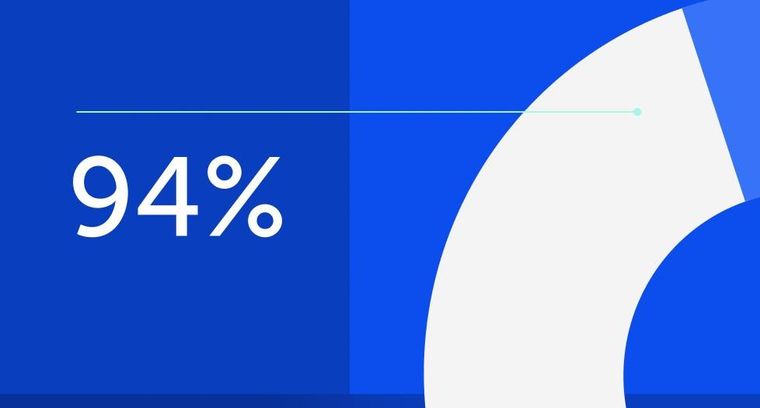
94% of researchers rate our articles as excellent or good
Learn more about the work of our research integrity team to safeguard the quality of each article we publish.
Find out more
ORIGINAL RESEARCH article
Front. Anim. Sci., 16 February 2022
Sec. Animal Physiology and Management
Volume 3 - 2022 | https://doi.org/10.3389/fanim.2022.807217
This article is part of the Research TopicEmbryonic Development, Pregnancy Establishment and LossView all 9 articles
This study compared the gene expression of extraembryonic membranes (EEM) from in vitro produced (IVP) and in vivo (AI) derived pregnancies. A piece of conceptus (day 18) or chorioallantois (day 32) was used for DNA and RNA isolation and sex determination. Male and female ratios were analyzed by Chi-square. A total of three samples per sex and group (AI and IVP, days 18 and 32) were used for transcriptome analysis. Differentially expressed genes (DEGs) were determined using edgeR-robust. A false discovery rate (FDR) <0.05 was used for statistical significance. Sex ratio was similar on day 18 for AI and IVP groups. On day 32, the IVP group had a greater number of females than males (75 vs. 25%, P = 0.004). When comparing AI and IVP males vs. females, in both groups, genes upregulated in females on day 18 were related to placental function such as PAGs and TKDPs. On males on day 18, IFNT-related genes were upregulated. Comparing the techniques within sex, on day 18 female conceptuses, 50 genes were upregulated in IVP, and 21 in AI. IGF2, which is involved in placenta development, and APOA2, APOB, and APOE, involved in lipid metabolism, were upregulated in IVP conceptuses. On day 18, males had 15 upregulated genes in AI and 7 in IVP. On day 32, females had 21 upregulated genes in AI and 53 in IVP. Genes involved in lipid synthesis and metabolism were increased in the IVP group. Males on day 32 presented 899 DEGs, 564 upregulated in AI and 335 in IVP. Embryos from IVP had decreased expression of genes related to lipid and carbohydrate metabolism. Interestingly, pregnancy-associated glycoproteins (PAG) 7, 9, 10, and 19, were downregulated in IVP male. In conclusion, IVP-derived male embryos were more susceptible to alterations in gene expression and these effects extend to the peri-implantation period including genes associated with placental development and markers of placental function.
It is generally accepted that embryos produced in vitro (IVP) differ in quality than in vivo-derived (IVD) embryos in terms of ultrastructure (Rizos et al., 2002a), gene expression profiles (Lonergan et al., 2003a,b; Gad et al., 2012), and cryotolerance (Rizos et al., 2002c). However, differences between IVP and IVD embryos have also been found past the blastocyst stage. When comparing the viability of IVP and IVD conceptuses on day 16 of pregnancy, more degenerated conceptuses (25 [7/28] vs. 5% [2/34]) and smaller embryonic discs were found in the IVP group compared to their IVD counterparts (Bertolini et al., 2002b). Furthermore, they seem to induce different endometrial gene expression during the peri-implantation period (15–20 days after fertilization), altering expression of genes associated with immune response and metabolism (Bauersachs et al., 2009; Mansouri-Attia et al., 2009; Sandra et al., 2011; Mathew et al., 2019).
Around day 18, genes involved in placental developmental such as the trophoblast Kunitz domain protein family (TKDPs) have been reported to have decreased expression in IVP conceptuses indicating potential differences in placental development between AI and IVP pregnancies (Hue et al., 2012; Betsha et al., 2013; Barnwell et al., 2016). Likewise, a contemporary comparison between embryo transfer (ET) of IVP embryos and artificial insemination (AI) reported pregnancies of 34% for ET vs. 50.3% for AI, and greater embryonic loss from days 30 to 60 for ET than AI [15.9 vs. 5.2% (Wiltbank et al., 2018)]. The increased number of losses of IVP pregnancies between days 30 and 90 have been attributed to some extent to reduced development of placental membranes and placental blood vessels (Hill et al., 2000), in part due to abnormal epigenetic reprogramming caused by the IVP systems (Bertolini et al., 2002a,b).
The development of the placenta is a critical process to pregnancy success, however, monitor their function or status can be challenging. In cattle, the pregnancy associated glycoproteins (PAGs), are products of trophoblast cells of the placenta, and can be measured in maternal blood (Wallace et al., 2015). In the bovine, the phylogenetically more ancient bovine PAGs, are usually found to be expressed mainly by mononucleated trophoblast cells although some are present in both mononucleated and binucleated cells (Green and Hennessy, 2018). The largest subgrouping of bovine PAGs has more recent origins and these are generally found to be restricted in their localization to binucleated cells and are considered modern PAGs (Green et al., 2000; Green and Hennessy, 2018). Due to their similarity with each other, up to date, they do not have specific antibodies to be individually detected in maternal circulation by common hormonal assays (Green et al., 2005; Wooding et al., 2005; Wallace et al., 2019), so their individual pattern of release is still unknown. Pohler et al. (2016) suggested that PAGs when measured as a group, might be present in distinct concentrations in pregnancies from in vitro vs. in vivo embryos. The circulating concentration of PAGs have been associated with pregnancy establishment and placentation and used as a pregnancy diagnostic tool (Green et al., 2000, 2005; Pohler et al., 2016). However, it is not known how much the individual PAGs expression varies between IVP and IVD embryos, as well as between males and females placentas.
Indeed, the IVP system seems to affect male and female embryos differently. For example, glucose supplementation in culture medium increases the male: female ratio at the blastocyst stage (Gutiérrez-Adán et al., 2001; Larson et al., 2001; Peippo et al., 2001). Differences in secretory activity have also been found between female and male IVP embryos, where female blastocysts release more IFNT in the culture medium than their male counterparts (Larson et al., 2001). This is further supported by other authors which reported a positive effect of the embryokine CSF2 on conceptus length and IFNT production in male embryos but not in females (Dobbs et al., 2014). Likewise, in humans and mice, its well-known that male embryos have greater vulnerability than females to environmental hazardous, altering the sex ratio (Radin et al., 2015), also suggesting that the uterine environment might alter the development of males and females differently.
