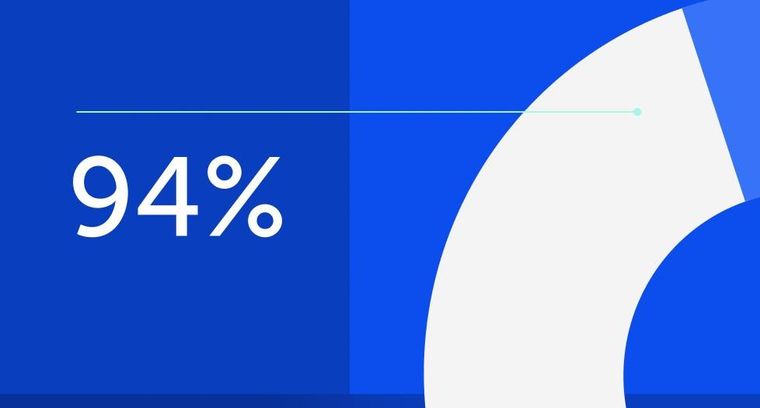
94% of researchers rate our articles as excellent or good
Learn more about the work of our research integrity team to safeguard the quality of each article we publish.
Find out more
REVIEW article
Front. Anim. Sci., 15 November 2022
Sec. Animal Nutrition
Volume 3 - 2022 | https://doi.org/10.3389/fanim.2022.962748
This article is part of the Research TopicNutrient Regulation of Feed Intake, Digestion, Metabolism, Behavior, and CognitionView all 10 articles
Livestock animals are often exposed to unavoidable stressful situations during their productive life that triggers stress-induced inflammatory responses, which are known to influence their nutrient requirements and feed intake. Decreased growth performance and immunocompetence of stressed livestock are often the main consequence of reduced feed intake. Because feed intake is usually reduced in animals experiencing stress conditions, concentrations of certain nutrients in the diets typically need to be increased to meet the requirements of the animals. Therefore, understanding the mechanisms that control feed intake in animals experiencing stress-induced inflammation is essential for increasing intake, milk or meat production, feed efficiency, and animal health. This review highlights the hormones regulating feed intake in ruminants and how stress-induced inflammation affect these hormones at local and systemic levels. The mechanism of feed intake regulation in ruminants is extremely complex and involves multiple controls. The liver is an important sensor of energy status in animals under homeostatic conditions, which transmits signals to brain feeding centers that modulate appetite. However, the physiologic consequences associated with different stressors will rearrange the hierarchy of mechanisms controlling feed intake compared to animals under homeostatic conditions, and other tissues (e.g., intestines), systems (e.g., endocrine and lymphatic) hormones (e.g., leptin and ghrelin) will directly affect intake regulation during stress and inflammatory conditions. It is suggested that the immune system can interact with the central nervous system to modulate feed intake. As example, stress events elicit numerous stressors that increase circulating proinflammatory cytokines, including tumor necrosis factor-alpha (TNF-α), interleukin-6 (IL-6), and IL-8, and acute-phase proteins (APP), and the magnitude of these responses are negatively correlated with feed intake. A direct effect of these cytokines on rumen microbial fermentation and intestinal barrier function was also reported and might indirectly affect intake regulation in ruminants. This review describes the main hormones and proinflammatory cytokines involved in stress-induced inflammation and how they can directly or indirectly affect intake regulation in ruminants. Understanding the mechanisms controlling feed intake in ruminants will help producers to implement management and feed strategies to optimize productivity and profitability in stressed livestock species.
Adequate feed intake will provide animals with essential nutrients and energy for maintenance, growth, reserves, and reproduction. Besides intake, the total amount of nutrients absorbed by the animals also depends on the ingredient or diet digestibility, which in turn, will be affected by feed intake and digestion kinetics (e.g., passage rate [solid and fluids] and digestion rate [soluble, potentially digestible, and indigestible fractions]). Several factors can influence feed intake, including dietary composition (e.g., energy density, nitrogen concentration, feed additives [ionophores]), physiological status (e.g., growth, pregnancy, and lactation [post-partum period]), sex, age, body composition (e.g., fatness), frame size (e.g., mature weight), health status (e.g., chronic, or acute diseases and metabolic disorders [rumen acidosis and bloat]), management (e.g., growth-promoting implants, grain/forage processing methods [physical such as particle size, and chemical such as digestibility characteristics], feed bunk space, frequency of feeding, and stocking density), and environmental factors (e.g., heat and cold stress, photoperiod, precipitation, mud, and wind). Throughout this review, some of these factors will be addressed in the context of cattle experiencing stress-induced inflammation. According to Ingvartsen and Andersen (2000), a better understanding of feed intake regulation and its integration with metabolism is the key to increasing intake, milk or meat production, feed efficiency, and health in livestock species.
The mechanism of feed intake regulation in ruminants is highly complex, involves multiple controls, and is not yet fully understood. Describing the mechanisms underlying intake control in ruminants is not the scope of this review and has been detailed by others (Anil and Forbes, 1988; Ingvartsen and Andersen, 2000; Allen et al., 2009; Allen and Piantoni, 2013; Allen, 2020). Briefly, physical and metabolic constraints are associated with feed intake control in beef and dairy cattle, but the hepatic oxidation theory (HOT) has frequently been suggested to be important in regulating feed intake in ruminants (Allen et al., 2009; Allen and Piantoni, 2013; Allen, 2020). According to the HOT, the liver is the primary sensor of energy (Allen, 2000), transmitting signals (inhibitory or stimulatory) to brain feeding centers (Anil and Forbes, 1988). Allen and Piantoni (2013) reported that there are three main mechanisms controlling feed intake in ruminants: 1) physical regulation (rumen fill), 2) endocrine regulation (gut peptides), and 3) metabolic regulation (oxidation of fuels). Signals from all these three mechanisms will reach the brain and provide the animal with information to initiate or end meals. According to Kuhla (2020), the hypothalamus is one of the key regions of the brain regulating intake. It can receive and integrate input signals from the periphery, detect humoral substances, such as nutrient-related metabolites, hormones and cytokines, and integrate neural signals from other brain regions to adjust feed intake. Ruminants are exposed to different stressors during their productive life (e.g., weather changes, weaning, commingling with different animals, road transportation, exposure to novel diets, feeding management, and environments), which has been shown to decrease feed intake and, consequently, cattle performance (Carroll and Forsberg, 2007; Cooke, 2017). Infectious diseases can also down-regulate appetite via cytokines in the immune system (Johnson, 1997), resulting in inadequate energy intake, reduced growth or lactation performance, and feed efficiency. According to Gautron and Laýe (2010), inflammation-associated anorexia reduces food intake during acute and chronic inflammatory states. Early evidence suggested that anorexia in sick mice is an adaptive-host defense response that increases survival (Murray and Murray, 1979). According to Johnson (1997), the immune system can interact with the central nervous system and modulate food intake. More specifically, cytokines such as interleukin-1b (IL-1b), interleukin-6 (IL-6), and tumor necrosis factor-α (TNF-α) may act as anorectic compounds (Johnson, 1997; Finck et al., 1998). Some studies also suggested that proinflammatory cytokines can also regulate feed intake by stimulating adipocytes to synthesize leptin (Grunfeld et al., 1996). Leptin has multiple physiological functions in the bovine (Chelikani et al., 2003), and has been implicated in the control of feed intake by regulating the synthesis and release of orexigenic (anabolic, e.g., neuropeptide Y) and anorexigenic (catabolic, e.g., corticotrophin-releasing hormone) neuropeptides in the hypothalamus (Houseknecht et al., 1998; Ingvartsen and Andersen, 2000).
