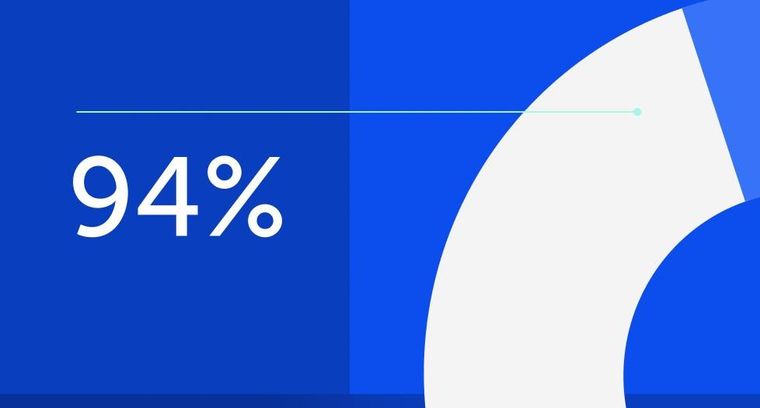
94% of researchers rate our articles as excellent or good
Learn more about the work of our research integrity team to safeguard the quality of each article we publish.
Find out more
ORIGINAL RESEARCH article
Front. Anim. Sci., 10 June 2022
Sec. Animal Physiology and Management
Volume 3 - 2022 | https://doi.org/10.3389/fanim.2022.919416
This article is part of the Research TopicAnimal Responses to Climatic Stress: Strategies for Coping with Harsh Climatic ConditionsView all 6 articles
The risk of climate change is increasing year by year and changing environmental temperatures will increasingly have effects on productivity in the poultry industry. Thermal conditioning is a method of improving thermotolerance and productivity in chickens (Gallus gallus domesticus) that experience high ambient temperatures. Thermal conditioning involves exposure of chickens to high temperatures at an early age. This conditioning treatment can affect tolerance to other type of stress. However, the effect of thermal conditioning on tolerance of low temperatures has not been investigated. Therefore, in this study we investigated the effect of thermal conditioning in chickens on thermoregulation during exposure to low temperatures. Three day-old female broiler chicks were exposed to high ambient temperatures (40°C for 12 h) as a thermal conditioning treatment. A control group of chicks was kept at 30°C. At 7 days-old, both groups of chicks were exposed to low temperatures (16 ± 0.5°C) for 3 h. Thermal conditioning treatment reduced the decrease in rectal temperature during cold exposure that occurred in control chicks. In addition, hypothalamic mRNA expression of brain derived neurotrophic factor, thyrotropin-releasing hormone and arginine vasotocin genes was higher in thermal conditioning treated chicks than control chicks. The mRNA expression of avian uncoupling protein in the liver was also higher in thermal conditioning chicks. These results suggest that thermal conditioning treatment can improve thermoregulatory mechanisms of chicks under low temperature environments.
The external environment, especially environmental temperature, has a great impact on the health and productivity of livestock animals. Currently, global warming is progressing due to the emission of warming gases associated with the use of fossil fuels. The increase in heat stress for livestock caused by global warming has led to a decrease in productivity and an increase in health risk (Lu et al., 2007; Kilic and Simsek, 2013; Lara and Rostagno, 2013; Zaboli et al., 2018; Vandana et al., 2021). On the other hand, global warming brings not only increasing summer temperatures but also climate change such as heavy precipitation, high temperature extremes (Fischer and Knutti, 2015). Due to this climate change, sudden low temperatures in winter, that is, the invasion of cold waves, is also a problem. For example, the major cold wave that hit East Asia and North America during the 2017-2018 winter season has been attributed to the meandering of the jet stream due to the reduction of sea ice in the Alaskan Sea caused by global warming (Tachibana et al., 2019). Environmental temperatures below the lower limit of the thermoneutral zone have a significant impact on livestock productivity. The effect of low environmental temperatures is generally referred to as cold stress. In poultry, cold stress can cause various effects such as increased feed intake, decreased digestibility, and decreased egg production (Arad and Marder, 1982; Sahin and Sahin, 2001; Blahova et al., 2007). And more, in broiler chicken (Gallus gallus domesticus), it is reported that low environment temperature increases the risk of ascites (Shlosberg et al., 1992: Yahav et al., 1997; Ipek and Sahan, 2006). As mentioned above, chickens are constantly at high risk of being stressed by environmental temperatures. Therefore, there is an urgent need to improve their ability to adapt to environmental temperature, that is, their ability to thermoregulate.
Thermal conditioning treatment in which young chicks are exposed to high environmental temperatures is one of the methods for improvement of thermoregulation in chickens under high ambient temperature environment, namely it improves heat tolerance in chickens (Arjona et al., 1988; Yahav and Hurwitz, 1996; Yahav and McMurtry, 2001; Tanizawa et al., 2014; Ouchi et al., 2021). The improvement of thermoregulation by thermal conditioning treatment is due to improvement of physiological and behavioral responses under high temperature environment. It is speculated that the thermal conditioning treatment changes the response to environmental temperature under heat environment by causing a modification of central thermoregulatory mechanisms (Tanizawa et al., 2014; Ouchi et al., 2020). Although the effects of thermal conditioning treatment on thermoregulation at high temperatures have been studied, there are no reports on the effects of the thermal conditioning treatment on thermoregulation at low temperatures.
