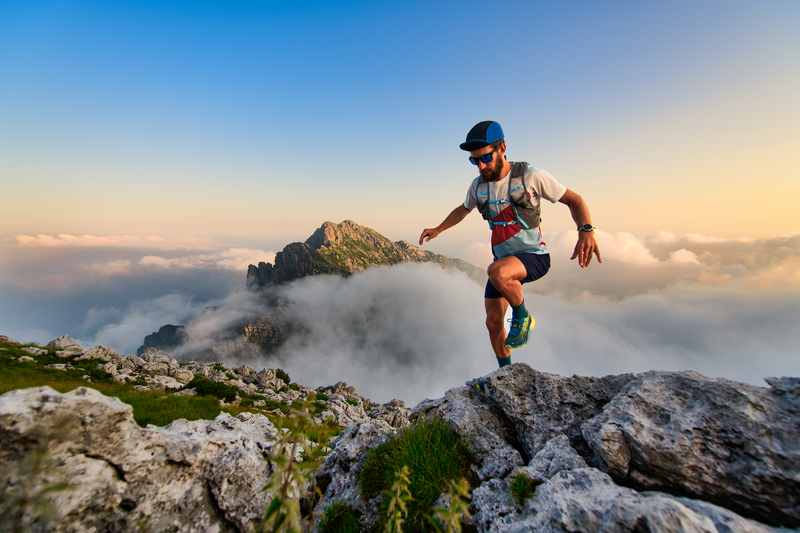
95% of researchers rate our articles as excellent or good
Learn more about the work of our research integrity team to safeguard the quality of each article we publish.
Find out more
REVIEW article
Front. Anim. Sci. , 25 April 2022
Sec. Animal Physiology and Management
Volume 3 - 2022 | https://doi.org/10.3389/fanim.2022.778440
This article is part of the Research Topic The Influences of Early Life Experiences on Future Health and Productivity View all 9 articles
Environmental perturbations during gestation can alter fetal development and postnatal animal performance. In humans, intrauterine growth restriction (IUGR) resulting from adaptive fetal programming is known as a leading cause of perinatal morbidity and mortality and predisposes offspring to metabolic disease, however, the prevalence and impact in livestock is not characterized as well. Multiple animal models have been developed as a proxy to determine mechanistic changes that underlie the postnatal phenotype resulting from these programming events in humans but have not been utilized as robustly in livestock. While the overall consequences are similar between models, the severity of the conditions appear to be dependent on type, timing, and duration of insult, indicating that some environmental insults are of more relevance to livestock production than others. Thus far, maternofetal stress during gestation has been shown to cause increased death loss, low birth weight, inefficient growth, and aberrant metabolism. A breadth of this data comes from the fetal ruminant collected near term or shortly thereafter, with fewer studies following these animals past weaning. Consequently, even less is known about how adaptive fetal programming impacts subsequent progeny. In this review, we summarize the current knowledge of the postnatal phenotype of livestock resulting from different models of fetal programming, with a focus on growth, metabolism, and reproductive efficiency. We further describe what is currently known about generational impacts of fetal programming in production systems, along with gaps and future directions to consider.
Postnatal growth and performance of mammalian species is largely influenced by fetal growth and development, with deviations from normal resulting in modification of typical growth patterns and aberrant metabolism after birth. Hales and Barker (2001) first explained this phenomenon with their thrifty phenotype hypothesis in humans, that credits adaptive fetal responses aimed at sparing nutrients as the epidemiological explanation for postnatal metabolic dysfunction and adult disease. Since then, a concerted effort has been made to understand how external factors during gestation alter the in utero environment, resulting in adaptive fetal growth and function (Gluckman and Hanson, 2004; Flinn et al., 2020). When these responses are not corrected before birth, they will result in lifelong impacts, which is referred to as developmental or fetal programming. These adaptations frequently result in a condition known as intrauterine growth restriction (IUGR) which remains the second leading cause of perinatal morbidity and mortality in humans (Alisi et al., 2011). Improved neonatal care for infants across the developed world, along with increased understanding of IUGR has led to decreased infant mortality in humans (Goldenberg and Culhane, 2007), however, the impacts and management of adaptive fetal programming in livestock species needs further understanding.