The scope of this review is to address the feeding regulation mechanisms in cattle experiencing stress-induced inflammation, particularly how proinflammatory cytokines impact the hormones that regulate feed intake at local (e.g., physical regulation) or systemic levels (e.g., endocrine and metabolic regulation). This will be followed by a discussion of the effects of stress and inflammation on the rumen function and its interaction with feed intake regulation. Understanding the mechanisms controlling feed intake in ruminants will help producers implement management and feed strategies to optimize the productivity and profitability of cattle experiencing stress-induced inflammation.
Feed intake is controlled by a multifaceted neural mechanism with the hypothalamus as its center. According to Kuhla (2020), the hypothalamus is one of the key regions of the brain regulating energy balance (intake vs. expenditure) as it receives and integrates input signals from the periphery. Still according to these last authors, hypothalamic neurons may detect humoral substances, such as nutrient-related metabolites, hormones and cytokines, and integrate neural signals from other brain regions (e.g., tongue or orinasal origin) to adjust feed intake. The hypothalamus contains neurons that stimulate feed intake such as neuropeptide Y (NPY) and agouti-related peptide (AgRp), as well as neurons that suppress feed intake including those that produce proopiomelanocortin (POMC) and cocaine- and amphetamine-regulated transcript (CART; Harada and Inagaki, 2022). Accordingly, Miner (1992) reported that intracerebral ventricular injection of NPY increased both water and feed consumption in sheep, and Ingvartsen and Andersen (2000) reported that NPY is one of the most potent inducers of feed intake in dairy cows. Conversely, the administration of POMC inhibits feed intake in rats (Fan et al., 1997), whereas POMC mRNA expression is positively correlated with feed restriction in sheep (Lincoln and Richardson, 1998).
Feed intake regulation by the central nervous system is also affected by integrative signals produced peripherally, including adipose tissue reserves and products from ruminal fermentation and intestinal absorption (Roche et al., 2008; Harada and Inagaki, 2022). For instance, insulin and leptin regulate appetite by transmitting signals regarding the animal’s energy balance to the central nervous system (Schwartz et al., 2000; Air et al., 2002). Leptin binds to the leptin receptor on the arcuate nucleus of the hypothalamus, suppressing NPY and AgRp neurons. Leptin also suppresses neurons that produce orexin and melanin-concentrating hormone, which then triggers POMC and CART neurons (Harada and Inagaki, 2022). Insulin is a pancreatic hormone that enters the brain from circulation and binds to its receptors in the hypothalamus, and depresses feed intake by inhibiting NPY and AgRp neurons and activating POMC and CART neurons (Schwartz et al., 2000; Air et al., 2002; Harada and Inagaki, 2022). Air et al. (2002) reported that exogenous insulin suppresses feed intake and regulates energy balance even in doses that do not affect blood glucose concentrations. Several hormones produced by the intestine also act directly on the hypothalamus, controlling feed intake (Schwartz et al., 2000; Air et al., 2002; Harada and Inagaki, 2022). Glucagon-like peptide-1 (GLP-1), cholecystokinin (CCK), and peptide YY (PYY) have feed intake-suppressing effects, whereas ghrelin stimulates appetite (Woods, 2004; Chaudhri et al., 2008). Enteroendocrine cells produce and release these hormones in the gastrointestinal tract, stomach, and pancreas (Gribble and Reimann, 2019). Products of feed digestion (glucose, amino acids, and fatty acids) and ruminal microbial fermentation (short-chain fatty acids [SCFA]) stimulate the production of these intestinal hormones, which in turn target diverse tissues involved in the control of intestinal function, insulin secretion, and appetite behavior (Schwartz et al., 2000; Chaudhri et al., 2008; Gribble and Reimann, 2019; Harada and Inagaki, 2022). As an example, GLP-1 markedly reduces gastrointestinal emptying and suppresses feed intake (Harada and Inagaki, 2022), whereas the GLP-1 receptor is expressed on POMC/CART neurons in the hypothalamus and its activation controls appetite and eating behavior (Adriaenssens et al., 2019; Harada and Inagaki, 2022).
In ruminants, feed intake is controlled by a combination of physical (meal size and frequency) and metabolic mechanisms, with dietary characteristics playing an essential role in these two processes (Fitzsimons et al., 2017). The gastrointestinal tract and the attached glands are the first system that senses the dietary components (i.e., energy), secreting hormones that regulate feed intake (i.e., insulin and ghrelin) and nutrient utilization (i.e., glucose-dependent insulinotropic polypeptide, insulin, and IGF-1; Cantalapiedra-Hijar et al., 2018). Diet composition and energy concentration can change gut hormone concentrations and expression of neuropeptides important in regulating feed intake, including NPY and POMC (Relling and Reynolds, 2008; Relling et al., 2014; Perkins et al., 2014).
The animal responses to factors that potentially disrupt its homeostasis encompass a cascade of adaptive reactions initiated in the central nervous and peripheral tissues, leading to transient physiological, psychological, and behavioral changes that influence appetite, metabolism, and feeding behavior (Adam and Epel, 2007). Beef cattle are inevitably subjected to inherent management situations that elicit a stress-induced response resulting in suppressed feed intake, such as weaning (Haley et al., 2005), road transport (Marques et al., 2012; Filho et al., 2014), water and feed deprivation (Marques et al., 2019), and feedlot entry (Hutcheson and Cole, 1986; Loerch and Fluharty, 1999; Cooke, 2017). This stress-induced response does not involve a pathogen but it may increase the susceptibility of the animal to an existent pathogen(s), and triggers an inflammatory response cascade that negatively impacts their performance and immunocompetence (Cooke, 2017).
Although stress-induced response depends on the stressor’s intensity, duration, and nature (Adam and Epel, 2007), the activation of the HPA axis is the primary response to a stressful situation, leading to the synthesis and release of the corticotropin-releasing hormone (CRH) and vasopressin (VP) by their respective neurons located into the paraventricular nucleus of the hypothalamus (Adam and Epel, 2007; Carroll and Forsberg, 2007). In cattle, CRH stimulates the secretion of the adrenocorticotropic hormone (ACTH) from the pituitary gland. Circulating ACTH acts on the adrenal cortex stimulating the synthesis and release of cortisol, generally classified as a stress hormone (Carroll and Forsberg, 2007). Cortisol stimulates a range of responses in different tissues of the body, including glycogen, muscle, and adipose tissues breakdown to provide energy to the animal, hepatic production of acute-phase proteins (APP) as part of an inflammatory response, increased synthesis and release of catecholamines, and suppression of the inflammatory and immune systems to prevent autoimmune disorders (Carroll and Forsberg, 2007; Cooke, 2017). In contrast, elevated cortisol levels might increase food intake in humans (Adam and Epel, 2007). Under unstressed situations, insulin and cortisol have antagonistic effects on metabolism (Dallman et al., 1993), leading to a balanced system to provide energy to the organism (Adam and Epel, 2007). In a stressful environment that increases cortisol levels, the metabolism becomes unbalanced which transiently stimulates appetite (Tataranni et al., 1996; Adam and Epel, 2007). Tataranni et al. (1996) reported that the administration of glucocorticoids to healthy humans increased energy intake, which might be related to the ability of glucocorticoids to act directly or indirectly on the central regulation of appetite. Additionally, elevated glucocorticoid concentrations have been associated with insulin and leptin resistance (Björntorp, 2001; Rosmond, 2003). Therefore, the stress-induced response might result in lessened sensitization of satiety signals increasing appetite in humans (Adam and Epel, 2007). Nonetheless, a reduction in feed intake might also occur during acute and chronic inflammatory reactions in humans and livestock species (Gautron, 2009; Rodrigues et al., 2015; Lippolis et al., 2017). It is widely known that the administration of proinflammatory cytokines such as LPS, tumor necrosis factor-alpha (TNF-α), IL-6, and IL-8 reduces feed intake (Kapás and Krueger, 1992; Fantino and Wieteska, 1993; Sonti et al., 1996; Rodrigues et al., 2015; Lippolis et al., 2017). Upon a pathogenic stimulus, the innate immune system elicits several responses, including the synthesis of proinflammatory cytokines (i.e., TNF- α) from leukocytes, with the intent of attenuating or eliminating the infection (Carroll and Forsberg, 2007; Carroll et al., 2009; Rodrigues et al., 2015). Moreover, increased circulating proinflammatory cytokines elicits the hepatic synthesis of APP, including haptoglobin (Carroll and Forsberg, 2007; Carroll et al., 2009). All these inflammation responses have been associated with reduced feed intake in animals and humans (Kapás and Krueger, 1992; Fantino and Wieteska, 1993; Sonti et al., 1996; Rodrigues et al., 2015; Lippolis et al., 2017) and will be covered in the next section of this review. It is important to highlight that stress and inflammation are two separate physiological events that often, but not always, occur simultaneously, and the LPS-induced inflammation may not necessarily represent all forms of stress-induced inflammation.