Adult chickens have a wide temperature range of 13 to 28°C as a thermoneutral zone. However, young chicks are extremely sensitive to environmental temperature, and the thermoneutral zone is around 30°C until 2 weeks of age (Meltzer, 1983). Therefore, young chicks are vulnerable to cold stress. Various adaptive reactions occur in chickens under cold environments. For example, there is shivering to produce heat, or the release of thyroid hormone into the blood and increases the production of heat in peripheral organs.
The aim of this study was to examine the effect of thermal conditioning treatment on thermoregulatory responses during low temperature exposure in chicks. Thus, we exposed chicks to cold temperatures after thermal conditioning treatment. In addition, the body temperature of chicks during cold exposure, the expression of thermoregulatory genes in the hypothalamus and liver, and blood biochemical parameters were investigated in this study.
The handling of birds was performed in accordance with the regulations of the Animal Experiment Committee of Hiroshima University (authorization No. C19-15) and complied with Law No. 105 and Notification No. 6 of the Japanese government.
Newly hatched female chicks (Chunky) were obtained from a local farm (Fukuda Hatchery, Okayama, Japan). The chicks were maintained in a room at 30 ± 0.2°C under continuous light. Birds were housed in polypropylene boxes (36 × 40 × 30 cm) with sawdust litter at a density of 6 chicks per box during the experiment period. A commercial starter diet (Nichiwa Sangyo Co. Ltd., Kobe, Japan) and water were supplied ad libitum until the end of experiment.
The experiment design of this study is shown in Figure 1. A total of 32 chicks were used in Experiment 1 and 2. At 3 days of age, the chicks were divided into two groups (n = 8 per group), with the mean body weight of each group as similar as possible (92 ± 5.3g and 93 ± 5.1g). Chicks in a thermal conditioning treatment group were exposed to high temperature environment (40 ± 0.5°C) as described elsewhere (Ouchi et al., 2021). A commercially available heat chamber (Type P-008B, Showa Furanki, Saitama, Japan), which can maintain the chamber at any desired temperature by means of a thermostat was used for thermal conditioning treatment. The chicks of thermal conditioning treatment group were promptly placed in the heat chamber pre-set at 40°C. They were exposed to treatment for 12 hours after entering the chamber. During thermal conditioning treatment they had free access to feed and water. Chicks in the thermal conditioning treatment group experienced one thermal conditioning treatment at 3 days of age and were reared in thermoneutral zone as in the control group until low temperature exposure. The chicks of control group were kept at thermoneutral zone until low temperature exposure.
Figure 1 Experiment design for this study. A thermal conditioning group (TC) was exposed to 40°C for 12 hours at 3 days of age. At 7 days of age, both TC and control groups (C) were exposed to a low temperature environment (16°C) for 180 minutes in experiment and for 30 minutes in experiment 2. Control chicks were kept in their thermoneutral zone until the low temperature exposure.
At 7 days of age, both control and thermal conditioning group of chicks were exposed to low ambient temperature (16 ± 0.5°C) for 180 min. The 7-day-old period was selected because a previous report indicated that the thermal conditioning treatment suppressed the increase in rectal temperature due to heat exposure at the 7-day-old period (Ouchi et al., 2021). Low temperature exposure was performed using a climate control room, which was pre-regulated to 16°C. The chicks of both groups were promptly moved from the thermos neutral zone to a prepared climate control room for low temperature exposure. Rectal temperature was measured every 30 min during low temperature exposure. The number of chicks was 8 per group.
At 7 days of age, chicks in control and thermal conditioning groups were exposed to a cold environment (16 ± 0.5°C) for 30 min. Chicks were handled as in Experiment 1. The number of chicks was 8 per group. Immediately after cold exposure, chicks were anesthetized by isoflurane (FUJIFILM Wako Pure Chemical Corporation, Osaka, Japan) and decapitated. Blood, diencephalic tissues and hepatic tissues were harvested. From the time of anesthesia to the completion of blood and all tissue collection and freezing took less than 3 minutes. The blood was centrifuged, and then plasma was stored at -20°C until analysis. The diencephalic samples were collected by referring to the atlas (Kuenzel and Masson, 1988). The diencephalic and hepatic tissue were stored at -80°C until RNA isolation.
The concentration of plasma glucose, free fatty acids (FFA) and corticosterone (CORT) were measured. Plasma glucose and FFA concentrations were measured using a commercially available assay kit and according to the instructions (Glucose CII-Test Wako and NEFA C-Test Wako, Wako Pure Chemical Industries Ltd., Osaka, Japan). Plasma CORT levels were measured by enzyme immunoassay, as used in previous studies (Tanizawa et al., 2014; Ouchi et al., 2021). For both assays, optical density was measured using an Ultramark Microplate Reader (BIO-RAD Laboratories, Tokyo, Japan).