In production settings, fetal programming in livestock is likely the result of poor fetal nutrition during gestation. Common industry practices such as breeding peripubertal dams contributes to these environments, as this causes competitive allocation of nutrients between the fetus, placenta, and the still growing dam. Other production standards such as selection for milk yield or lamb prolificacy (Gootwine et al., 2007), the presence of multiple fetuses, poor pasture conditions, as well as environmental perturbations throughout gestational periods can also contribute to poor fetal nutrition (Redmer et al., 2004; Wu et al., 2004, 2006; Reynolds et al., 2010; Yates et al., 2018). Reduced energy reserves and decreased vigor at birth in conjunction with reduced birthweight leads to a significant disadvantage throughout the first few months of life (Dwyer et al., 2016). Similar to IUGR-born children, livestock experiencing compromised fetal growth are at increased risk of perinatal morbidity and mortality, while also being predisposed to metabolic and organ dysfunction (Brown et al., 2015), cardiovascular disease (Wu et al., 2006; Reynolds and Vonnahme, 2017) and poor body composition (De Blasio et al., 2007; Gibbs et al., 2019). Approximately 8% of products from the U.S. livestock industry are lost annually due to a lack of management techniques for low birthweight animals (Wu et al., 2006), necessitating further understanding of the etiology and long-term consequences of adaptive fetal growth. Current research in livestock largely focuses on perinatal mechanistic changes with fewer studies following the postnatal animal. Consequently, there is a gap in knowledge that falls after weaning and in the production and performance of subsequent generations. In this review, we highlight current knowledge regarding postnatal growth, metabolic, and reproductive consequences of adaptive fetal programming as seen in various ruminant models. We further present the limited knowledge of intergenerational programming in ruminants along with areas of focus for future research.
Since the placenta is the sole mediator of nutrient transport between dam and fetus, developmental programming is often the result of placental insufficiency due to placental stunting (Cox and Marton, 2009). In livestock, placental stunting can occur as the result of environmental perturbations, poor nutritional management or illness leading to maternal stress that increases body temperature and repartitions blood flow away from the uterus (Wallace et al., 2005; Greenwood and Cafe, 2007). Impact on the placenta is dependent upon timing and duration of maternal insult (Painter et al., 2005; Van Eetvelde et al., 2016). Maternal stress maintained during early to mid-gestation, when peak placental growth is taking place, is more likely to cause placental stunting than during late gestation when it may instead lead to a direct fetal insult. As the disparity between fetal nutrient and oxygen requirements and those supplied by the placenta grows, the fetus will begin to elicit a series of responses aimed at sparing nutrients for vital organs (Hales and Barker, 1992, 2001; Redmer et al., 2004; Poudel et al., 2015). These responses are first mediated through stress systems that contribute to the redirection of blood flow to prioritize delivery to vital organs at the expense of skeletal muscle and other visceral tissue growth (Galan et al., 1999). Brain sparing activity in conjunction with impaired skeletal muscle growth results in an asymmetric growth pattern (Galan et al., 1999; Macko et al., 2013) and by term, fetal growth can be reduced by up to 50% compared to their uncompromised counterparts. Along with growth disparities, developmental adaptations contribute to a multitude of tissue specific metabolic dysfunction within the pancreas (Limesand et al., 2006; Leos et al., 2010), adipose tissue (Chen et al., 2010), skeletal muscle (Yates et al., 2016; Rozance et al., 2018; Cadaret et al., 2019a), liver (Thorn et al., 2009; Brown et al., 2015), heart, and brain (Poudel et al., 2015) of the fetus. Although these developmental adaptations occur to increase survivability in utero, they elicit life-long growth and metabolic changes that can impact favorable production traits and reduce efficiency in livestock.