According to Allen (2020) multiple signals are integrated in brain feeding center to ultimately determine feeding behavior. Fuels oxidized in the liver are derived from both the diet and tissues, and includes non-esterified fatty acids (NEFA), amino acids (AA), lactate, glycerol, and shot-chain fatty acids (mainly propionate; Allen, 2020). Vagal afferents will transmit signals (inhibitory and stimulatory) from the liver to brain feeding center (Langhans et al., 1996). According to Friedman (1997), firing rate of afferent fibers in the hepatic branch of the vagus will affect intake and a subpopulation of small vagal afferent neurons carries the hepatic signal to initiate eating behavior. The energy status of the liver is dependent upon the balance between energy-consuming and energy production reactions - fuels extracted from the blood by the liver can be converted to acetyl CoA (AcCoA) and oxidized in the tricarboxylic acid (TCA) cycle (Allen, 2020). However, according to Allen (2020) hormones and cytokines can modulate the hepatic oxidation by affecting availability of AcCoA for oxidation and/or by anaplerotic/cataplerotic reactions in TCA cycle. Leptin for example is known to act centrally to inhibit the effects of neuropeptide NPY (Houseknecht et al., 1998), apparently by inhibiting its synthesis in the arcuate nucleus of the hypothalamus (Cusin et al., 1996), causing a dose-dependent decrease in food intake (Pelleymounter et al., 1995). According to Houseknecht et al. (1998), there is mounting evidence that leptin may act peripherally as well, since leptin receptors are found outside the central nervous system (Tartaglia et al., 1995), for example on pancreatic β-cells (Kieffer et al., 1996), which can elicit its effects by directly inhibit β-cell secretion of insulin by altering ion channel function (Emilsson et al., 1997; Kieffer et al., 1996). Plata-Salaman et al. (1996) concluded that IL-1β can induce long-term anorexia when chronically administered intracerebroventricular. The infusion of 0.01 μg LPS per kilogram BW for approximately 6 h per day increased plasma IL-1β concentrations and decreased feed intake of mid-lactating Holstein cows (Ning et al., 2018). According to Plata-Salaman (1989) proinflammatory cytokines can regulate food intake by a direct action in the central nervous system through a specific neuro-immune interaction.
In summary, under stress-induced inflammation, it appears that the hierarchy of mechanisms controlling feed intake is rearranged and the direct effect of proinflammatory cytokines and hormones in the central nervous system will affect feed intake before the liver can detect the energy status and the HOT can take place to control feeding behavior.
Antigenic stimuli and/or inflammation can cause clinical and metabolic alterations in the host’s metabolism. Scientists have long recognized that different stressors and diseases during the productive life of ruminants can decrease intake and growth performance (Coates et al., 1963; Johnson, 1997; Carroll and Forsberg, 2007). Acute and chronic inflammatory reactions stimulate the synthesis and release of cytokines, including IL- 1b, IL-6, IL-8, and TNF-α, that elicit the hepatic synthesis of APP (Carroll and Forsberg, 2007; Carroll et al., 2009). These cytokines are known to result in anorexia (Johnson, 1997; Finck et al., 1998). Other studies suggest that these cytokines can regulate feed intake by stimulating adipocytes to synthesize leptin (Grunfeld et al., 1996). For instance, circulating TNF-α levels were previously reported as a feed intake reducer by modulating the central nervous and endocrine system and disrupting gastrointestinal function (Klasing and Korver, 1997; Lippolis et al., 2017). Gautron and Laýe (2010) reported that feed intake reduction during an acute and chronic inflammatory state results from the action of proinflammatory cytokines and prostaglandins E2 on the nervous system. The elevation of circulating cytokines also elicits an innate immune response by the hepatic tissue, releasing APP such as haptoglobin and ceruloplasmin (Carroll and Forsberg, 2007; Cooke, 2017). According to Carroll and Forsberg (2007), the innate immune system triggers the hepatic synthesis of APP in response to proinflammatory cytokines initiating an acute phase response within minutes after tissue damage (e.g., infection, disease, or trauma). Pathogen-associated molecular patterns also trigger additional sentinel cell responses and pain due to tissue damage, causing sensory nerves to release bioactive peptides (Tizard, 2018). In fact, Johnson (1997) reported that the immune system could interact with the central nervous system and modulate feed intake. Both proinflammatory cytokines and APP responses demand significant body resources, increase animal requirements, and decrease nutrient intake (Elsasser et al., 1997; Johnson, 1997). Accordingly, Araujo et al. (2010) observed a negative correlation (r ≤ -0.50) between circulating levels of APP and feed intake and weight gain in cattle, demonstrating that stress-induced inflammatory response is unfavorable to feed intake and animal performance.
Although the innate immune system responses are essential for restoring homeostasis following pathogenic infection, these reactions may be unnecessary when induced by stressors that are part of the management routine practices in beef cattle systems (Kushner, 1982; Cooke, 2017). These management practices, including weaning (Haley et al., 2005), transportation (Marques et al., 2012), and feedlot receiving (Loerch and Fluharty, 1999), cause inflammation and acute-phase reactions that frequently impair feed intake and health parameters, leading to a subsequent immunosuppressive state (Carroll and Forsberg, 2007). Additionally, feed deprivation stimulates the mobilization of body nutrients (Marques et al., 2019) and inflammatory stress response (Ward et al., 1992; Henricks et al., 1994), which also elicits acute-phase reactions in cattle (Cooke et al., 2011; Cooke and Arthington, 2012). Feed and water deprivation may also disturb the ruminal flora and cause microbial death (Meiske et al., 1958), resulting in the release of microbial endotoxins, which could activate the acute-phase response (Carroll et al., 2009). Collectively, these multiple inflammatory pathways are involved and likely impact the mechanisms associated with feed intake in cattle.