Trizol reagent (Invitrogen, CA, USA) was used for isolation of total RNA from the collected tissues. The isolation analysis was performed according to the manufacturer’s instructions. The total RNA concentration was measured by the spectrophotometer (NanoDrop-ONE, Thermo Scientific, Inc) at 260 nm. Thereafter, the RNA samples were treated with DNase I using a DNA-free kit (Ambion, Austin, USA). cDNAs were synthesized from DNase treated total RNA samples using a PrimeScript RT reagent kit (TaKaRa Bio Inc., Shiga, Japan). Gene expression was measured using a light cycler system (Light cycler Nano: Roche Applied Science, IN, USA). Target genes in diencephalic tissue were brain derived neurotrophic factor (BDNF), thyrotropin-releasing hormone (TRH), neuropeptide Y (NPY), corticotropin-releasing hormone (CRH), arginine vasotocin (AVT), pro-opiomelanocortin (POMC). Target genes in hepatic tissue were avian uncoupling protein (av-UCP), carnitine palmitoyltransferase 1 (CPT1), fatty acid synthase (FAS), acetyl-CoA carboxylase (ACC). The sequences of primers are shown in Table 1. The cycling conditions of PCR was: initialization at 95°C for 5 minutes, amplification of 45 cycles with 95°C for 10 seconds and 60°C for 20 seconds. The total volume of PCR was 20 μl, containing 2 μl of cDNA, 1.5 μl of each 0.2 μM primers, 10 μl of 2 × FastStart Essential DNA Green Master (Roche Life Science, Basel, Switzerland) and 5 μl of PCR grade water. Ribosomal protein S17 (RPS17) was used for geometrical mean of internal control after confirming that there was no significant difference in ct values between the control and thermal conditioning groups, and 2-△△Ct methods were used for normalization of data. The ct values of RPS 17 in diencephalon are control= 20.41 ± 0.351 and TC= 20.24 ± 0.378. And those in liver are control= 22.31 ± 0.441 and TC= 22.84 ± 0.521. These date are presented as mean ± SEM. Since both RPS17 ct values showed a normal distribution, a t-test was performed (in diencephalon: P= 0.662, in liver: P= 0.475).
StatView (Version 5, SAS Institute, Cary, USA, 1998) was used for stastical analysis. Rectal temperature data f were evaluated by two-way repeated ANOVA. Other data were evaluated by t-test after confirming that they were normally distributed to determine the effect of thermal conditioning treatment. P < 0.05 was used to indicate significant differences. All data were expressed as means ± standard error of the mean (SEM).
Figure 2 shows the changes in rectal temperature in thermal conditioning treated and control chicks during cold exposure. There was a significant effect of thermal conditioning on rectal temperature (P = 0.012), with rectal temperatues of thermal conditioning treated chicks higher than those of control chicks. In addition, there was a significant (P < 0.001) effect of time. Rectal temperatures of both groups of chicks declined 30 min after cold exposure, then increased from 60 min onwards after cold exposure. The interaction between effect of time and thermal conditioning was not observed (P = 0.855).
Figure 2 Changes in rectal temperature during and after low temperature exposure in experiment 1. The number of chicks in each group was n = 8. C, control; TC, thermal conditioning.
The plasma glucose concentration of thermal conditioning treated group tended to be lower than that of control (P = 0.061). There were no significant differences between control and thermal conditioning chicks in plasma FFA (P = 0.428) and corticosterone (P = 0.517) concentrations (Figure 3). FFA concentrations in both groups were around 200 nEq/dL, and corticosterone concentrations were around 4 ng/mL.
Figure 3 Plasma metabolite concentrations after low temperature exposure in experiment 2 (mean ± SEM). (A) shows plasma glucose concentrations, (B) shows plasma free fatty acid (FFA) concentrations and (C) shows plasma corticosterone concentrations. The number of chicks in each group was n = 8. C, control; TC, thermal conditioning. † means a differential trend between the two groups.
Figure 4 shows the expression of thermoregulation and stress related genes in diencephalic tissue. Expression of BDNF and TRH genes was higher in thermal conditioning treated chicks than in control chicks (Figures 4A, B; P = 0.011, P = 0.020). In the gene expression of AVT, the level of thermal conditioning treated chicks was tendency higher than that of control (Figure 4E; P = 0.086). However, there was no significant difference between thermal conditioning treated chicks and control chicks on the gene expression of NPY, CRH and POMC (Figures 4C–E; P = 0.192, P = 0.314, P = 0.356).
Figure 4 Gene expression levels in hypothalamic tissue after exposure to low temperature in experiment 2 (mean ± SEM). (A) shows gene expression of brain-derived neurotrophic factor (BDNF), (B) shows gene expression of thyrotropin-releasing hormone (TRH), (C) shows gene expression of neuropeptide Y (NPY), (D) shows gene expression of corticotropin-releasing hormone (CRH), (E) shows gene expression of arginine vasotocin (AVT), and (F) shows gene expression of proopiomelanocortin (POMC). The number of chicks in each group was n = 8.* Indicates a significant difference between groups.
Figure 5 shows gene expression in liver tissue after cold exposure. The expression level of av-UCP was higher in thermal conditioning treated chicks than control chicks (Figure 5A; P = 0.048). There were no differences between control and thermal conditioning treated chicks in the expression levels of CPT-1, FAS and ACC (Figures 5B–D; P = 0.2674, P = 0.550 and P = 0.450).