Thus, the objective of this study was to investigate the sex effect on the transcriptome of IVP and IVD placentas on days 18 and 32 of pregnancy and to delineate biological pathways that contribute to pregnancy failure in IVP-derived pregnancies in cattle.
Details of the animals included in the study and methods for collections and management were detailed in Drum (2019) and in Figure 1. Briefly, a total of 162 Nelore (Bos indicus) cows were used, and from that, 40 cows were selected as oocyte donors. The remaining 122 cows were enrolled in a GnRH-BE synchronization protocol. On the day of artificial insemination (AI), 50 cows were inseminated, and the 73 were assigned to receive a single IVP embryo 6.5 days later if a corpus luteum was present. Pregnancy was confirmed by the presence of an elongated conceptus (day 18 uteri), or by ultrasonography on day 31 before slaughter (day 32 uteri). A total of 56 samples were collected being 25 AI and 31 IVP. The experiment was conducted at the Experimental Station Hildegard Georgina Von Pritzelwiltz, located in Londrina, PR, Brazil. Animal procedures were performed under the approval of the Animal Research Ethics Committee of the College of Agriculture (ESALQ)/University of São Paulo.
Figure 1. Experimental design. Donors were aspirated (OPU) on day-1. On day 0, was the day of insemination (AI) for AI group. After 6.5 days, IVP group, received an embryo transfer (ET). On day 18, half of the animals from each group had the uterus collected. On day 32, cows confirmed pregnant were collected. A fragment of the conceptuses recovered on day 18 and placentas on day 32 days were collected and snap frozen for posterior RNA/DNA extraction.
On day-1, 40 cows previously selected had OPU performed by a trained technician, following the procedure previously described (Seneda et al., 2001). Visible follicles > 2 mm in diameter were aspirated using a real-time B-mode ultrasound scanner, equipped with a 7.5-MHz convex array transducer (Mindray DP 2200, Mindray Bio-Medical Electronics Co. Ltd., Shenzhen, China) fitted into an intravaginal guide and a stainless-steel guide. The follicular puncture was performed using a disposable 19-gauge hypodermic needle connected to a 50-mL conical tube via silicon tubing (0.8 mm; 2.0 mm id). Aspiration was performed using a vacuum pump (WTA, Watanabe, Cravinhos, SP, Brazil) with a negative pressure of 60 mm Hg. The collection medium used was phosphate buffer solution (PBS, Nutricell, Campinas, SP, Brazil) with 10,000 IU/L sodium heparin (Sigma H-3149). After aspiration, fluid was filtered and backwash into a petri dish containing fresh collection medium as previously described (Seneda et al., 2001). Cumulus-oocyte complexes (COCs) were recovered and classified according to the presence of cumulus cells layers and ooplasm quality using the criteria: more than three layers of cumulus cells was classified as good, at least one layer of cumulus cells was classified as regular, and atretic with dark cumulus or no cumulus cells and signs of cytoplasmic degeneration were considered denuded or atretic. Good and regular oocytes were considered viable and used, whereas denuded and atretic oocytes were discarded. Procedures for in vitro maturation (IVM), in vitro fertilization (IVF), and in vitro culture (IVC) were performed as previously described by Seneda et al. (2001) and Silva-Santos et al. (2014). For fertilization, frozen/thawed sperm were used from the same Aberdeen Angus sire used for AI. By the end of IVC, embryos were evaluated on d6 (d0 = day of IVF and day of AI for the control group) according to International Embryo Technology Society (IETS) criteria (Stringfellow and Seidel, 1998).
Cows were slaughtered on day 18 and 32 after AI (d0). On each of the collection days, the uteri were collected immediately after slaughter. The tracts were placed in plastic bags and kept on ice until processing. For the day 18 group, uteri were flushed using 20 mL of sterile saline solution. Pregnancy was confirmed by the presence of an elongated conceptus. Conceptuses were disposed linearly in a petri dish and measured using a ruler. For cows slaughtered on day 32, 1 day before slaughter (day 31), pregnancy diagnosis was performed using ultrasonography to detect fetal heart beating and only pregnant cows were collected. A piece of trophectoderm on day 18 or chorioallantois on day 32 were snap frozen for posterior analysis.
The PAG1 concentration were analyzed from blood collected at the time of the pregnancy diagnosis on day 31. Blood samples were collected by puncture of the coccygeal vein or artery into evacuated 10 mL tubes containing sodium heparin (Vacutainer, Dickinson, Franklin Lakes, NJ). Immediately after collection, the tubes were placed on ice and kept refrigerated until processing. Blood samples were centrifuged at 1,700 x g for 15 min and aliquots of plasma were frozen and stored in duplicates at −20°C until assayed. A commercial quantitative ELISA assay kit was used (Biopryn, BioTracking LLC, Moscow, ID) as previously described (Toledo et al., 2017; Drum et al., 2020).
Total DNA and RNA from day 18 conceptuses were isolated from a maximum of 30 mg of tissue using the All-Prep DNA/RNA/Protein Mini kit (Qiagen, Valencia, CA), according to the manufacturer's protocol. For day 32 placentas, total RNA was isolated using Tri Reagent (Molecular Research Center, Cincinnati, OH) according to the manufacturer's instructions. In both, RNA was treated with DNaseI during RNA purification using the RNase-Free DNase Set (Qiagen) to eliminate DNA contamination. A separate piece was used for DNA isolation following previously described procedures (Ortega et al., 2018). Briefly, a small piece of tissue was collected using a pipette tip and added to a tube containing 6 μl of embryo lysis buffer and subjected to incubation at 60°C for 30 m, followed by 10 m at 85°C in an Eppendorf Mastercycler® Nexus (Eppendorf, Hauppauge, NY). The RNA and DNA concentrations were measured using NanoDrop 2000 spectrophotometer (Thermo Scientific, Rockford, IL), and RNA integrity was determined by quantitative high sensitivity RNA analysis on the Fragment Analyzer instrument (Advanced Analytical Technologies, Inc., Ankeny, IA).
All the conceptuses/placentas were sexed using a polymerase chain reaction (PCR). The primers and procedure were performed as previously described (Park et al., 2001; Xiao et al., 2021). Briefly, each PCR reaction consisted of 3 μL of DNA sample, 2 μL of 10X PCR buffer, 1.6 μL of dNTPs mix (0.4 mM each dNTP), 0.4 μL of each primer (10 μM primer solution), 0.25 μL of Hot-Start Taq-polymerase (Takara Bio, Mountain View, CA, USA), and 12.35 μL of nuclease-free water for a total volume reaction of 17 μL. A Y-linked region of DNA was amplified for 20 cycles of 95°C for 15 s, 58°C for 15 s, and 72°C for 15 s in the first round of PCR. Subsequently, 1 μL of primers amplifying an autosomal gene was added to the resulting PCR reaction, and an additional 17 cycles of PCR were performed under the same conditions. PCR Products (20 μL) were mixed with 5 μL of Orange Dye and fragments were separated by electrophoresis in a 1.5% (wt/vol) agarose gel. The sex of the embryo was determined as male if both the Y-linked amplicon and autosomal amplicon were present and as female if the only amplicon present was the autosomal amplicon. From the cohort of embryos collected, three samples per sex/group/day were chosen for transcriptome analysis.