During an inflammatory response, leptin synthesis is increased to modulate the activation and maturation of leukocytes (Matarese et al., 2005; Fernández-Riejos et al., 2010; Rodrigues et al., 2015). Moreover, both leptin and CCK are known to synergistically limit gastrointestinal motility, resulting in satiety and reduced voluntary feed intake (Matson and Ritter, 1999). Rodrigues et al. (2015) observed that administration of lipopolysaccharide (LPS) to healthy steers increased insulin and leptin synthesis during an inflammatory reaction, with the intent of increasing energy utilization by the body to restore homeostasis (Waggoner et al., 2009). Accordingly, Adam and Epel (2007) suggest that the interactions between glucocorticoids, leptin, insulin, and NPY facilitate the storage, distribution, and release of energy during an acute activation of the hypothalamic-pituitary-adrenal (HPA) axis, which contributes to the initiation and termination of a meal.
To better understand the physiologic consequences of stressors and mechanisms controlling feed intake, Lippolis et al. (2017) evaluated the effects of intravenous infusion of LPS (0.5 μg/kg of body weight [BW]; Escherichia coli 0111:B4) on feed intake of beef steers. Steers receiving LPS consumed 50% less feed, expressed as kilograms per day or percent of BW, compared with the control group (i.v. injection of 0.9% sterile saline) on the day following the LPS challenge. However, intake was similar from days 2 to 6 following LPS injection. Waggoner et al. (2009) also conducted a study to evaluate the effects of supplemental metabolizable amino acids on beef steers exposed to an LPS challenge (Escherichia coli O55:B55). The intravenous infusion of LPS (1.5 µg of LPS/kg of BW) increased rectal temperature, serum cortisol, and haptoglobin concentration, resulting in a 6.5% reduction in feed intake compared with the control group. Several response mechanisms are involved in activating the immune system during routine management practices in beef cattle. These management practices may induce inflammatory and acute phase reactions that habitually impair cattle productivity and health, including feed intake. Hence, stress-induced inflammation impacts feed intake in beef cattle through several inflammatory agents; however, it seems like these are not the only mechanisms regulating feeding behavior, and additional research is warranted.
The physiologic consequences associated with different stressors will result in different mechanisms controlling feed intake than those observed in animals under homeostatic conditions. More specifically, the local control of feed intake in the gastrointestinal tract of ruminants can involve rumen motility and distension, passage rate, absorption of SCFA, and intestinal permeability dysfunction. Primary adaptive responses to stress or inflammation that alter the homeostasis of the gastrointestinal tract can lead to changes in one or more of these mechanisms that ultimately can affect intake regulation with a cause-and-effect nature.
According to Waggoner et al. (2009), the intravenous LPS infusion decreased by 25% and 24% the ruminal liquid and solid passage rates respectively, which is probably a result of reduced gut motility (Waggoner et al., 2009). Löest et al. (2018) conducted a similar experiment to evaluate the effects of supplementing branched-chain amino acids to beef steers exposed or not to a gram-negative bacterial LPS challenge (Escherichia coli O55:B55). Steers infused with LPS had 50% and 40% lower solid and liquid total tract passage rates, respectively, than the control group receiving saline. Lohuis et al. (1988) also reported that rumen-reticulum motility and abomasal emptying were impaired after administering intravenous LPS endotoxins. Still, according to Lohuis et al. (1988), the reduction of rumen motility starts between 30 and 60 min after endotoxins challenge, and both intensity and frequency of ruminal contractions were reduced or even ceased for up to 7 h after endotoxin administration. Rumen contractions are primarily controlled by the dorsal vagal nucleus in the brainstem. Foster (2017) reported that afferent fibers in the vagus nerve transmit regular and coordinated contractions, also known as primary contractions. Rumen distension resulting from recent meals usually increases the signals for primary contractions that contribute to an adequate mixing of the rumen content and removal of solid and liquid phases from the rumen (Constable et al., 1990; Foster, 2017). Nakade et al. (2007) concluded that restraint stress affects colonic motility via central CRH and peripheral 5-HT3 receptors located in the peripheral and central nervous systems of rats. Collectively, research suggests that reduction in feed intake by animals experiencing stress-inducing inflammation might also be due to impairment of rumen function and motility controlled by the central nervous system.
Löest et al. (2018) observed an interaction between LPS infusion and time (hours after LPS administration) for ruminal pH, in which steers infused with LPS had decreased pH at 8 hours after LPS infusion compared to the control group, but not at 0, 2, 4, 12, or 24 hours. Feed intake was reduced in steers infused with LPS compared to the control group in both Waggoner et al. (2009) and Löest et al. (2018), but no effects of LPS challenge were observed in the apparent total digestibility of dry matter or neutral detergent fiber in these experiments. Nonetheless, Lippolis et al. (2017) evaluated the effects of intravenous infusion of LPS on ruminal passage rate and forage degradability of beef steers. The LPS infusion reduced rumen liquid dilution rate and rumen liquid volume compared to the control group during the 24-h sampling period following treatment administration (Figure 1). Steers receiving LPS also had a reduced ruminal disappearance rate (Figure 1) compared with the control group. Contrary to Waggoner et al. (2009) and Löest et al. (2018), the LPS challenge decreased the effective degradability of dry matter and neutral detergent fiber compared with the control group in Lippolis et al. (2017). As expected, the LPS challenge increased plasma cortisol in all three studies, which modulates early physiological responses following a pathogenic stimulus to elicit proinflammatory and acute-phase reactions (Carroll et al., 2009; Cooke, 2017) and might have impaired rumen function and motility in cattle experiencing stress-induced reactions. In addition, Kent-Dennis et al. (2020) reported that when cultured ruminal epithelial cells are exposed to LPS stimulation, they increase the transcription of proinflammatory genes. The magnitude of this response is influenced by the dose and duration of LPS exposure. Moreover, the permeability of rumen tissues increases in the presence of LPS (Emmanuel et al., 2007), which might result in an increased transfer of microbial endotoxin into the circulation, eliciting an inflammation reaction (Carroll et al., 2009). Contrary, Gao et al. (2022), using an ex vivo method, concluded that LPS (at a pH of 7.4) does not affect the permeability of rumen tissues.
Figure 1 Effect of intravenous injection of lipopolysaccharide Escherichia coli 0111:B4 (LPS; 0.5 μg/kg of body weight) or saline solution (CON; 5-mL i.v. injection of 0.9% sterile saline) on rumen liquid dilution rate (%/hour; Panel A), rumen liquid volume (ml/kg of body weight; Panel B), and ruminal disappearance rate of dry matter and neutral detergent fiber (%/h; Panel C) of beefs steers. Adapted from Lippolis et al. (2017).
Stress conditions can also affect the rumen microbial community and, consequently, affect feed digestibility and feed intake in ruminants (Tajima et al., 2007). Changes in ruminal pH and ruminal microbiota composition of Holstein heifers in response to different housing temperatures were reported by Tajima et al. (2007). These authors evaluated the effects of three housing temperatures (20, 28, and 33°C) at 60% relative humidity on the rumen bacterial diversity of Holstein heifers. No effect of temperature was observed on dry matter intake, but ruminal pH decreased with the increase of housing temperature. The microbiota composition was significantly different at elevated temperatures. According to these authors, the ruminal microorganisms were responsive to the changes in the physiological parameters resulting from the heat stress in their host, but the factors that triggered these changes in ruminal diversity have yet to be elucidated. Freestone and Lyte (2010) reported that microorganisms in the gastrointestinal tract could interact with their host under specific stress conditions and have evolved particular systems for sensing hormones produced by the host, which is used as an environmental cue to respond to different conditions. Still, according to these authors, neuroendocrine hormones produced by the host, such as adrenaline and dopamine, can enhance the growth and virulence of some bacteria.