Figure 5 Gene expression levels in liver tissue after low temperature exposure in experiment 2 (mean ± SEM). (A) shows gene expression of uncoupling protein (UCP), (B) shows gene expression of carnitine palmitoytransferase 1 (CPT-1), (C) shows gene expression of fatty acid synthase (FAS), (D) shows gene expression of acetyl-CoA carboxylase (ACC). The number of chicks in each group was n = 8. * indicates a significant difference between groups.
Thermal conditioning treatment of chicks is known to alter thermoregulatory mechanisms of chickens under high ambient temperatures (Yahav and McMurtry, 2001; Ouchi et al., 2021). This study shows that thermal conditioning at early age affects the decrease in rectal temperature due to exposure to low temperature environment. In addition, it was found that thermal conditioning affects the expression of several important genes related to thermoregulation in the diencephalon and liver after 30 minutes of low temperature exposure.
Thermal conditioning significantly reduced the magnitude of the decline of rectal temparture during and after cold exposure, suggesting a recovery of rectal temperature after the 30 minute cold esposure was similar in control and conditioned chicks. In a previous study, it was reported that thermal conditioning treatment supressed the rise in body temperature during heat exposure at y days of age, especially the rise in rectal temperature 30 min after exposure (Ouchi et al., 2021). Thus, thermal conditioning at an early age may improve responsiveness to both high and low environmental temperatures.
Homeothermic animals can maximize activities necessary for life support by keeping their body temperature constant. They can respond and make adjustments when ambiet temperatures are outside the thermoneutral zone. These adaptive responses include behavioral adaptation and physiological adaptation (Etches et al., 2008; Yahav, 2014). Physiological adaptation is to maintain body temperature homeostasis by actions of autonomic nervous system and the endocrine system. The hypothalamic-pituitary-thyroid (HPT) axis is involved in thermoregulation through the actions of thyroid hormones (Mullur et al., 2014). TRH is secreted from the hypothalamic neurons and acts on thyrotropin-producing cells in the anterior pituitary gland to control the synthesis and secretion of thyroid stimulating hormone (TSH), thus playing an important role as the starting point of the HPT axis. It has been reported that a lot of TRH neurons and perikaryal are present in hypothalamus of chickens (Geris et al., 1999; De Groef et al., 2005). In the present study, TRH gene expression was higher in thermal conditioning treated chicks than in control chicks. Therefore, it is suggested that HPT axis in thermal conditioning treated chicks was more active than that in untreated chicks during low temperature exposure. The HPT axis is one of the mechanisms that controls heat production in peripheral tissues. This is because thyroid hormones released from the thyroid gland by activation of the HPT axis stimulate heat production and metabolism by peripheral tissues such as liver and muscle (Williamson and Davison, 1985; Lam et al., 1986). UCP is one of the proteins activated in peripheral tissues by thyroid hormone. UCP plays a role in thermogenesis by uncoupling mitchondria (Cannon and Nedergaard, 1985; Himms-Hagen and Harper, 2001). Av-UCP is present in chickens and has high homology with mammalian UCP3 (Raimbault et al., 2001; Toyomizu et al., 2002). Moreover, it is reported that cold stimulation increases the expression of av-UCP in chickens (Toyomizu et al., 2002; Dridi et al., 2008). In this study, hepatic av-UCP gene expression after cold exposure in thermal conditioning treated chicks was higher than that of control chicks. Thus, in thermal conditioning treated chicks, the decrease in body temperature caused by exposure to low temperatures may have been activated av-UCP, together with activation of the HPT axis generating heat compared to the control chicks.
There was an indication that AVT gene expression may have been higher in thermal conditioning treated chicks than control chciks. Administration of AVT induced hypothermia, decreased heart rate, respiratory rate and oxygen consumption, but did not induce quivering behavior in birds (John and George, 1992). Additionally, AVT can increase plasma thyroxine (T4) and decrease triiodothyronine (T3) levels (John and George, 1992; Ruuskanen et al., 2021). Thyroid hormones, including T3 and T4, play important roles in avian heat production and thermoregulation (Klandorf et al., 1981; Decuypere et al., 2005), and plasma AVT concentrations have been negatively correlated with body surface temperature (Giloh et al., 2012). Therefore, AVT may have a relationship with thermogenesis in birds. However, it is not clear if gene expression of AVT during cold exposure was increased by thermal conditioning treatment. AVT is associated with heat production as well as water balance in the body. It has been reported that plasma AVT concentrations were increased due to water deficiency in the body (Koike et al., 1977; Arad et al., 1985; Saito and Grossmann, 1998). It is known that water in the body plays an important role in body heat production and body temperature maintenance. Although water is closely related to body temperature, it is not known if thermal conditioning treatment affects body water. This needs to be considered in future experiments.
There was no difference between thermal conditioning treated chicks and control chicks in CRH and POMC gene expression and no difference in plasma corticosterone levels after low temperature exposure. The genes mentioned above are closely associated with stress responses (Herman and Cullinan, 1997; Bonfiglio et al., 2011; Herman et al., 2016) and stress responses involve the hypothalamo-pituitary-adrenal (HPA) axis. Thermal conditioning treatment had no effect on the HPA axis after low temperature exposure. The HPA axis can be activated within several minutes after a stimulus is detected by an animal as a stressor (Cockrem, 2007). While activation of the HPA axis can raise blood glucose levels (Munck et al., 1984; Wingfield et al., 1998; Valeria et al., 2012), blood glucose levels in chicks after cold exposure were not affected by thermal conditioning treatment. However, there could have been effects of the treatment before the first sampling point 30 min after the beginning of the cold exposure, so further investigation will be worthwhile in the future.
