., Martínez B., García-Cachán M. D. (2009). Effect of frozen storage conditions (temperature and length of storage) on microbiological and sensory quality of rustic crossbred beef at different states of ageing. Meat Sci. 83, 398–404. doi: 10.1016/j.meatsci.2009.06.013
Wanapat M., Cherdthong A., Phesatcha K., Kang S. (2015). Dietary sources and their effects on animal production and environmental sustainability. Anim. Nutr. 1 (3), 96–103. doi: 10.1016/j.aninu.2015.07.004
Weekes T. L., Luimes P. H., Cant J. P. (2006). Responses to amino acid imbalances and deficiencies in lactating dairy cows. J. Dairy Sci. 89 (6), 2177–2187. doi: 10.3168/jds.S0022-0302(06)72288-3
Keywords: rumen-protected amino acids, rumen-protected methionine, rumen-protected lysine, steer, beef cattle, meat quality
Citation: Cabezas A, De la Fuente J, Díaz MT, Bermejo-Poza R, del Olmo DM, Mateos J, Llanes N and Jimeno V (2023) Effect of the inclusion of rumen-protected amino acids in the diet of growing beef cattle on animal performance and meat quality. Front. Anim. Sci. 4:1269775. doi: 10.3389/fanim.2023.1269775
Received: 30 July 2023; Accepted: 11 October 2023;
Published: 27 October 2023.
Edited by:
Erick D. Batista, Universidade Federal de Lavras, BrazilReviewed by:
Jon Schoonmaker, Purdue University, United StatesPriscilla Dutra Teixeira, Universidade Federal de Lavras, Brazil
Copyright © 2023 Cabezas, De la Fuente, Díaz, Bermejo-Poza, del Olmo, Mateos, Llanes and Jimeno. This is an open-access article distributed under the terms of the Creative Commons Attribution License (CC BY). The use, distribution or reproduction in other forums is permitted, provided the original author(s) and the copyright owner(s) are credited and that the original publication in this journal is cited, in accordance with accepted academic practice. No use, distribution or reproduction is permitted which does not comply with these terms.
*Correspondence: Almudena Cabezas, YWxtdWNhYmVAdWNtLmVz