Jing et al. (2014) evaluated the effects of three doses (0.0, 0.4, and 0.8 mg/kg body weight) of LPS (Escherichia coli 0111:B4) on changes in ruminal microbiota and ruminal fermentation characteristics of dairy cows. After the LPS challenge, ruminal pH was linearly decreased, decreasing the organic matter degradability of alfalfa hay and soybean meal in the rumen. Also, the LPS infusion linearly increased the abundance of Firmicutes and linearly reduced the percentage of Bacteroidetes, Tenericutes, Spirochaetes, Chlorobi, and Lentisphaerae. Hence, changes in ruminal microbiota in this experiment can be an indirect result of LPS on ruminal pH, which might be related to reduced rumination time, as described by Borderas et al. (2008). A direct effect of cortisol on rumen microbial fermentation was reported by Samuelson et al. (2016). These authors used dual-flow continuous culture fermentors equipped to maintain pH between 5.0 and 8.0 to evaluate the effects of salivary cortisol on rumen microbial fermentation and nutrient digestibility in vitro. Donor cattle were fed a 41% (dry matter basis) cracked corn-based diet at 1.7% of BW for a minimum of 7 d before collecting rumen contents. According to these authors, rumen microbial fermentation and nutrient digestibility are altered, especially when exposed to 9 ng/mL salivary cortisol concentrations. Digestibility of NDF decreased in fermentors receiving cortisol at 9 ng/mL compared to 0, 3, and 6 ng/mL (quadratic effect; Figure 2). This decrease was 8.1% compared to the control group. Also, ruminal ammonia nitrogen concentration decreased after cortisol treatment in fermenters receiving 3, 6, or 9 ng/ml of cortisol compared to control (no cortisol infusion; Figure 2), or a 37% decrease compared to the control group.
Figure 2 Effects of cortisol concentration (0, 3, 6, and 9 ng/mL) on in vitro neutral detergent fiber (NDF) digestibility (quadratic effect; Panel A) and ruminal ammonia nitrogen (linear effect; Panel B). Adapted from Samuelson et al. (2016).
Many cytokines, such as TNF-α, IL-8, or IL-10, regulate the intestinal barrier function through the tight junction, and an increased or inappropriate cytokine profile will increase permeability (Wang et al., 2012; Guo et al., 2013). According to Salvo-Romero et al. (2015), the vagus nerve activity may also modulate intestinal barrier function via the release of neuropeptides such as acetylcholine and vasoactive intestinal peptide. In ruminants, some stressors that can cause intestinal barrier dysfunction include weaning, transportation stress, heat stress, feed deprivation, and acute or subacute ruminal acidosis (Baumgard and Rhoads, 2013; Kvidera et al., 2017). Although the mechanisms affecting intestinal permeability in ruminants are not yet completely elucidated, the negative effect of proinflammatory cytokines is probably the main factor involved in intestinal permeability. Therefore, induced inflammation might also affect gastrointestinal function and permeability, resulting in reduced feed intake in cattle and other livestock species.
Stress-induced inflammation will trigger adaptive physiological responses interfering with feed intake regulation in ruminants compared to those observed in animals under homeostatic conditions. Proinflammatory cytokines can decrease feed intake by stimulating different tissues to synthesize hormones that can stimulate or inhibit appetite. Although the liver is a key sensor of energy status, in ruminants, a direct effect of proinflammatory cytokines on rumen microbial fermentation and intestinal barrier function can indirectly affect intake regulation by their impact on the supply of energy to the host animals. Understanding the mechanisms controlling feed intake in ruminants will help producers in implementing management and feed strategies to optimize productivity and profitability in stress-induced animals. Future studies using more appropriate models, such as a direct effect of heat stress or road transportation, or direct infusion of stress hormones (e.g., vasopressin and corticotropin-releasing hormone) are necessary to better understand the cause-and-effect nature of stress-induced inflammation. Differences among animal category, physiological state, and dietary composition (e.g., receiving and finishing), duration/intensity of stressors, and the long-term effects of stressors on feed intake regulation should be investigated. More research is also needed to understand the hierarchical mechanisms that control feed intake in beef cattle experiencing stress-induced inflammation.
All authors listed have made a substantial, direct, and intellectual contribution to the work, and approved it for publication.
The authors declare that the research was conducted in the absence of any commercial or financial relationships that could be construed as a potential conflict of interest.
All claims expressed in this article are solely those of the authors and do not necessarily represent those of their affiliated organizations, or those of the publisher, the editors and the reviewers. Any product that may be evaluated in this article, or claim that may be made by its manufacturer, is not guaranteed or endorsed by the publisher.
Adam T. C., Epel E. S. (2007). Stress, eating and the reward system. Physiol. Behav. 91, 449–458. doi: 10.1016/j.physbeh.2007.04.011
Adriaenssens A. E., Biggs E. K., Darwish T., Tadross J., Sukthankar T., Girish M., et al. (2019). Glucose-dependent insulinotropic polypeptide receptor-expressing cells in the hypothalamus regulate food intake. Cell Metab. 30, 987–996.e6. doi: 10.1016/j.cmet.2019.07.013
Air E. L., Benoit S. C., Blake Smith K. A., Clegg D. J., Woods S. C. (2002). Acute third ventricular administration of insulin decreases food intake in two paradigms. Pharmacol. Biochem. Behav. 72, 423–429. doi: 10.1016/s0091-3057(01)00780-8
Allen M. S. (2000). Effects of diet on short-term regulation of feed intake by lactating dairy cattle. Journal of Dairy Science, 83 (7), 1598–1624. doi: 10.3168/jds.S0022-0302(00)75030-2
Allen M. S. (2020). Review: Control of feed intake by hepatic oxidation in ruminant animals: Integration of homeostasis and homeorhesis. Animal 14, S55–S64. doi: 10.1017/S1751731119003215
Allen M. S., Bradford B. J., Oba M. (2009). Board-invited review: The hepatic oxidation theory of the control of feed intake and its application to ruminants. J. Anim. Sci. 87, 3317–3334. doi: 10.2527/jas.2009-1779
Allen M. S., Piantoni P. (2013). Metabolic control of feed intake. implications for metabolic disease of fresh cows. Vet. Clin. N Am. 29, 279–297. doi: 10.1016/j.cvfa.2013.04.001
Anil M. H., Forbes J. M. (1988). The roles of hepatic nerves in the reduction of food intake as a consequence of intraportal sodium propionate administration in sheep. Q J. Exp. Physiol. 73, 539–546. doi: 10.1113/expphysiol.1988.sp003174
Araujo D. B., Cooke R. F., Hansen G. R., Staples C. R., Arthington J. D. (2010). Effects of rumen-protected polyunsaturated fatty acid supplementation on performance and physiological responses of growing cattle after transportation and feedlot entry. J. Anim. Sci. 88, 4120–4132. doi: 10.2527/jas.2009-2684
Baumgard L. H., Rhoads R. P. (2013). Effects of heat stress on postabsorptive metabolism and energetics. Annu. Rev. Anim. Biosci. 1, 311–337. doi: 10.1146/annurev-animal-031412-103644
Björntorp P. (2001). Do stress reactions cause abdominal obesity and comorbidities? Obes. Rev. 2, 73–86. doi: 10.1046/j.1467-789x.2001.00027.x
Borderas T. F., de Passillé A. M., Rushen J. (2008). Behavior of dairy calves after a low dose of bacterial endotoxin. J. Anim. Scie 86, 2920–2927. doi: 10.2527/jas.2008-0926
Cantalapiedra-Hijar G., Abo-Ismail M., Carstens G. E., Guan L. L., Hegarty R., Kenny D. A., et al. (2018). Review: Biological determinants of between-animal variation in feed efficiency of growing beef cattle. Animal 12, s321–s335. doi: 10.1017/S1751731118001489
Carroll J. A., Forsberg N. E. (2007). Influence of stress and nutrition on cattle immunity. Vet. Clin. N Am. 23, 105–149. doi: 10.1016/j.cvfa.2007.01.003
Carroll J. A., Reuter R. R., Chase C. C., Coleman S. W., Riley D. G., Spiers D. E., et al. (2009). Profile of the bovine acute-phase response following an intravenous bolus-dose lipopolysaccharide challenge. Innate Immun. 15, 81–89. doi: 10.1177/1753425908099170
Chaudhri O. B., Salem V., Murphy K. G., Bloom S. R. (2008). Gastrointestinal satiety signals. Annu. Rev. Physiol. 70, 239–255. doi: 10.1146/annurev.physiol.70.113006.100506
Chelikani P. K., Glimm D. R., Kennelly J. J. (2003). Short communication: Tissue distribution of leptin and leptin receptor mRNA in the bovine. J. Dairy Sci. 86, 2369–2372. doi: 10.3168/jds.S0022-0302(03)73830-2
Coates M. E., Fuller R., Harrison G. F., Lev M., Suffolk S. F. (1963). A comparison of the growth of chicks in the gustafsson germ-free apparatus and in a conventional environment, with and without dietary supplements of penicillin. B J. N 17, 141–150. doi: 10.1079/bjn19630015
Constable P. D., Gf H., Dm R. (1990). The reticulorumen: normal and abnormal motor function. II. secondary contraction cycles, rumination, and esophageal groove closure. Compend Contin Educ. Vet. 12, 169–174.