It has been reported that BDNF plays a role in feeding regulation and energy metabolism in animals (Nakagawa et al., 2000; Xu et al., 2003). Gene expression of BDNF was higher in thermal conditioning treated chicks than control chicks in the current study. It is probable that the treated group required more energy because it maintained a higher body temperature than the control group. Increased BDNF expression in the diencephalon may have been associated with increased energy metabolism. It was reported that thermal conditioning treatment at early age induced DNA methylation and demethylation at hypothalamus in chicks (Yossifoff et al., 2008; Kisliouk and Meiri, 2009; Ouchi et al., 2021). DNA methylation is one of the epigenetic modifications that regulate gene expression without changing the gene sequence (Kass et al., 1997; Crider et al., 2012). It has been reported that thermal conditioning treatment alters DNA methylation levels in the BDNF promoter region (Yossifoff et al., 2008; Ouchi et al., 2021). It can be suggested that the increase in BDNF gene expression in thermal conditioning treated group was due to DNA methylation reorganization in the central BDNF gene promoter region due to thermal conditioning treatment.
Prior to this study, there were many reports that thermal conditioning treatment affets physiological and behavioral responses in high temperature environment (Yahav and Hurwitz, 1996; Yahav and McMurtry, 2001; Ouchi et al., 2021). In this study, as a new finding, it was shown that thermal conditioning treatment affects the physiological responses in a low temperature environment. Such a phenomenon is recognized as cross-resistance, that is, the acquisition of tolerance to other stresses by stress treatment (Li and Gong, 2011). Poultry are exposed to many kinds of environmental stressors (Janczak et al., 2007; Zhang et al., 2011; Tsiouris et al., 2018). Although it may be possible to acquire tolerance to various environmental stressors by thermal conditioning treatment, further research is needed to investigate the mechanism for acquiring cross tolerance. And more, it is reported that thermal conditioning treatment at 3 days of age improve thermotorelance in 42-day-old broiler chickens (Yahav and McMurtry, 2001). Therefore, it is likely that the effects of thermal conditioning treatment in ealry life period on physiological responses to the low temperature environment will be confirmed in older chickens that had a long period of time since the thermal conditioning treatment. However, futher pursuit is needed because the thermoregulation of chickens changes as they grow.
The original contributions presented in the study are included in the article/supplementary material. Further inquiries can be directed to the corresponding author.
The animal study was reviewed and approved by Animal Experiment Committee of Hiroshima University (authorization No. C19-15).
YO collected the data used in this study, analyzed the data, and drafted the manuscript. TB designed the study and analyzed the partial data. JC and VC contributed to the development and writing. YO and TB interpreted the data, and reviewed and improved the manuscript. All authors contributed to the article and approved the submitted version.
This work was supported by a Grants-in Aid for Scientific Research from JSPS (JP17KT0077 and 21H02344 to TB).
The authors declare that the research was conducted in the absence of any commercial or financial relationships that could be construed as a potential conflict of interest.
All claims expressed in this article are solely those of the authors and do not necessarily represent those of their affiliated organizations, or those of the publisher, the editors and the reviewers. Any product that may be evaluated in this article, or claim that may be made by its manufacturer, is not guaranteed or endorsed by the publisher.
The authors acknowledge the staff of the Laboratory of Animal Behavior and Physiology, Hiroshima University for their technical support in maintaining the animals.
Arad Z., Arnason S. S., Chadwick A., Skadhauge E. (1985). Osmotic and Hormonal Responses to Heat and Dehydration in the Fowl. J. Comp. Physiol. B. 155, 227–234. doi: 10.1007/BF00685217
Arad Z., Marder J. (1982). Comparison of the Productive Performances of the Sinai Bedouin Fowl, the White Leghorn and Their Crossbreds: Study Under Natural Desert Conditions. Br. Poult. Sci. 23, 333–338. doi: 10.1080/00071688208447966
Arjona A. A., Denbow D. M., Weaver W. D. (1988). Effect of Heat Stress Early in Life on Mortality of Broilers Exposed to High Environmental Temperatures Just Prior to Marketing. Poult. Sci. 67, 226–231. doi: 10.3382/ps.0670226
Blahova J., Dobsikova R., Strakova E., Suchy P. (2007). Effect of Low Environmental Temperature on Performance and Blood System in Broiler Chickens (Gallus Domesticus). Acta Vet. Brno. 76, S17–S23. doi: 10.2754/avb200776S8S017
Bonfiglio J. J., Inda C., Refojo D., Holsboer F., Arzt E., Silberstein S. (2011). The Corticotropin-Releasing Hormone Network and the Hypothalamic-Pituitary-Adrenal Axis: Molecular and Cellular Mechanisms Involved. Neuroendocrinology 94, 12–20. doi: 10.1159/000328226
Cannon B., Nedergaard J. (1985). The Biochemistry of an Inefficient Tissue: Brown Adipose Tissue. Essays Biochem. 20, 110–164.