Samples were sequenced to an average read count of 50 million per sample using 75 bp paired-end sequencing carried out on the Illumina NovaSeq by the University of Missouri Genomics Technology Core and GENOHUB facilities as previously described (Geary et al., 2016). The quality of fastq files of sequences data was checked with a FastQC tool (https://www.bioinformatics.babraham.ac.uk/projects/fastqc/). Illumina adapters were trimmed and reads with fewer than 10 nucleotides were discarded. After quality control, reads were mapped to the bovine reference genome (Bos_taurus.ARS-UCD1.2) using the Hisat2 (Kim et al., 2015). Read counts were determined using FeatureCounts program (Liao et al., 2014). Differentially expressed genes (DEGs) between sample groups were determined by edgeR-robust (Zhou et al., 2014), using a false discovery rate (FDR) <0.05 was used as a threshold for statistical significance. Two main comparisons were made according to the sex of the embryos: first, the source of the embryo (AI vs. IVP) and second, the day of pregnancy (day 18 vs. 32). The DEGs found in each comparison were subjected to gene enrichment analysis using the ToppFun algorithm (Chen et al., 2009).
For PAG 1 concentration and conceptus length the statistical analysis was performed with group effects using the MIXED procedure with models fitting a Gaussian distribution of the statistical analysis system SAS (V9.4) and are presented in Means ± SEM. The male and female ratio among groups was analyzed by Chi-square. Differences were considered significant for P ≤ 0.05.
An average of 27.0% (159/588) blastocyst rate per the total number of cumulus-oocyte-complexes (COCs) collected and 34.2% (129/465) blastocyst rate per viable COCs were obtained. A total of 73 high-quality expanded blastocysts (7-1) were transferred, of which, 38 resulted in a pregnancy (52% P/ET). From these pregnancies, there was 33.87% P/ET (17/39) on day 18 and 61.76% (21/34) on day 32. The AI group had 30 pregnancies from 50 inseminations (60% P/AI), being even in both groups (15/25 day 18 and 15/25 day 32. Embryos not consistent with the stage (<17 cm), or fragmented, making the measurement unfeasible, were excluded from the further analysis.
The sex ratio for each group is depicted in Table 1. The only group that presented a significant difference (P = 0.004) in the sex ratio was day 32 for the IVP group, where 75% of the embryos were females. There was no difference (P > 0.05) in conceptus length comparing males and females (Figure 2A), or on circulating PAG1 on day 32 (Figure 2B).
Table 1. Sex ratio for embryos from artificial insemination (AI) or produced in vitro (IVP) on days 18 and 32 of pregnancy.
Figure 2. Physical and endocrine comparison of female and male pregnancies at day 18 and 32 of development. (A) Distribution of the length of conceptuses on day 18 from AI or IVP. (B) Circulating concentrations of pregnancy associated glycoprotein 1 (PAG1) on day 31 of pregnancy, comparing sex and technique. Data are least square means ± SEM. Mean conceptus length is represented by the dashed line.
There were 8,266 DEGs on day 18, with increased expression of 3,973 genes in females and 4,293 in male conceptuses (Figure 3A). Interestingly, 15 genes from the PAGs family (PAG3, PAG4, PAG5, PAG6, PAG7, PAG8, PAG9, PAG10, PAG14, PAG16, PAG17, PAG18, PAG20 and PAG21; Figure 3B) and TKDPs (TKDP1, TKPD2 and TKPD4) were found increased in female conceptuses. On the other hand, PAG1 was found increased in the male conceptuses (Figure 3B), as well as genes related to maternal recognition of pregnancy, such as IFNAR1, IFN-TAU, IFNT, and IFNT2. Gene ontology of biological processes and pathways are shown in Supplementary Tables 1–4. On day 32, 8,694 DEGs were found, with 5,174 genes with increased expression in females and 3,520 in male placentas (Figure 3C). Among the genes with increased expression in female placentas are TKDP4, PLAC8A and PLAC8B, while PAG1, IFNAR1, IGF1 and IGF2R were increased in male placentas (Figure 3D). Gene ontology of biological processes and pathways are shown in Supplementary Tables 5–8.
Figure 3. Differentially expressed genes (DEGs) of the AI extraembryonic membranes comparing male vs female. (A) Volcano plot representing the number of DEGs comparing male vs female on day 18 conceptuses. Black dots are not significant (FDR > 0.05), Red dots are significant (FDR < 0.05), Gray line at FDR = 0.05, Black dashed lines delimit Fold Change (FC) −1.3 to 1.3. (B) Representative genes upregulated in the female conceptuses (PAG9) and in male conceptuses (PAG1). (C) Volcano plot representing the number of DEGs comparing male vs female on day 32 conceptuses. Black dots are not significant (FDR > 0.05), Red dots are significant (FDR < 0.05), Gray line at FDR = 0.05, Black dashed lines delimit Fold Change at−1.3 to 1.3. (D) Representative gene upregulated in the female conceptuses (PAG6) and in the male conceptuses (PAG1). *FDR < 0.05. AI, artificial insemination.
When comparing males and females IVP conceptuses and placentas, on day 18, there were 6,753 DEGs, with 3,091 genes increased in females, and 3,662 in male conceptuses (Figure 4A). Similar to what was found in AI, some genes identified with increased expression in female conceptuses belong to the PAGs family: PAG8, PAG6, PAG19, PAG18, PAG17, PAG12, and PAG10, as well as TKDP4 and PLAC8A which are also associated with placental function. In male conceptuses, there was increased expression of IFNT, IFNT2 IFNT3, and IFN-T (Figure 4B). Gene ontology of biological processes and pathways are shown in Supplementary Tables 9–12. On day 32, there were 6772 DEGs, with 3,252 genes with increased expression in female, and 3,520 in male placentas (Figure 4C). Among the genes increased in female placentas are genes involved in placentation: PAG17, PAG19, PAG9, and PLAC8B (Figure 4D). On the other hand, similar to AI placentas, PAG1 was detected as highly expressed in male placentas (Figure 4D). Gene ontology of biological processes and pathways are shown in Supplementary Tables 13–16.
Figure 4. Differentially expressed genes (DEGs) of the IVP extraembryonic membranes comparing male vs. female. (A) Volcano plot representing the number of DEGs comparing male vs female on day 18 conceptuses. Black dots are not significant (FDR > 0.05), Red dots are significant (FDR < 0.05), Gray line at FDR = 0.05, Black dashed lines delimit Fold Change at −1.3 to 1.3. (B) Representative genes upregulated in the female conceptuses (PAG12) and in male conceptuses (IFNT). (C) Volcano plot representing the number of DEGs comparing male vs female on day 32 conceptuses. Black dots are not significant (FDR > 0.05), Red dots are significant (FDR < 0.05), Gray line at FDR = 0.05, Black dashed lines delimit FC −1.3 to 1.3. (D) Representative gene upregulated in the female conceptuses (PAG10) and in the male conceptuses (PAG1). *FDR < 0.05. IVP, in vitro produced embryos.