Cooke R. F. (2017). Invited paper: Nutritional and management considerations for beef cattle experiencing stress-induced inflammation. Prof Anim. Sci. 33, 1–11. doi: 10.15232/pas.2016-01573
Cooke R. F., Arthington J. D. (2012). Concentrations of haptoglobin in bovine plasma determined by ELISA or a colorimetric method based on peroxidase activity. J. Anim. Physiol. Anim. Nutr. 97, 531–536. doi: 10.1111/j.1439-0396.2012.01298.x
Cooke R. F., Bohnert D. W., Moriel P., Hess B. W., Mills R. R. (2011). Effects of polyunsaturated fatty acid supplementation on ruminal in situ forage degradability, performance, and physiological responses of feeder cattle. J. Anim. Sci. 89, 3677–3689. doi: 10.2527/jas.2010-3515
Cusin I., Rohner-Jeanrenaud F., Stricker-Krongrad A., Jeanrenaud B. (1996). The weight-reducing effect of an intracerebroventricular bolus injection of leptin in genetically obese fa/fa rats. reduced sensitivity compared with lean animals. Diabetes 45, 1446–1450. doi: 10.2337/diab.45.10.1446
Dallman M. F., Strack A. M., Akana S. F., Bradbury M. J., Hanson E. S., Scribner K. A., et al. (1993). Feast and famine: critical role of glucocorticoids with insulin in daily energy flow. Front. Neuroendocrinol 14, 303–347. doi: 10.1006/frne.1993.1010
Elsasser T. H., Kahl S., Steele N. C., Rumsey T. S. (1997). Nutritional modulation of somatotropic axis-cytokine relationships in cattle: A brief review. Comp. Biochem. Physiol. 116, 209–221. doi: 10.1016/S0300-9629(96)00279-4
Emilsson V., Liu Y. L., Cawthorne M. A., Morton N. M., Davenport M. (1997). Expression of the functional leptin receptor mRNA in pancreatic islets, and direct inhibitory action of leptin on insulin secretion. Diabetes 46, 313–316. doi: 10.2337/diab.46.2.313
Emmanuel D. G. V., Madsen K. L., Churchill T. A., Dunn S. M., Ametaj B. N. (2007). Acidosis and lipopolysaccharide from escherichia coli B:055 cause hyperpermeability of rumen and colon tissues. J. Dairy Sci. 90, 5552–5557. doi: 10.3168/jds.2007-0257
Fan W., Boston B. A., Kesterson R. A., Hruby V. J., Cone R. D. (1997). Role of melanocortinergic neurons in feeding and the agouti obesity syndrome. Nature 385, 165–168. doi: 10.1038/385165a0
Fantino M., Wieteska L. (1993). Evidence for a direct central anorectic effect of tumor-necrosis-factor-alpha in the rat. Physiol. Behav. 53, 477–483. doi: 10.1016/0031-9384(93)90141-2
Fernández-Riejos P., Najib S., Santos-Alvarez J., Martín-Romero C., Pérez-Pérez A., González-Yanes C., et al. (2010). Role of leptin in the activation of immune cells. Mediators Inflammation 2010, 568343. doi: 10.1155/2010/568343
Filho T. A. G., Cooke R. F., Cappellozza B. I., Reis M. M., Marques R. S., Bohnert D. W. (2014). Effects of meloxicam administration on physiological and performance responses of transported feeder cattle. J. Anim. Sci., 92, 4137–4144. doi: 10.2527/jas.2014-7783
Finck B. N., Kelley K. W., Dantzer R., Johnson R. W. (1998). In vivo and in vitro evidence for the involvement of tumor necrosis factor-in the induction of leptin by lipopolysaccharide. Endocrinology 139, 2278–2283. doi: 10.1210/endo.139.5.6012
Fitzsimons C., McGee M., Keogh K., Waters S. M., Kenny D. A. (2017). “Molecular physiology of feed efficiency in beef cattle,” in Biology of domestic animals. Eds. Scanes C. G., Hill R. A. (Boca Raton, FL: CRC Press), 120–163.