Clinical cases of developmental programming can be studied in humans, but animal models have become increasingly useful to understand fetal pathophysiological and mechanistic changes throughout gestation (Anthony et al., 2003; Barry et al., 2006). The ovine model of IUGR is widely accepted as an ideal model (reviewed by Beede et al., 2019), due to similarities with human gestational milestones, as well as relatively comparable developmental stages to other ruminants such as cattle (Anthony et al., 2003). The tendency for singletons or twins, in addition to the average size of offspring, make sheep a more ideal model compared to the small litter-bearing species of rodents that are often used for human studies. Lastly, sheep tend to be more tolerable to surgical manipulation during gestation, with fetal size large enough to allow surgical catheterization to monitor maternofetal gas and nutrient transfer (Morrison, 2008). For these reasons, more literature exists about the effects of fetal programming in sheep, but these models have also been extended to cattle. Although the information provided by animal models is useful in improving perinatal morbidity and mortality rates in humans, in many cases it can also be applicable to livestock settings. With the help of ruminant models of developmental programming, producers can optimize nutrient intake in both critical pre and postnatal settings to improve growth for various food animals. The degree to which developmental programming occurs varies based on the model (Anthony et al., 2003), however, each animal model allows for greater insight into mechanisms of developmental and postnatal growth and metabolic efficiency. In this section, we will cover ruminant models and, when not available, rodent models of developmental programming that mimic naturally occurring insults within production systems including heat stress, maternal inflammation, nutrient restriction, maternal obesity, and fetal exposure to glucocorticoids.
Induction of fetal growth restriction through maternal exposure to high ambient temperatures has created a long-standing animal model for developmental programming. This model has been refined in the ovine, consistently producing placental insufficiency-induced IUGR (PI-IUGR) and entails placement of ewes into conditions ranging from 35 to 40°C with ~35% relative humidity, for 50 consecutive days (Beede et al., 2019). Treatment begins around the 40th day of gestation to coincide with peak placental growth, ultimately causing placental stunting (Hay et al., 2016; Limesand et al., 2018). PI-IUGR has been established through many studies, implicating fetal hypoxia and hypoglycemia as the drivers of adaptive growth (Thureen et al., 1992; Limesand et al., 2018). As placental stunting occurs prior to exponential fetal growth, impacts are not observed until the third trimester when the placenta can no longer meet the demand of the growing fetus resulting in fetal malnutrition.
Sustained maternal hyperthermia from early to late gestation results in greater reduction of placental and fetal mass (up to 64 and 30–60% reduction, respectively) compared to exposure from mid to late gestation (Bell et al., 1989; Galan et al., 1999), substantially increasing perinatal death (Van Wettere et al., 2021). Developmental adaptations become a permanent change to the phenotype of the fetus when the insult is not removed in time, but it is possible for the fetus to experience compensatory growth or even fully restore placental function when the insult is removed before 55 days of gestation in sheep (Galan et al., 1999). Despite these variations, maternal heat stress has been associated with reduced birth weights, reduced survival rates prior to weaning, postnatal metabolic dysfunction, and a reduction in carcass merit in cattle and sheep (Yates et al., 2011; Monteiro et al., 2016; Limesand et al., 2018; Dahl et al., 2019). Catecholamines secreted as a response to hypoxia and hypoglycemia impair insulin secretion and responsiveness contributing to metabolic adaptations that result in increased adiposity and fat storage during early postnatal phases of compensatory growth (Limesand et al., 2006; Yates et al., 2011). Slowed myoblast proliferation leading to decreased fiber size (Yates et al., 2016), and reduced capacity for insulin stimulated glucose utilization (Limesand et al., 2007, 2018; Chen et al., 2010) contribute to altered muscle development and asymmetric growth patterns manifesting as poor body composition in animals after birth. There is also evidence of reduced placental transfer of amino acids (Regnault et al., 2013), along with decreased circulation of anabolic factors further suppressing cardiac and skeletal muscle growth (Bartelds et al., 2000). Additional organ weights such as thymus, spleen, heart, and liver have been shown to be reduced in newborn bull calves after gestational heat stress (Ahmed et al., 2021). Dairy cattle experiencing heat stress in utero can exhibit decreased body weight for up to 1 year of age, along with greater susceptibility to illness caused by comprised passive immunity (Tao et al., 2012; Monteiro et al., 2016; Davidson et al., 2021) putting animals at greater risk of death. However, perinatal heat abatement strategies have been shown to improve dairy calf growth and welfare and may serve as a strategy to prevent or mediate outcomes of in utero heat stress (Dado-Senn et al., 2020). Multiple studies have also demonstrated that late gestation heat stress impacts mammary development, which may contribute to persistent poor calf performance in future generations (Skibiel et al., 2018a,b; Ouellet et al., 2020).