The total number of DEGs for the comparisons AI vs. IVP are depicted in Supplementary Figure 1. There were 71 DEGs between IVP and AI female conceptuses, with 50 genes were increased in the IVP group and 21 in the AI group (Figure 5A; Supplementary Table 17). Genes related to the IGF system (IGF2, IGFBP4H), interferon-stimulated genes (ISG15), steroid synthesis (CYP11A1), and lipid metabolism (APOB, APOA2, APOE) were increased in IVP group (Figure 5B). On the other hand, genes related to prostaglandin metabolism (PTGR1) and trophoblast growth (TKDP2) were increased in the AI group (Figure 5B). On day 32, female placentas had 74 DEGs, with 21 increased in AI and 53 in IVP placentas (Figure 5C; Supplementary Table 18). Interestingly, a gene with increased expression in the AI group is associated with steroid synthesis (CYP17A1; Figure 5D). Genes associated with lipoprotein metabolism (APOB, APOH), prostaglandin synthesis (PTGIS), and IGF-system (IGFBP1) were found also increased in the IVP group (Figure 5D).
Figure 5. Differentially expressed genes (DEGs) in female conceptuses on day 18 and in female placentas on day 32. (A) Volcano plot representing the number of DEGs comparing IVP vs AI on day 18 conceptuses. Black dots are not significant (FDR > 0.05), Red dots are significant (FDR < 0.05), Gray line at FDR = 0.05, Black dashed lines delimit FC −1.3 to 1.3. Representative DEGs of important roles during this time period are shown in (B). (C) Volcano plot representing the number of DEGs comparing IVP vs AI on day 32 conceptuses. Black dots are not significant (FDR > 0.05), Red dots are significant (FDR < 0.05), Gray line at FDR = 0.05, Black dashed lines delimit FC −1.3 to 1.3. Some representative DEGs of important roles during this time period are shown in (D). *FDR < 0.05. AI, artificial insemination; IVP, in vitro produced embryos.
For male conceptuses on day 18, 22 DEGs were found, with 15 being increased in AI and 7 in IVP conceptuses (Figure 6A; Supplementary Table 19). The heat shock protein (HSPA1A) was increased in the IVP male conceptuses, while collagen type V alpha 2 chain gene (COL5A2) and a gene related to lipid metabolism (MTTP) were increased in AI conceptuses (Figure 6B). In contrast, male placentas on day 32 had 899 DEGs, 564 upregulated in AI, and 335 in IVP placentas (Figure 6C). In IVP male placentas, gene ontology terms associated with upregulated genes were related to embryo development (SOX11, COL1A1, SOX6, COL5A1, COL7A1, COL11A1), embryo morphogenesis (MYH3, AHI1, PDGFRA, PAX8, PAX2, PAX3, etc.), and developmental growth (EPPK1, PDGFRB, ESR1, PTPRS, GLI3, COL9A1, COL27A1, APOE). For male AI placentas, the increased genes were associated with lipid metabolism (SLC25A17, PLA2G3, LGMN, CYP51A1, AKR1D1, PTDSS1, SLC16A11, HSD17B12, CYP2U1, TNFRSF1A, HSD17B11) and carbohydrate metabolism (GALNT1, GALNT2, GALNT3, TMEM165, SLC35B2, TMEM258, HSD17B12, COX7A2L). Interestingly, pregnancy associated glycoproteins (PAG10, PAG19, PAG7, and PAG9) and PLAC8B were found to have decreased expression in the IVP group (Figure 6D). The complete list of gene ontology terms for biological processes and pathways with increased or decreased expression in IVP embryos are shown in Supplementary Tables 20–23.
Figure 6. Differentially expressed genes (DEGs) of the male conceptuses on day 18 and placentas on day 32. (A) Volcano plot representing the number of DEGs comparing IVP vs AI on day 18 conceptuses. Black dots are not significant (FDR >0.05), Red dots are significant (FDR < 0.05), Gray line at FDR = 0.05, Black dashed lines delimit FC −1.3 to 1.3. Some representative DEGs of important roles during this time period, are shown in (B). (C) Volcano plot representing the number of DEGs comparing IVP vs AI on day 32 conceptuses. Black dots are not significant (FDR >0.05), Red dots are significant (FDR < 0.05), Gray line at FDR = 0.05, Black dashed lines delimit FC −1.3 to 1.3. Some representative DEGs of important roles during this time period are shown in (D) *FDR < 0.05. AI, artificial insemination; IVP, in vitro produced embryos.
Embryo manipulation has an important impact on pregnancy outcomes (Wiltbank et al., 2018). Differences between IVP and IVD embryos have been previously reported regarding embryonic disc diameter (Bertolini et al., 2002a,b), gene expression at the blastocyst stage (Driver et al., 2012), endometrium response to the embryo (Bauersachs et al., 2009; Mansouri-Attia et al., 2009; Sandra et al., 2011; Mathew et al., 2019) or pregnancy rate (Wiltbank et al., 2016). This study has its uniqueness by providing important new information regarding the endocrine and molecular profiles in two different periods, through IFNT and post-IFNT releasing period in pregnancy. Several studies have described the differences between IVP and IVD embryos, especially comparing the first days of development (Rizos et al., 2002b,c; Corcoran et al., 2006; Clemente et al., 2011; Driver et al., 2012). However, only a few studies compared these embryos during the elongation period, maternal recognition time, and after the first month of pregnancy, during the peri-implantation period (Betsha et al., 2013; Barnwell et al., 2016; Biase et al., 2016).
Surprisingly, in this study, a relatively small number of genes were found differentially expressed on day 18 when comparing IVP and AI. This data suggests that conceptuses derived by AI and IVP are largely similar in their transcriptome at this time point. However, some authors have shown that they are capable to induce different gene expression responses from the endometrium (Bauersachs et al., 2009; Mansouri-Attia et al., 2009; Sandra et al., 2011) which could translate to different pregnancy outcomes later in pregnancy. Among the genes found differentially expressed, several genes were involved in the uptake, intracellular metabolism and/or transport of substrates (glucose, mannose, fructose and fatty acid) which are crucial for maternal-embryo crosstalk and successful implantation (Mansouri-Attia et al., 2009). This suggests that, potentially, the high pregnancy loss reported for the time of maternal recognition (Wiltbank et al., 2016), might be related to the capacity of the embryos from assisted reproductive technologies (ARTs) to communicate with the endometrium and optimize the uterine environment. Another example is the SCNT-derived embryos, that despite presenting lower gene expression variability at day 7 of development, have a much lower pregnancy rate compared to IVP embryos (Smith et al., 2005; Wiltbank et al., 2016). These two techniques were compared before, and they both affected the physiology of the endometrium qualitatively (biological functions) and quantitatively (gene fold expression) when compared with AI controls (Mansouri-Attia et al., 2009).