Cockrem J. F. (2007). Stress, Corticosterone Responses and Avian Personalities. J. Ornithol. 148, 169–178. doi: 10.1007/s10336-007-0175-8
Crider K. S., Yang T. P., Berry R. J., Bailey L. B. (2012). Folate and DNA Methylation: A Review of Molecular Mechanisms and the Evidence for Folate’s Role. Adv. Nutr. 3, 21–38. doi: 10.3945/an.111.000992
Decuypere E., Van As P., van der Geyten S., Darras V. M. (2005). Thyroid Hormone Availability and Activity in Avian Species: A Review. Domest. Anim. Endocrinol. 29, 63–77. doi: 10.1016/j.domaniend.2005.02.028
De Groef B., Vandenborne K., Van As P., Darras V. M., Kühn E. R., Decuypere E., et al. (2005). Hypothalamic Control of the Thyroidal Axis in the Chicken: Over the Boundaries of the Classical Hormonal Axes. Domest. Anim. Endocrinol. 29, 104–110. doi: 10.1016/j.domaniend.2005.02.008
Dridi S., Temim S., Derouet M., Tesseraud S., Taouis M. (2008). Acute Cold- and Chronic Heat-Exposure Upregulate Hepatic Leptin and Muscle Uncoupling Protein (UCP) Gene Expression in Broiler Chickens. J. Exp. Zool. Part A Ecol. Genet. Physiol. 309, 381–388. doi: 10.1002/jez.461
Etches R. J., John T. M., Verrinder Gibbins A. M. (2008). “Behavioral, Physiological, Neuroendocrine and Molecular Responses to Heat Stress,” in Poultry Production in Hot Climates, 2nd edition. Ed. Daghir N. J. (Wallingford UK: CAB International), 48–79.
Fischer E., Knutti R. (2015). Anthropogenic Contribution to Global Occurrence to Heavy-Precipitation and High-Temperature Extremes. Nat. Clim Change. 5, 560–564. doi: 10.1038/nclimate2617
Geris K. L., D’Hondt E., Kühn E. R., Darras V. M. (1999). Thyrotropin-Releasing Hormone Concentrations in Different Regions of the Chicken Brain and Pituitary: An Ontogenetic Study. Brain Res. 818, 260–266. doi: 10.1016/S0006-8993(98)01281-5
Giloh M., Shinder D., Yahav S. (2012). Skin Surface Temperature of Broiler Chickens is Correlated to Body Core Temperature and is Indicative of Their Thermoregulatory Status. Poult. Sci. 91, 175–188. doi: 10.3382/ps.2011-01497
Herman J., Cullinan W. (1997). Neurocircuitry of Stress: Central Control of the Hypothalamo-Pituitary-Adrenal Axis. Trends Neurosci. 20, 78–84. doi: 10.1016/S0166-2236(96)10069-2
Herman J. P., McKlveen J. M., Ghosal S., Kopp B., Wulsin A., Makinson R., et al. (2016). Regulation of the Hypothalamic-Pituitary-Adrenocortical Stress Response. Compr. Physiol. 6, 603–621. doi: 10.1002/cphy.c150015
Himms-Hagen J., Harper M. E. (2001). Physiological Role of UCP3 may be Export of Fatty Acids From Mitochondria When Fatty Acid Oxidation Predominates: An Hypothesis. Proc. Soc Exp. Biol. Med. 226, 78–84. doi: 10.1177/153537020122600204
Ipek A., Sahan U. (2006). Effects of Cold Stress on Broiler Performance and Ascites Susceptibility. Asian-Aust. J. Anim. Sci. 19, 734–738. doi: 10.5713/ajas.2006.734
Janczak A. M., Torjesen P., Palme R., Bakken M. (2007). Effects of Stress in Hens on the Behaviour of Their Offspring. Appl. Anim. Behav. Sci. 107, 16. doi: 10.1016/j.applanim.2006.09.016
John T. M., George J. C. (1992). Effects of Arginine Vasotocin on Cardiorespiratory and Thermoregulatory Responses in the Pigeon. Comp. Biochem. Physiol. Part C Comp. 102, 353–359. doi: 10.1016/0742-8413(92)90126-R
Kass S. U., Pruss D., Wolffe A. P. (1997). How Does DNA Methylation Repress Transcription? Trends Genet. 13, 444–449. doi: 10.1016/s0168-9525(97)01268-7
Kilic I., Simsek E. (2013). The Effects of Heat Stress on Egg Production and Quality of Laying Hens. J. Anim. Vet. Adv. 12, 42–47. doi: 10.36478/javaa.2013.42.47
Kisliouk T., Meiri N. (2009). Critical Role for Dynamic Changes in Histone H3 Methylation at the Bdnf Promoter During Postnatal Thermotolerance Acquisition. Eur. J. Neurol. 30, 1909–1922. doi: 10.1111/j.1460-9568.2009.06957.x
Klandorf H., Sharp P. J., Macleod M. G. (1981). The Relationship Between Heat Production and Concentrations of Plasma Thyroid Hormones in the Domestic Hen. Gen. Comp. Endocrinol. 45, 513–520. doi: 10.1016/0016-6480(81)90056-3
Koike T. I., Pryor L. R., Neldon H. L., Venable R. S. (1977). Effect of Water Deprivation of Plasma Radioimmunoassayable Arginine Vasotocin in Conscious Chickens (Gallus Domesticus). Gen. Comp. Endocrinol. 33, 359–364. doi: 10.1016/0016-6480(77)90051-X
Kuenzel W. J., Masson M. A. (1988). Stereotaxic Atlas of the Brain of the Chick (Gallus domesticus). Baltimore, MD, USA: The Johns Hopkins University Press.