Heat stress is known to impact maternal reproductive health and performance during gestation (recently reviewed by Alves et al., 2020; Van Wettere et al., 2021), but recent studies in dairy cattle indicate it may have lasting effects on adult offspring fertility as well. Indeed, animals whose dams were exposed to heat stress during gestation had longer days to first service, calving to conception interval, greater culling rate, and reduced Anti-Mullerian hormone (AMH) concentrations (Akbarinejad et al., 2017). A study in dairy cows found that heat stress did not appear to effect age of first insemination and parturition but did result in an increase in the number of services per pregnancy, increased age at pregnancy, and fewer calves survived to their first lactation (Monteiro et al., 2016). While there is growing research on the impacts of heat stress on pregnant dairy cows and their progeny fertility, data in grazing ruminants is missing and remains an area needing attention.
Maternofetal inflammatory responses are strongly associated with hypoxia induced by placental insufficiency from various causes (Bertucci et al., 2011). One such cause can be sustained maternal inflammation, like that seen with prolonged illness, which directly causes fetal inflammation and while not confirmed yet, likely placental insufficiency (Beede et al., 2019). Periods of infection, such as those seen with respiratory diseases that are common in livestock species, result in a febrile response and systemic inflammation that can last for multiple weeks (Gifford et al., 2012). Heat stress and lameness can also induce chronic systemic inflammation when not alleviated (Swanson et al., 2020). Much of the research around the influence of inflammation on animal performance is performed outside of gestational windows yet pregnant animals have increased susceptibility as their immune system is altered in early gestation to allow for establishment of pregnancy (Hansen, 2011). Thus, more data is needed to identify the prevalence of systemic inflammation during pregnancy in production settings. In an experimental setting we have implemented a model of maternofetal inflammation-induced IUGR (MI-IUGR) in the sheep and rat that involves the administration of the endotoxin lipopolysaccharide (LPS) to gestating animals, and found similar results to other models of IUGR (Cadaret et al., 2019a,b). Skeletal muscle is highly responsive to inflammatory regulation (Frost et al., 1997), and enhanced cytokine expression in ovine models of MI-IUGR impairs myoblast function and diminishes muscle fiber hypertrophy, contributing to decreased total body weight in the near term fetus and postnatal lamb (Cadaret et al., 2019a; Posont et al., 2019). Along with dynamic changes in skeletal muscle growth, shifts in skeletal muscle glucose metabolism can be seen as part of the adaptive response to increased maternal inflammation, as fetal and postnatal MI-IUGR lambs demonstrate reduced glucose oxidation and impaired insulin regulation (Cadaret et al., 2019b; Posont et al., 2019). These responses to maternofetal inflammation persist postnatal and often result in asymmetric growth, impaired growth capacity, β-cell function, and metabolic capacity along with low birthweight pathologies in the sheep.
Since the MI-IUGR model is relatively new, factors outside of skeletal muscle growth and glucose metabolism have not been addressed in the literature. Hence, there is a lack of knowledge about the relationship between maternofetal inflammation and reproductive competency in progeny. While not in a ruminant species, a preliminary study in rats found that chronic maternal inflammation decreases the proportion of preantral follicles and AMH in offspring from LPS treated dams (Shalom-Paz et al., 2017). To our knowledge, no other studies exist in any species related to maternofetal inflammation, providing a gap in the literature around the role of inflammatory pathways in the programming of reproductive competency and efficiency.
Nutrient imbalance is an especially common precursor of maternal stress in animals. Rangelands of grazing livestock can have large fluctuations in quantity and quality of forage, leaving these animals predisposed to prolonged periods of undernutrition (Anderson, 1993; Bohnert and Stephenson, 2016). Models of maternal nutrient restriction have not consistently indicated placental stunting; although, the direct reduction in maternal nutrient status results in reduced placental uptake and decreased blood flow (Vonnahme, 2012). As such, fetal growth restriction may not always be evident, but adaptations to this insult can be seen at various gestational timepoints (Painter et al., 2005), providing greater insight to the tissue specific adaptive fetal mechanisms. Undernutrition during early gestation is more subdued compared to mid-late gestation, especially if animals are realimented by late gestation, and any growth deficits can likely be overcome by proper nutrition after birth (reviewed by Kenyon and Blair, 2014; Bell and Greenwood, 2016). Since it is considered relatively easy to implement this model, considerable research has focused on both the fetal and postnatal consequences of developmental programming due to maternal undernutrition. To mimic low forage quality, gestating animals will often be fed a diet consisting of ~50–70% of their nutritional requirements (Vonnahme et al., 2003; Ford et al., 2007; Martin et al., 2007; Beede et al., 2019) for differing lengths of time, inducing a series of fetal developmental adaptations varying in severity based on the degree of malnutrition.