In line to what was found in this work, another study comparing AI, IVP, and cloned embryos on day 16 showed around 80 DEGs when comparing IVP and AI conceptuses. Likewise, genes involved in trophectoderm development such as the trophectoderm-specific gene TKDP2 was downregulated in the IVP conceptuses, and pathways related to lipid synthesis and metabolism were altered in IVP conceptuses (Betsha et al., 2013). TKDPs (trophoblast Kunitz domain proteins) are proteins that are secreted by conceptuses in the period prior to implantation. These proteins are likely to be important in the interaction between the developing placenta and the endometrium. Along with the results of the present study, this is suggestive that the lipid metabolism of IVP embryos is not only altered during the first week of development (Lonergan et al., 2003a; Stoecklein et al., 2021), but this effect seems to be carried throughout the early pregnancy as well.
Lipid metabolism has been frequently identified as a process differentially expressed between IVP, and IVD elongating conceptuses (Hue et al., 2012; Ribeiro et al., 2012, 2016). In this study, genes such as APOE, APOB, and APOA2, described as part of the lipoprotein metabolism were highly expressed in IVP female conceptuses on day 18. Furthermore, genes involved in lipid metabolism such as SLC25A17, CYP51A1, AKR1D1, SLC16A11, HSD17B12, CYP2U1, HSD17B11, were decreased in the IVP male placentas on day 32. Lipids are used by trophectoderm cells for nutrition, homeostasis, signaling, and coordination of gene expression (Ribeiro et al., 2016). Lipids and fatty acids are also precursors for prostaglandin and steroid biosynthesis. In particular, the gene apolipoprotein A-1 (APOA1) is expressed in cattle during conceptus elongation and is one of the twenty most highly expressed genes from Day 7–19 of gestation (Mamo et al., 2011, 2012; Forde et al., 2013).
Another evidence of different placental development between ARTs, in our data is the increased expression of TKDP2 in the female AI conceptuses. The TKDPs are a family of proteins secreted by conceptuses in the period prior to implantation (MacLean II et al., 2003). They are important in the interaction between the developing placenta and the maternal uterine endometrium (MacLean II et al., 2003; Mamo et al., 2012). Betsha et al. (2013), also found this same gene upregulated in AI embryos when compared to IVP and cloned embryos. The difference in expression of trophoblast-placental related proteins of the ARTs during a crucial time of attachment could help explain the differences in pregnancy success observed when comparing ARTs to AI.
Aiming to detect potential cross-effects of embryo source and sex in placentation we analyzed the circulating PAG1 in the day 31 cows. The PAG1 concentration results contraries previous suggestions that IVF and AI-derived embryos release a different amount of this protein during pregnancy (Pohler et al., 2016) as well as it was not different between females and males. Nevertheless, this hypothesis was not completely discarded in this study, first, due to our small sample size, and second, the family of PAGs is composed of more than 20 proteins divided into ancient and modern groups and are released in different patterns throughout pregnancy (Green et al., 2000). Indeed, results from this study indicates expression of different PAGs at each timepoint analyzed. On day 32 placentas, PAG7, PAG9, PAG10, and PAG19 were found upregulated in AI male embryos when compared to the in vitro counterparts. Also, IVP females presented increased expression PAG9, PAG17, and PAG19 upregulated in comparison to the males. Knowing that PAG 7, 9 and 17, are modern PAGs, exclusively produced by binucleate cells (Green et al., 2000), these results suggest that male placentas produced in vitro might have impaired formation or function of the binucleate cells, leading to potential effects on pregnancy maintenance.
There were also differences in PAGs expression in males and females in this study, where 15 PAGs genes had increased expression in females from AI on day 18, while only PAG1 was increased in AI males. However, male conceptuses from both AI and IVP presented greater expression of IFNT-related genes. These results suggest that maybe, female conceptuses transition from the through-IFNT to post-IFNT period sooner than the males. Also, the expression of these factors seem to be altered at some level by ARTs, since not all the same PAGs were altered in AI and IVP females.
Early embryo studies have reported that male embryos develop faster to 8-cell stage in vitro than their female counterparts (Yadav et al., 1993) but female embryos produced in vitro had greater IFNT2 expression than male embryos at Day 14 (Bermejo-Alvarez et al., 2011). The same study also revealed that female conceptuses were less active concerning oxidative phosphorylation, with many genes of this pathway downregulated (Bermejo-Alvarez et al., 2011). Using IPA analysis to predict cellular processes that would change as a result of gene expression variation between female and male embryos, a study indicated that female embryos were likely to be less active than male embryos in the translation of protein, metabolism of protein, and transport of lipids (Dobbs et al., 2014). The decreased metabolism of female embryos could reflect the earlier onset of elongation and reduced metabolism as the rate of growth decreases at the end of the elongation period (Dobbs et al., 2014). Together, our results and previous reports, suggest that the male embryos might be in a different developmental stage than females, as indicated by the decreased expression of genes associated with placental development, such as PAGs expression. Other authors reported similar alterations in PAGs as well as, another placental factor, PLAC8 (El-Sayed et al., 2006; Salilew-Wondim et al., 2013; Pillai et al., 2019). The biological functions of the PLAC8 protein is not fully determined but it seems to be involved in the endometrium-trophoblast interactions (Mansouri-Attia et al., 2009).
In conclusion, our data indicates that the in vitro system seems to affect the male embryos more prominently than the females. The sexual dimorphism encountered in early stages of embryo development is still present in advanced stages (day 32), especially regarding genes associated with placentation. More studies need to be done to understand the mechanisms by which the placental factors such as PAGs, are acting differently in male and female embryos in vitro.
The datasets presented in this study can be found in online repositories. The names of the repository/repositories and accession number(s) can be found below: Gene Expression Omnibus, GSE192417.
The animal study was reviewed and approved by Animal Research Ethics Committee of the College of Agriculture (ESALQ)/University of São Paulo.
JD, RS, MW, and MO designed the research. JD and GM worked on the animal handling, collected, and processed the samples. MS and CR produced the embryos in vitro. JD and MO analyzed data, prepared the figures, and wrote the paper. All other authors reviewed, corrected, and approved the final manuscript.
This work was funded by the São Paulo Research Foundation (FAPESP, São Paulo, Brazil, Project 2018/03798-7), National Council for Scientific and Technological Development (CNPq 147856/2016-7, Brasília, Brazil), and the Agriculture and Food Research Initiative Competitive (Grant No. 2019-67015-28998) from the USDA National Institute of Food and Agriculture supported the study. The FAPESP, CNPq, and USDA did not participate in any component of the study design, collection, analysis, interpretation of data, or in writing the manuscript.
The authors declare that the research was conducted in the absence of any commercial or financial relationships that could be construed as a potential conflict of interest.
All claims expressed in this article are solely those of the authors and do not necessarily represent those of their affiliated organizations, or those of the publisher, the editors and the reviewers. Any product that may be evaluated in this article, or claim that may be made by its manufacturer, is not guaranteed or endorsed by the publisher.
The authors thank Laísse G. Lima, José R. S. Gonçalves, and the staff of Experimental Station Hildegard Georgina Von Pritzelwitz (Londrina, PR, Brazil) for the use of their cows, facilities, and all the technical support; Prof, Danila B. Campos, Maria C. G. Macêdo, João V. R. Vicente, Mario Oliveira; Lucas O. Silva and Rodrigo L. O. R. Alves for helping with animal handling and sample collection. Prof. Fábio Bim and Prof. Marcelo Araújo for providing structure for sample processing. We thank the University of Missouri Genomics Technology Core for technical support.