Foster D. (2017). Disorders of rumen distension and dysmotility. Vet. Clin. N Am. - Food Anim. Pract. 33, 499–512. doi: 10.1016/j.cvfa.2017.06.006
Freestone P., Lyte M. (2010). Stress and microbial endocrinology: Prospects for ruminant nutrition. Animal 4, 1248–1257. doi: 10.1017/S1751731110000674
Friedman M. I. (1997). An energy sensor for control of energy intake. Proc. Nutr. Soc. 56, 41–50. doi: 10.1079/pns19970008
Gao S., Zhula A., Liu W., Lu Z., Shen Z., Penner G. B., et al. (2022). Direct effect of lipopolysaccharide and histamine on the permeability of the rumen epithelium of steers ex vivo. J. Anim. Sci. 100, 1–9. doi: 10.1093/jas/skac005
Gautron L. (2009). Neurobiology of inflammation-associated anorexia. Front. Neurosci. 3. doi: 10.3389/neuro.23.003.2009
Gautron L., Laýe S. (2010). Neurobiology of inflammation-associated anorexia. Front. Neurosci. 3. doi: 10.3389/neuro.23.003.2009
Gribble F. M., Reimann F. (2019). Function and mechanisms of enteroendocrine cells and gut hormones in metabolism. Nat. Rev. Endocrinol. 15, 226–237. doi: 10.1038/s41574-019-0168-8
Grunfeld C., Zhao C., Fuller J., Pollock A., Moser A., Friedman J., et al. (1996). Endotoxin and cytokines induce expression of leptin, the ob gene product, in hamsters. J. Clin. Investig. 97, 2152–2157. doi: 10.1172/JCI118653
Guo S., Al-Sadi R., Said H. M., Ma T. Y. (2013). Lipopolysaccharide causes an increase in intestinal tight junction permeability in vitro and in vivo by inducing enterocyte membrane expression and localization of TLR-4 and CD14. Amer J. Path 182, 375–387. doi: 10.1016/J.AJPATH.2012.10.014
Haley D. B., Bailey D. W., Stookey J. M. (2005). The effects of weaning beef calves in two stages on their behavior and growth rate. J. Ani Sci. 83, 2205–2214. doi: 10.2527/2005.8392205x
Harada N., Inagaki N. (2022). Regulation of food intake by intestinal hormones in brain. J. Diabetes Investig. 13, 17–18. doi: 10.1111/jdi.13708
Henricks D. M., Jenkins T. C., Ward J. R., Krishnan C. S., Grimes L. (1994). Endocrine responses and body composition changes during feed restriction and realimentation in young bulls. J. Anim. Sci. 72, 2289–2297. doi: 10.2527/1994.7292289x
Houseknecht K. L., Baile C. A., Matteri R. L., Spurlock M. E. (1998). The biology of leptin: A review. J. Sci. 76, 1405–1420. doi: 10.2527/1998.7651405x
Hutcheson D. P., Cole N. A. (1986). Management of transit-stress syndrome in cattle: Nutritional and environmental effects. J. Anim. Sci. 62, 555–560. doi: 10.2527/jas1986.622555x
Ingvartsen K. L., Andersen J. B. (2000). Integration of metabolism and intake regulation: A review focusing on periparturient animals. J. Dairy Sci. 83, 1573–1597. doi: 10.3168/jds.S0022-0302(00)75029-6
Jing L., Zhang R., Liu Y., Zhu W., Mao S. (2014). Intravenous lipopolysaccharide challenge alters ruminal bacterial microbiota and disrupts ruminal metabolism in dairy cattle. B J. Nutr. 112, 170–182. doi: 10.1017/S000711451400066X
Johnson R. W. (1997). Inhibition of growth by pro-inflammatory cytokines: An integrated view. J. Sci. 75, 1244–1255. doi: 10.2527/1997.7551244x
Kapás L., Krueger J. M. (1992). Tumor necrosis factor-beta induces sleep, fever, and anorexia. Am. J. Physiol. 263, R703–R707. doi: 10.1152/ajpregu.1992.263.3.R703
Kent-Dennis C., Aschenbach J. R., Griebel P. J., Penner G. B. (2020). Effects of lipopolysaccharide exposure in primary bovine ruminal epithelial cells. J. Dairy Scie 103, 9587–9603. doi: 10.3168/jds.2020-18652
Kieffer T. J., Heller R. S., Habener J. F. (1996). Leptin receptors expressed on pancreatic β-cells. Biochem. Biophys. Res. Commun. 224, 522–527. doi: 10.1006/bbrc.1996.1059
Klasing K. C., Korver D. I. (1997). Leukocytic cytokines regulate growth rate and composition activation of the immune system. J. Anim. Sci. 75, 58–67. doi: 10.2527/animalsci1997.75Supplement_258x
Kuhla B. (2020). Review: Pro-inflammatory cytokines and hypothalamic inflammation: Implications for insufficient feed intake of transition dairy cows. Animal 14 (S1), S65–S77. doi: 10.1017/S1751731119003124
Kushner I. (1982). The phenomenon of the acute phase response. Ann. N Y Acad. Sci. 389, 39–48. doi: 10.1111/j.1749-6632.1982.tb22124.x
Kvidera S. K., Dickson M. J., Abuajamieh M., Snider D. B., Fernandez M. V. S., Johnson J. S., et al. (2017). Intentionally induced intestinal barrier dysfunction causes inflammation, affects metabolism, and reduces productivity in lactating Holstein cows. J. Dairy Sci. 100, 4113–4127. doi: 10.3168/jds.2016-12349
Langhans W. (1996). Role of the liver in the metabolic control of eating: what we know and what we do not know. Neurosci. Biobehav. Rev. 20 (1), 145–153. doi: 10.1016/0149-7634(95)00045-g
Lincoln G. A., Richardson M. (1998). Photo-neuroendocrine control of seasonal cycles in body weight, pelage growth and reproduction: lessons from the HPD sheep model. Comp. Biochem. Physiol. C Pharmacol. Toxicol. Endocrinol. 119, 283–294. doi: 10.1016/s0742-8413(98)00017-6
Lippolis K. D., Cooke R. F., Schubach K. M., Marques R. S., Bohnert D. W. (2017). Effects of intravenous lipopolysaccharide administration on feed intake, ruminal forage degradability, and liquid parameters and physiological responses in beef cattle. J. Anim. Sci. 95, 2859–2870. doi: 10.2527/jas2017.1502
Loerch S. C., Fluharty F. L. (1999). Physiological changes and digestive capabilities of newly received feedlot cattle. J. Anim. Sci. 77, 1113–1119. doi: 10.2527/1999.7751113x
Löest C. A., Gilliam G. G., Waggoner J. W., Turner J. L. (2018). Post-ruminal branched-chain amino acid supplementation and intravenous lipopolysaccharide infusion alter blood metabolites, rumen fermentation, and nitrogen balance of beef steers. J. Anim. Sci. 96, 2886–2906. doi: 10.1093/jas/sky168
Lohuis J. A., Verheijden J. H., Burvenich C., van Miert A. S. (1988). Pathophysiological effects of endotoxins in ruminants. Vet. Quart 10 (2), 109–116. doi: 10.1080/01652176.1988.9694157
Marques R. S., Bohnert D. W., de Sousa O. A., Brandão A. P., Schumaher T. F., Schubach K. M., et al. (2019). Impact of 24-h feed, water, or feed and water deprivation on feed intake, metabolic, and inflammatory responses in beef heifers. J. Anim. Sci. 97, 398–406. doi: 10.1093/jas/sky397
Marques R. S., Cooke R. F., Francisco C. L., Bohnert D. W. (2012). Effects of twenty-four hour transport or twenty-four hour feed and water deprivation on physiologic and performance responses of feeder cattle. J. Anim. Sci. 90, 5040–5046. doi: 10.2527/jas.2012-5425
Matarese G., Moschos S., Mantzoros C. S. (2005). Leptin in immunology. J. Immun. 174, 3137–3142. doi: 10.4049/jimmunol.174.6.3137
Matson C. A., Ritter R. C. (1999). Long-term CCK-leptin synergy suggests a role for CCK in the regulation of body weight. Am. J. Physiol. 276, R1038–R1045. doi: 10.1152/ajpregu.1999.276.4.R1038
Meiske J. C., Salsbury R. L., Hoefer J. A., Luecke R. W. (1958). The effect of starvation and subsequent re-feeding on some activities of rumen microorganisms in vitro. J. Anim. Sci. 17, 774–781. doi: 10.2527/jas2012-5425