Lam S. K., Harvey S., Hall T. R. (1986). In Vitro Release of Triiodothyronine and Thyroxine From Thyroid Glands of the Domestic Fowl (Gallus Domesticus). Gen. Comp. Endocrinol. 63, 178–185. doi: 10.1016/0016-6480(86)90154-1
Lara L. J., Rostagno M. H. (2013). Impact of Heat Stress on Poultry Production. Animals 3, 356–369. doi: 10.3390/ani3020356
Li Z. G., Gong M. (2011). Mechanical Stimulation-Induced Cross-Adaptation in Plants: An Overview. J. Plant Biol. 54, 358–364. doi: 10.1007/s12374-011-9178-3
Lu Q., Wen J., Zhang H. (2007). Effect of Chronic Heat Exposure on Fat Deposition and Meat Quality in Two Genetic Types of Chicken. Poult. Sci. 86, 1059–1064. doi: 10.1093/ps/86.6.1059
Meltzer A. (1983). Thermoneutral Zone and Resting Metabolic Rate of Broilers. Br. Poult. Sci. 24, 471–476. doi: 10.1080/00071668308416763
Mullur R., Liu Y. Y., Brent G. A. (2014). Thyroid Hormone Regulation of Metabolism. Physiol. Rev. 94, 355–382. doi: 10.1152/physrev.00030.2013
Munck A., Guyre P. M., Holbrook N. J. (1984). Physiological Functions of Glucocorticoids in Stress and Their Relation to Pharmacological Actions. Endocr. Rev. 5, 25–44. doi: 10.1210/edrv-5-1-25
Nakagawa T., Tsuchida A., Itakura Y., Nonomura T., Ono M., Hirota F., et al. (2000). Brain-Derived Neurotrophic Factor Regulates Glucose Metabolism by Modulating Energy Balance in Diabetic Mice. Diabetes 49, 436–444. doi: 10.2337/diabetes.49.3.436
Ouchi Y., Chowdhury V. S., Cockrem J. F., Bungo T. (2021). Effects of Thermal Conditioning on Changes in Hepatic and Muscular Tissue Associated With Reduced Heat Production and Body Temperature in Young Chickens. Front. Vet. Sci. 187. doi: 10.3389/fvets.2020.610319
Ouchi Y., Tanizawa H., Shiraishi J. I., Cockrem J. F., Chowdhury V. S., Bungo T. (2020). Repeated Thermal Conditioning During the Neonatal Period Affects Behavioral and Physiological Responses to Acute Heat Stress in Chicks. J. Therm. Biol. 94, 102759. doi: 10.1016/j.jtherbio.2020.102759
Raimbault S., Dridi S., Denjean F., Lachuer J., Couplan E., Bouillaud F., et al. (2001). An Uncoupling Protein Homologue Putatively Involved in Facultative Muscle Thermogenesis in Birds. Biochem. J. 353, 441–444. doi: 10.1042/bj3530441
Ruuskanen S., Hsu B. Y., Nord A. (2021). Endocrinology of Thermoregulation in Birds in a Changing Climate. Mol. Cell. Endocrinol. 519, 111088. doi: 10.1016/j.mce.2020.111088
Sahin N., Sahin K. (2001). Optimal Dietary Concentrations of Vitamin C and Chromium Picolinate for Alleviating the Effect of Low Ambient Temperature (6.2 Degrees C) on Egg Production, Some Egg Characteristics, and Nutrient Digestibility in Laying Hens. Vet. Med. 46, 229–236. doi: 10.17221/7887-VETMED
Saito N., Grossmann R. (1998). Effects of Short-Term Dehydration on Plasma Osmolality, Levels of Arginine Vasotocin and its Hypothalamic Gene Expression in the Laying Hen. Comp. Biochem. Physiol. - A Mol. Integr. Physiol. 121, 235–239. doi: 10.1016/S1095-6433(98)10123-X
Shlosberg A., Pano G., Handji V., Berman E. (1992). Prophylactic and Therapeutic Treatment of Ascites in Broiler Chickens. Br. Poult. Sci. 33, 141–148. doi: 10.1080/00071669208417451
Tachibana Y., Komatsu K. K., Alexeev V. A., Cai L., Ando Y. (2019). Warm Hole in Pacific Arctic Sea Ice Cover Forced Mid-Latitude Northern Hemisphere Cooling During Winter 2017-18. Sci. Rep. 9, 5567. doi: 10.1038/s41598-019-41682-4
Tanizawa H., Shiraishi J. I., Kawakami S. I., Tsudzuki M., Bungo T. (2014). Effect of Short-Term Thermal Conditioning on Physiological and Behavioral Responses to Subsequent Acute Heat Exposure in Chicks. J. Poult. Sci. 51, 80–86. doi: 10.2141/jpsa.0130040
Toyomizu M., Ueda M., Sato S., Seki Y., Sato K., Akiba Y. (2002). Cold-Induced Mitochondrial Uncoupling and Expression of Chicken UCP and ANT mRNA in Chicken Skeletal Muscle. FEBS Lett. 529, 313–318. doi: 10.1016/S0014-5793(02)03395-1
Tsuouris V., Georgopoulou I., Batzios C., Pappaioannou N., Ducatelle R., Fortomaris P. (2018). Heat Stress as a Predisposing Factor for Necrotic Enteritis in Broiler Chicks. Avian Pathol. 47, 616–624. doi: 10.1080/03079457.2018.1524574.