The Supplementary Material for this article can be found online at: https://www.frontiersin.org/articles/10.3389/fanim.2022.807217/full#supplementary-material
Supplementary Figure 1. The overall number of differentially expressed genes (DEGs) obtained from the RNA-sequencing analysis. Numbers of DEGs in the AI (black) and IVP (gray). Comparison according to sex. AI, Artificial insemination; IVP, in vitro produced embryos.
Barnwell, C. V., Farin, P. W., Ashwell, C. M., Farmer, W. T., Galphin, S. P. Jr., et al. (2016). Differences in mRNA populations of short and long bovine conceptuses on Day 15 of gestation. Mol. Reprod. Dev. 83, 424–441. doi: 10.1002/mrd.22640
Bauersachs, S., Ulbrich, S. E., Zakhartchenko, V., Minten, M., Reichenbach, M., Reichenbach, H. D., et al. (2009). The endometrium responds differently to cloned versus fertilized embryos. Proc. Natl. Acad. Sci. U. S. A. 106, 5681–5686. doi: 10.1073/pnas.0811841106
Bermejo-Alvarez, P., Rizos, D., Lonergan, P., and Gutierrez-Adan, A. (2011). Transcriptional sexual dimorphism in elongating bovine embryos: implications for XCI and sex determination genes. Reproduction 141, 801–808. doi: 10.1530/REP-11-0006
Bertolini, M., Beam, S. W., Shim, H., Bertolini, L. R., Moyer, A. L., Famula, T. R., et al. (2002a). Growth, development, and gene expression by in vivo- and in vitro-produced day 7 and 16 bovine embryos. Mol. Reprod. Dev. 63, 318–328. doi: 10.1002/mrd.90015
Bertolini, M., Mason, J. B., Beam, S. W., Carneiro, G. F., Sween, M. L., Kominek, D. J., et al. (2002b). Morphology and morphometry of in vivo- and in vitro-produced bovine concepti from early pregnancy to term and association with high birth weights. Theriogenology 58, 973–994. doi: 10.1016/S0093-691X(02)00935-4
Betsha, S., Hoelker, M., Salilew-Wondim, D., Held, E., Rings, F., Große-Brinkhause, C., et al. (2013). Transcriptome profile of bovine elongateed conceptus obtained from SCNT and IVP pregnancies. Mol. Reprod. Dev. 80, 315–333. doi: 10.1002/mrd.22165
Biase, F. H., Rabel, C., Guillomot, M., Hue, I., Andropolis, K., Olmstead, C. A., et al. (2016). Massive dysregulation of genes involved in cell signaling and placental development in cloned cattle conceptus and maternal endometrium. Proc. Natl. Acad. Sci. USA 113, 14492–14501. doi: 10.1073/pnas.1520945114
Chen, J., Bardes, E. E., Aronow, B. J., and Jegga, A. G. (2009). ToppGene Suite for gene list enrichment analysis and candidate gene prioritization. Nucleic Acids Res. 37, W305–W311. doi: 10.1093/nar/gkp427
Clemente, M., Lopez-Vidriero, I., O'Gaora, P., Mehta, J. P., Forde, N., Gutierrez-Adan, A., et al. (2011). Transcriptome changes at the initiation of elongation in the bovine conceptus. Biol. Reprod. 85, 285–295. doi: 10.1095/biolreprod.111.091587
Corcoran, D., Fair, T., Park, S., Rizos, D., Patel, O. V., Smith, G. W., et al. (2006). Suppressed expression of genes involved in transcription and translation in in vitro compared with in vivo cultured bovine embryos. Reproduction 131, 651–660. doi: 10.1530/rep.1.01015
Dobbs, K. B., Gagné, D., Fournier, E., Dufort, I., Robert, C., Block, J., et al. (2014). Sexual dimorphism in developmental programming of the bovine preimplantation embryo caused by colony-stimulating factor 21. Biol. Reprod. 91, 80. doi: 10.1095/biolreprod.114.121087
Driver, A. M., Peñagaricano, F., Huang, W., Ahmad, K. R., Hackbart, K. S., Wiltbank, M. C., et al. (2012). RNA-Seq analysis uncovers transcriptomic variations between morphologically similar in vivo- and in vitro-derived bovine blastocysts. BMC Genomics 13, 118. doi: 10.1186/1471-2164-13-118
Drum, J. N. (2019). Mechanisms Involved in Maintaining the Corpus Luteum During the First Two Months of Pregnancy. Piracicaba: Luiz de Queiroz College of Agriculture.
Drum, J. N., Wiltbank, M. C., Monteiro, P. L. J., Prata, A. B., Gennari, R. S., Gamarra, C. A., et al. (2020). Oxytocin-induced prostaglandin F2-alpha release is low in early bovine pregnancy but increases during the second month of pregnancy. Biol. Reprod. 102, 412–423. doi: 10.1093/biolre/ioz169
El-Sayed, A., Hoelker, M., Rings, F., Salilew, D., Jennen, D., Tholen, E., et al. (2006). Large-scale transcriptional analysis of bovine embryo biopsies in relation to pregnancy success after transfer to recipients. Physiol. Genomics 28, 84–96. doi: 10.1152/physiolgenomics.00111.2006
Forde, N., Mehta, J. P., McGettigan, P. A., Mamo, S., Bazer, F. W., Spencer, T. E., et al. (2013). Alterations in expression of endometrial genes coding for proteins secreted into the uterine lumen during conceptus elongation in cattle. BMC Genomics 14, 321. doi: 10.1186/1471-2164-14-321
Gad, A., Hoelker, M., Besenfelder, U., Havlicek, V., Cinar, U., Rings, F., et al. (2012). Molecular mechanisms and pathways involved in bovine embryonic genome activation and their regulation by alternative in vivo and in vitro culture conditions1. Biol Reprod 87, 100. doi: 10.1095/biolreprod.112.099697
Geary, T. W., Burns, G. W., Moraes, J. G., Moss, J. I., Denicol, A. C., Dobbs, K. B., et al. (2016). Identification of beef heifers with superior uterine capacity for pregnancy. Biol Reprod 95, 47. doi: 10.1095/biolreprod.116.141390
Green, J. A., and Hennessy, M. E. (2018). “Pregnancy associated glycoproteins,” in Encyclopedia of Reproduction, 2nd Edn, ed M. K. Skinner (Oxford: Academic Press), 508–513. doi: 10.1016/B978-0-12-801238-3.64679-2
Green, J. A., Parks, T. E., Avalle, M. P., Telugu, B. P., McLain, A. L., Peterson, A. J., et al. (2005). The establishment of an ELISA for the detection of pregnancy-associated glycoproteins (PAGs) in the serum of pregnant cows and heifers. Theriogenology 63, 1481–1503. doi: 10.1016/j.theriogenology.2004.07.011
Green, J. A., Xie, S., Quan, X., Bao, B., Gan, X., Mathialagan, N., et al. (2000). Pregnancy-associated bovine and ovine glycoproteins exhibit spatially and temporally distinct expression patterns during pregnancy 1. Biol Reprod 62, 1624–1631. doi: 10.1095/biolreprod62.6.1624