Miner J. L. (1992). Recent advances in the central control of intake in ruminants. J. Sci. 70, 1283–1289. doi: 10.2527/1992.7041283x
Murray M. J., Murray A. B. (1979). Anorexia of infection as a mechanism of host defense. Am. J. Clin. Nutr. 32, 593–596. doi: 10.1093/ajcn/32.3.593
Nakade Y., Fukuda H., Iwa M., Tsukamoto K., Yanagi H., Yamamura T., et al. (2007). Restraint stress stimulates colonic motility via central corticotropin-releasing factor and peripheral 5-HT 3 receptors in conscious rats. Am. J. Physiol. Gastrointest Liver Physiol. 292, 1037–1044. doi: 10.1152/ajpgi.00419.2006
Ning L. T., Dong G. Z., Ao C., Zhang D. G., Erdene K., Zhang F. Q., et al. (2018). Effects of continuous low dose infusion of lipopolysaccharide on inflammatory responses, milk production and milk quality in dairy cows. J. Anim. Physiol. Anim. Nutr. 102, e262–e269. doi: 10.1111/jpn.12737
Pelleymounter M. A., Cullen M. J., Baker M. B., Hecht R., Winters D., Boone T., et al. (1995). Effects of the obese gene product on body weight regulation in ob/ob mice. Science 269, 540–543. doi: 10.1126/science.762477
Perkins S. D., Key C. N., Garrett C. F., Foradori C. D., Bratcher C. L., Kriese-Anderson L. A., et al. (2014). Residual feed intake studies in Angus-sired cattle reveal a potential role for hypothalamic gene expression in regulating feed efficiency. J. Ani Sci. 92, 549–560. doi: 10.2527/jas.2013-7019
Plata-Salaman C. R. (1989). Review: Immunomodulators and feeding regulation: A humoral link between the immune and nervous systems. Brain Behav. Immun. 3, 193–213. doi: 10.1016/0889-1591(89)90036-6
Plata-salaman C. R., Sonti G., Borkoski J. P., Wilson C. D., Ffrench-Mullen J. M. (1996). Anorexia induced by chronic central administration of cytokines at estimated pathophysiological concentrations. Physiol. Behav. 60, 867–875. doi: 10.1016/0031-9384(96)00148-5
Relling A. E., Crompton L. A., Loerch S. C., Reynolds C. K. (2014). Short communication: plasma concentration of glucose-dependent insulinotropic polypeptide may regulate milk energy production in lactating dairy cows. J. Dairy Sci. 97, 2440–2443. doi: 10.3168/jds.2013-7574
Relling A. E., Reynolds C. K. (2008). Abomasal infusion of casein, starch and soybean oil differentially affect plasma concentrations of gut peptides and feed intake in lactating dairy cows. Domest. Anim. Endocrinol. 35, 35–45. doi: 10.1016/j.domaniend.2008.01.005
Roche J. R., Blache D., Kay J. K., Miller D. R., Sheahan A. J., Miller D. W. (2008). Neuroendocrine and physiological regulation of intake with particular reference to domesticated ruminant animals. Nutr. Res. Rev. 21, 207–234. doi: 10.1017/S0954422408138744
Rodrigues M. C., Cooke R. F., Marques R. S., Arispe S. A., Keisler D. H., Bohnert D. W. (2015). Effects of oral meloxicam administration to beef cattle receiving lipopolysaccharide administration or vaccination against respiratory pathogens1. J. Anim. Sci. 93, 5018–5027. doi: 10.2527/jas.2015-9424
Rosmond R. (2003). Stress induced disturbances of the HPA axis: a pathway to type 2 diabetes? Med. Sci. Monit 9, RA35–RA39.
Salvo-Romero E., Alonso-Cotoner C., Pardo-Camacho C., Casado-Bedmar M., Vicario M. (2015). The intestinal barrier function and its involvement in digestive disease. Rev. Esp Enferm Dig 107, 686–696. doi: 10.17235/reed.2015.3846/2015
Samuelson K. L., Salazar A. L., Rath L. L., Alford J. B., Oosthuysen E. R., Ivey S. L., et al. (2016). 1670 WS salivary cortisol concentrations affect rumen microbial fermentation and nutrient digestibility in vitro. J. Anim. Sci. 94, 813–814. doi: 10.2527/jam2016-1670
Schwartz M. W., Woods S. C., Porte D., Seeley R. J., Baskin. D. G. (2000). Central nervous system control of food intake. Nature 404, 661–671. doi: 10.1038/35007534
Sonti G., Ilyin S. E., Plata-Salaman C. R. (1996). Anorexia induced by cytokine interactions at pathophysiological concentrations. Am. J. Physiol. 270, R1394–R1402. doi: 10.1152/ajpregu.1996.270.6.R1394
Tajima K., Nonaka I., Higuchi K., Takusari N., Kurihara M., Takenaka A., et al. (2007). Influence of high temperature and humidity on rumen bacterial diversity in Holstein heifers. Anaerobe 13, 57–64. doi: 10.1016/j.anaerobe.2006.12.001
Tartaglia L. A., Dembski M., Weng X., Deng N., Culpepper J., Devos R., et al. (1995). Identification, and expression cloning of a leptin receptor, OB-r. Cell 83, 1263–1271. doi: 10.1016/0092-8674(95)90151-5
Tataranni P. A., Larson D. E., Snitker S., Young J. B., Flatt J. P., Ravussin E. (1996). Effects of glucocorticoids on energy metabolism and food intake in humans. Am. J. Physiol. 271, E317–E325. doi: 10.1152/ajpendo.1996.271.2.E317
Waggoner J. W., Löest C. A., Turner J. L., Mathis C. P., Hallford D. M. (2009). Effects of dietary protein and bacterial lipopolysaccharide infusion on nitrogen metabolism and hormonal responses of growing beef steers1. J. Anim. Sci. 87, 3656–3668. doi: 10.2527/jas.2009-2011
Wang Y., Liu Y., Sidhu A., Ma Z., McClain C., Feng W., et al. (2012). Lactobacillus rhamnosus GG culture supernatant ameliorates acute alcohol-induced intestinal permeability and liver injury. Am. J. Physiol. Gas-trointest Liver Physiol. 303, 32–41. doi: 10.1152/ajpgi.00024.2012.-Endotoxemia
Ward J. R., Henricks D. M., Jenkins T. C., Bridges W. C. (1992). Serum hormone and metabolite concentrations in fasted young bulls and steers. Domest Anim. Endocrinol. 9, 97–103. doi: 10.1016/0739-7240(92)90023-Q
Keywords: feedlot cattle, leptin, liver, microbial fermentation, pro-inflammatory cytokines, ruminant
Citation: Gouvêa VN, Cooke RF and Marques RS (2022) Impacts of stress-induced inflammation on feed intake of beef cattle. Front. Anim. Sci. 3:962748. doi: 10.3389/fanim.2022.962748
Received: 06 June 2022; Accepted: 31 October 2022;
Published: 15 November 2022.
Edited by:
Kendall C Swanson, North Dakota State University, United StatesReviewed by:
Amanda K Lindholm-Perry, Agricultural Research Service (USDA), United StatesCopyright © 2022 Gouvêa, Cooke and Marques. This is an open-access article distributed under the terms of the Creative Commons Attribution License (CC BY). The use, distribution or reproduction in other forums is permitted, provided the original author(s) and the copyright owner(s) are credited and that the original publication in this journal is cited, in accordance with accepted academic practice. No use, distribution or reproduction is permitted which does not comply with these terms.
*Correspondence: Reinaldo F. Cooke, cmVpbmFsZG9jb29rZUB0YW11LmVkdQ==
Disclaimer: All claims expressed in this article are solely those of the authors and do not necessarily represent those of their affiliated organizations, or those of the publisher, the editors and the reviewers. Any product that may be evaluated in this article or claim that may be made by its manufacturer is not guaranteed or endorsed by the publisher.
Research integrity at Frontiers
Learn more about the work of our research integrity team to safeguard the quality of each article we publish.