Valeria M., Jane R., Pawel H., Karen A. S. (2012). Pre- and Post-Natal Stress in Context: Effects on the Stress Physiology in a Precocial Bird. J. Exp. Biol. 215, 3955–3964. doi: 10.1242/jeb.071423
Vandana G. D., Sejian V., Lees A. M., Pragna P., Silpa M. V., Maloney S. K. (2021). Heat Stress and Poultry Production: Impact and Amelioration. Int. J. Biometeorol. 65, 163–179. doi: 10.1007/s00484-020-02023-7
Williamson R. A., Davison T. F. (1985). The Effect of a Single Injection of Thyrotrophin on Serum Concentrations of Thyroxine, Triiodothyronine, and Reverse Triiodothyronine in the Immature Chicken (Gallus Domesticus). Gen. Comp. Endocrinol. 58, 109–113. doi: 10.1016/0016-6480(85)90142-X
Wingfield J. ,. C., Maney D. L., Breuner C. W., Jacobs J. D., Lynn S., Ramenofsky M., et al. (1998). Ecological Bases of Hormone—Behavior Interactions: The “Emergency Life History Stage”. Am. Zool. 38, 191–206. doi: 10.1093/icb/38.1.191
Xu B., Goulding E. H., Zang K., Cepoi D., Cone D., Jones K. R., et al. (2003). Brain-Derived Neurotrophic Factor Regulates Energy Balance Downstream of Melanocortin-4 Receptor. Nat. Neurosci. 6, 736–742. doi: 10.1038/nn1073
Yahav S. (2014). “Regulation of Body Temperature – Strategies and Mechanisms,” in Sturkie’s Avian Physiology, 6th Edition. Ed. Scanes C. (London UK: Elsevier Publications), 869–905.
Yahav S., Hurwitz S. (1996). Induction of Thermotolerance in Male Broiler Chickens by Temperature Conditioning at an Early Age. Poult. Sci. 75, 402–406. doi: 10.3382/ps.0750402
Yahav S., McMurtry J. P. (2001). Thermotolerance Acquisition in Broiler Chickens by Temperature Conditioning Early in Life - The Effect of Timing and Ambient Temperature. Poult. Sci. 80, 1662–1666. doi: 10.1093/ps/80.12.1662
Yahav S., Straschnow A., Plavnik I., Hurwitz S. (1997). Blood System Response of Chickens to Changes in Environmental Temperature. Poult. Sci. 76, 627–633. doi: 10.1093/ps/76.4.627
Yossifoff M., Kisliouk T., Meiri N. (2008). Dynamic Changes in DNA Methylation During Thermal Control Establishment Affect CREB Binding to the Brain-Derived Neurotrophic Factor Promoter. Eur. J. Neurol. 28, 2267–2277. doi: 10.1111/j.1460-9568.2008.06532.x
Zaboli G., Huang X., Feng X., Ahn D. U. (2018). How can Heat Stress Affect Chicken Meat Quality? – a Review. Poult. Sci. 98, 1551–1556. doi: 10.3382/ps/pey399
Keywords: thermal conditioning, low temperature exposure, tolerance, thermoregulation, poultry
Citation: Ouchi Y, Chowdhury VS, Cockrem JF and Bungo T (2022) Thermal Conditioning Can Improve Thermoregulation of Young Chicks During Exposure to Low Temperatures. Front. Anim. Sci. 3:919416. doi: 10.3389/fanim.2022.919416
Received: 13 April 2022; Accepted: 17 May 2022;
Published: 10 June 2022.
Edited by:
Aristide Maggiolino, University of Bari Aldo Moro, ItalyReviewed by:
Vincenzo Tufarelli, University of Bari Aldo Moro, ItalyCopyright © 2022 Ouchi, Chowdhury, Cockrem and Bungo. This is an open-access article distributed under the terms of the Creative Commons Attribution License (CC BY). The use, distribution or reproduction in other forums is permitted, provided the original author(s) and the copyright owner(s) are credited and that the original publication in this journal is cited, in accordance with accepted academic practice. No use, distribution or reproduction is permitted which does not comply with these terms.
*Correspondence: Takashi Bungo, dC1idW5nb0BvdXMuYWMuanA=
Disclaimer: All claims expressed in this article are solely those of the authors and do not necessarily represent those of their affiliated organizations, or those of the publisher, the editors and the reviewers. Any product that may be evaluated in this article or claim that may be made by its manufacturer is not guaranteed or endorsed by the publisher.
Research integrity at Frontiers
Learn more about the work of our research integrity team to safeguard the quality of each article we publish.