Gutiérrez-Adán, A., Granados, J., Pintado, B., and Fuente, J. D. L. (2001). Influence of glucose on the sex ratio of bovine IVM/IVF embryos cultured in vitro. Reprod. Fertil. Dev. 13, 361–365. doi: 10.1071/RD00039
Hill, J. R., Burghardt, R. C., Jones, K., Long, C. R., Looney, C. R., Shin, T., et al. (2000). Evidence for placental abnormality as the major cause of mortality in first-trimester somatic cell cloned bovine fetuses. Biol Reprod 63, 1787–1794. doi: 10.1095/biolreprod63.6.1787
Hue, I., Degrelle, S. A., and Turenne, N. (2012). Conceptus elongation in cattle: genes, models and questions. Anim. Reprod. Sci. 134, 19–28. doi: 10.1016/j.anireprosci.2012.08.007
Kim, D., Langmead, B., and Salzberg, S. L. (2015). HISAT: a fast spliced aligner with low memory requirements. Nat. Methods 12, 357–360. doi: 10.1038/nmeth.3317
Larson, M. A., Kimura, K., Kubisch, H. M., and Roberts, R. M. (2001). Sexual dimorphism among bovine embryos in their ability to make the transition to expanded blastocyst and in the expression of the signaling molecule IFN-τ. PNAS 98, 9677–9682. doi: 10.1073/pnas.171305398
Liao, Y., Smyth, G. K., and Shi, W. (2014). featureCounts: an efficient general purpose program for assigning sequence reads to genomic features. Bioinformatics 30, 923–930. doi: 10.1093/bioinformatics/btt656
Lonergan, P., Rizos, D., Gutiérrez-Adán, A., Moreira, P. M., Pintado, B., de la Fuente, J., et al. (2003a). Temporal divergence in the pattern of messenger RNA expression in bovine embryos cultured from the zygote to blastocyst stage in vitro or in vivo1. Biol. Reprod. 69, 1424–1431. doi: 10.1095/biolreprod.103.018168
Lonergan, P., Rizos, D., Kanka, J., Nemcova, L., Mbaye, A. M., Kingston, M., et al. (2003b). Temporal sensitivity of bovine embryos to culture environment after fertilization and the implications for blastocyst quality. Reproduction 126, 337–346. doi: 10.1530/rep.0.1260337
MacLean, J. A. II., Chakrabarty, A., Xie, S., Bixby, J. A., Roberts, R. M., et al. (2003). Family of Kunitz proteins from trophoblast: Expression of the trophoblast Kunitz domain proteins (TKDP) in cattle and sheep. Mol. Reprod. Dev. 65, 30–40. doi: 10.1002/mrd.10262
Mamo, S., Mehta, J. P., McGettigan, P., Fair, T., Spencer, T. E., Bazer, F. W., et al. (2011). RNA Sequencing reveals novel gene clusters in bovine conceptuses associated with maternal recognition of pregnancy and implantation. Biol. Reprod. 85, 1143–1151. doi: 10.1095/biolreprod.111.092643
Mamo, S., Rizos, D., and Lonergan, P. (2012). Transcriptomic changes in the bovine conceptus between the blastocyst stage and initiation of implantation. Anim. Reprod. Sci. 134, 56–63. doi: 10.1016/j.anireprosci.2012.08.011
Mansouri-Attia, N., Sandra, O., Aubert, J., Degrelle, S., Everts, R. E., Giraud-Delville, C., et al. (2009). Endometrium as an early sensor of in vitro embryo manipulation technologies. PNAS 106, 5687–5692. doi: 10.1073/pnas.0812722106
Mathew, D. J., Sanchez, J. M., Passaro, C., Charpigny, G., Behura, S. K., Spencer, T. E., et al. (2019). Interferon tau-dependent and independent effects of the bovine conceptus on the endometrial transcriptomedagger. Biol. Reprod. 100, 365–380. doi: 10.1093/biolre/ioy199
Ortega, M. S., Moraes, J. G. N., Patterson, D. J., Smith, M. F., Behura, S. K., Poock, S., et al. (2018). Influences of sire conception rate on pregnancy establishment in dairy cattle. Biol. Reprod. 99, 1244–1254. doi: 10.1093/biolre/ioy141
Park, J. H., Lee, J. H., Choi, K. M., Joung, S. Y., Kim, J. Y., Chung, G. M., et al. (2001). Rapid sexing of preimplantation bovine embryo using consecutive and multiplex polymerase chain reaction (PCR) with biopsied single blastomere. Theriogenology 55, 1843–1853. doi: 10.1016/S0093-691X(01)00526-X
Peippo, J., Kurkilahti, M., and Bredbacka, P. (2001). Developmental kinetics of in vitro produced bovine embryos: the effect of sex, glucose and exposure to time-lapse environment. Zygote 9, 105–113. doi: 10.1017/S0967199401001113
Pillai, V. V., Siqueira, L. G., Das, M., Kei, T. G., Tu, L. N., Herren, A. W., et al. (2019). Physiological profile of undifferentiated bovine blastocyst-derived trophoblasts. Biol. Open 8, bio037937. doi: 10.1242/bio.037937
Pohler, K. G., Peres, R. F. G., Green, J. A., Graff, H., Martins, T., Vasconcelos, J. L. M., et al. (2016). Use of bovine pregnancy-associated glycoproteins to predict late embryonic mortality in postpartum Nelore beef cows. Theriogenology 85, 1652–1659. doi: 10.1016/j.theriogenology.2016.01.026
Radin, R. G., Mumford, S. L., Silver, R. M., Lesher, L. L., Galai, N., Faraggi, D., et al. (2015). Sex ratio following preconception low-dose aspirin in women with prior pregnancy loss. J. Clin. Investig. 125, 3619–3626. doi: 10.1172/JCI82357
Ribeiro, E., Monteiro, A. P., Bisinotto, R., Lima, F., Greco, L., Thatcher, W., et al. (2012). Conceptus development and global gene expression at preimplantation stages in lactating dairy cows of distinct genetic groups and estrous cyclic statuses. Reprod. Domest. Anim. 47, 546–546. doi: 10.1111/j.1439-0531.2012.02119.x
Ribeiro, E. S., Monteiro, A. P. A., Bisinotto, R. S., Lima, F. S., Greco, L. F., Ealy, A. D., et al. (2016). Conceptus development and transcriptome at preimplantation stages in lactating dairy cows of distinct genetic groups and estrous cyclic statuses. J. Dairy Sci. 99, 4761–4777. doi: 10.3168/jds.2015-10315
Rizos, D., Fair, T., Papadopoulos, S., Boland, M. P., and Lonergan, P. (2002a). Developmental, qualitative, and ultrastructural differences between ovine and bovine embryos produced in vivo or in vitro. Mol. Reprod. Dev. 62, 320–327. doi: 10.1002/mrd.10138
Rizos, D., Lonergan, P., Boland, M. P., Arroyo-Garcia, R., Pintado, B., de la Fuente, J., et al. (2002b). Analysis of differential messenger RNA expression between bovine blastocysts produced in different culture systems: Implications for blastocyst quality. Biol Reprod 66, 589–595. doi: 10.1095/biolreprod66.3.589
Rizos, D., Ward, F., Duffy, P., Boland, M. P., and Lonergan, P. (2002c). Consequences of bovine oocyte maturation, fertilization or early embryo development in vitro versus in vivo: Implications for blastocyst yield and blastocyst quality. Mol. Reprod. Dev. 61, 234–248. doi: 10.1002/mrd.1153
Salilew-Wondim, D., Tesfaye, D., Hossain, M., Held, E., Rings, F., Tholen, E., et al. (2013). Aberrant placenta gene expression pattern in bovine pregnancies established after transfer of cloned or in vitro produced embryos. Physiol. Genomics 45, 28–46. doi: 10.1152/physiolgenomics.00076.2012
Sandra, O., Mansouri-Attia, N., Lea, R. G., Sandra, O., Mansouri-Attia, N., and Lea, R. G. (2011). Novel aspects of endometrial function: a biological sensor of embryo quality and driver of pregnancy success. Reprod. Fertil. Dev. 24, 68–79. doi: 10.1071/RD11908
Seneda, M. M., Esper, C. R., Garcia, J. M., de Oliveira, J. A., and Vantini, R. (2001). Relationship between follicle size and ultrasound-guided transvaginal oocyte recovery. Anim. Reprod. Sci. 67, 37–43. doi: 10.1016/S0378-4320(01)00113-0
Silva-Santos, K. C., Santos, G. M. G., Koetz, C., Morotti, F., Siloto, L. S., Marcantonio, T. N., et al. (2014). Antral follicle populations and embryo production - in vitro and in vivo- of Bos indicus-taurus donors from weaning to yearling ages. Reprod. Domest. Anim. 49, 228–232. doi: 10.1111/rda.12255
Smith, S. L., Everts, R. E., Tian, X. C., Du, F., Sung, L.-Y., Rodriguez-Zas, S. L., et al. (2005). Global gene expression profiles reveal significant nuclear reprogramming by the blastocyst stage after cloning. PNAS 102, 17582–17587. doi: 10.1073/pnas.0508952102
Stoecklein, K. S., Ortega, M. S., Spate, L. D., Murphy, C. N., and Prather, R. S. (2021). Improved cryopreservation of in vitro produced bovine embryos using FGF2, LIF, and IGF1. PLOS ONE 16, e0243727. doi: 10.1371/journal.pone.0243727
Stringfellow, D. A., and Seidel, S. M. (1998). Manual of the International Embryo Transfer Society (IETS), 4th Edn. Champaign, IL: International Embryo Transfer Society.
Toledo, M. Z., Baez, G. M., Garcia-Guerra, A., Lobos, N. E., Guenther, J. N., Trevisol, E., et al. (2017). Effect of feeding rumen-protected methionine on productive and reproductive performance of dairy cows. PLoS ONE 12, e0189117. doi: 10.1371/journal.pone.0189117
Wallace, R. M., Hart, M. L., Egen, T. E., Schmelzle, A., Smith, M. F., Pohler, K. G., et al. (2019). Bovine pregnancy associated glycoproteins can alter selected transcripts in bovine endometrial explants. Theriogenology 131, 123–132. doi: 10.1016/j.theriogenology.2019.03.026
Wallace, R. M., Pohler, K. G., Smith, M. F., and Green, J. A. (2015). Placental PAGs: gene origins, expression patterns, and use as markers of pregnancy. Reproduction 149, R115–R126. doi: 10.1530/REP-14-0485
Wiltbank, M. C., Baez, G. M., Garcia-Guerra, A., Toledo, M. Z., Monteiro, P. L., Melo, L. F., et al. (2016). Pivotal periods for pregnancy loss during the first trimester of gestation in lactating dairy cows. Theriogenology 86, 239–253. doi: 10.1016/j.theriogenology.2016.04.037
Wiltbank, M. C., Mezera, M. A., Toledo, M. Z., Drum, J. N., Baez, G. M., García-Guerra, A., et al. (2018). Physiological mechanisms involved in maintaining the corpus luteum during the first two months of pregnancy. Anim. Reprod. 15, 805–821. doi: 10.21451/1984-3143-AR2018-0045
Wooding, F. B. P., Roberts, R. M., and Green, J. A. (2005). Light and electron microscope immunocytochemical studies of the distribution of pregnancy associated glycoproteins (PAGs) throughout pregnancy in the cow: possible functional implications. Placenta 26, 807–827. doi: 10.1016/j.placenta.2004.10.014
Xiao, Y., Sosa, F., de Armas, L. R., Pan, L., and Hansen, P. J. (2021). An improved method for specific-target preamplification PCR analysis of single blastocysts useful for embryo sexing and high-throughput gene expression analysis. J. Dairy Sci. 104, 3722–3735. doi: 10.3168/jds.2020-19497
Yadav, B. R., King, W. A., and Betteridge, K. J. (1993). Relationships between the completion of first cleavage and the chromosomal complement, sex, and developmental rates of bovine embryos generated in vitro. Mol. Reprod. Dev. 36, 434–439. doi: 10.1002/mrd.1080360405
Keywords: sexual dimorphism, peri-implantation, assisted reproductive technologies, bovine, in vitro fertilization, artificial insemination
Citation: Drum JN, Madureira G, Rosa CO, Seneda MM, Wiltbank MC, Sartori R and Ortega MS (2022) Male Embryos Produced in vitro Deviate From Their in vivo Counterparts in Placental Gene Expression on Day 32 of Pregnancy. Front. Anim. Sci. 3:807217. doi: 10.3389/fanim.2022.807217
Received: 01 November 2021; Accepted: 21 January 2022;
Published: 16 February 2022.
Edited by:
Graham Cliff Lamb, Texas A&M University College Station, United StatesReviewed by:
Pat Lonergan, University College Dublin, IrelandCopyright © 2022 Drum, Madureira, Rosa, Seneda, Wiltbank, Sartori and Ortega. This is an open-access article distributed under the terms of the Creative Commons Attribution License (CC BY). The use, distribution or reproduction in other forums is permitted, provided the original author(s) and the copyright owner(s) are credited and that the original publication in this journal is cited, in accordance with accepted academic practice. No use, distribution or reproduction is permitted which does not comply with these terms.
*Correspondence: M. Sofia Ortega, b3J0ZWdhb2JhbmRvbUBtaXNzb3VyaS5lZHU=
Disclaimer: All claims expressed in this article are solely those of the authors and do not necessarily represent those of their affiliated organizations, or those of the publisher, the editors and the reviewers. Any product that may be evaluated in this article or claim that may be made by its manufacturer is not guaranteed or endorsed by the publisher.
Research integrity at Frontiers
Learn more about the work of our research integrity team to safeguard the quality of each article we publish.