Multiple studies using maternal undernutrition have shown programmed growth and development of the fetus in utero and postnatal, along with alterations to normal placental development (Vonnahme et al., 2007; Funston et al., 2010; Vonnahme, 2012), sometimes paired with reduced placental weight (Heasman et al., 1999). When ewes were fed a diet composed of 50% National Research Council (1985) requirements from days 28 to 78 of gestation, fetal weight was reduced by ~7.5% (Vonnahme et al., 2003). A similar study implemented the same diet restrictions and reported a decrease in birth weights, and significant catch-up growth with nutrient restricted (NR) lambs weighing more at 4 months of age and at slaughter (280 days) than control lambs (Ford et al., 2007). Compensatory weight gain may be attributed to increased plasma leptin concentrations that often favor fat deposition over muscle growth, as indicated by increased backfat thickness (Ford et al., 2007). Like other models, undernutrition is associated with hypoinsulinemia, hypoglycemia, and increased cortisol that promotes the development of glucose intolerance and insulin resistance (Ford et al., 2007; Cripps et al., 2008). Vulnerability of skeletal muscle to changes in nutrient availability additionally contributes to changes in body composition (Funston et al., 2010). Zhu et al. (2006) found damage to skeletal muscle development, evident by reductions in skeletal muscle mass, muscle fiber number, and Type IIa oxidative fibers. This coincided with reductions in GLUT 4 receptors in lambs slaughtered after 120 days of age, which indicates alterations to insulin sensitivity and glucose utilization in skeletal muscle (Zhu et al., 2006). Metabolic changes, reductions in loin muscle area, and increased fat mass (Lemley et al., 2012) of young sheep and cattle can persist throughout weaning and at slaughter compromising the weight and quality of carcasses (Larson et al., 2009). One model in dairy heifers, while not direct nutrient restriction, found that heifers born from dams lactating during gestation showed decreased milk production, survivability, and metabolic efficiency compared to heifers born to dams that were not lactating (Gonzalez-Recio et al., 2012) likely due to the competition of nutrients between the fetus and milk production.
Growth and metabolic adaptations occur concurrently to reproductive changes in NR offspring (reviewed by Chavatte-Palmer et al., 2014). Early gestational nutrient restriction in cattle followed by protein supplementation reduced healthy antral follicle count, prepubertal follicle size, and primordial follicle density at nearly 2 years of age (Sullivan et al., 2009). Similarly, early gestational nutrient restriction in cattle results in reductions in AMH concentrations along with diminished ovarian reserve (Mossa et al., 2013). In ewes, maternal nutrient restriction prior to or during folliculogenesis results in delayed ovarian reserve development (Rae et al., 2001). Other studies in ewes have exhibited impaired pituitary function in nutrient restricted offspring, influencing endocrine regulation of cellular proliferation, and reproductive function (Kotsampasi et al., 2009b; Long et al., 2021). Additionally, nutrient restriction in the first 95 days of pregnancy in ewes was shown to reduce ovulation rate in female progeny, independent of growth restriction or endocrine dysregulation (Rae et al., 2002) impacting fertility of adult female offspring born from nutrient restricted dams.
In male ruminant offspring, gestational nutrient restriction results in declines in both the number of Sertoli cells and the diameter of seminiferous tubules without changes in hypothalamic-pituitary-gonadal (HPG) regulation or testis weight (Kotsampasi et al., 2009a; Martín et al., 2012). Fortunately, age of puberty in both sexes appears largely uninfluenced by maternal undernutrition (Rae et al., 2002; Sullivan et al., 2009). However, as gonadal development and growth appears to be vulnerable to gestational nutrient restriction, more research is needed to evaluate if these transient changes impact lifelong reproduction, as reproductive efficiency is a major driver of ranch productivity and profitability.
Another popular model for inducing adaptive fetal programming involves the over feeding of adolescent ewes. When over nourished, the adolescent body will preferentially allocate nutrients toward maternal growth, compromising fetal growth and development (Anthony et al., 2003). To achieve maternal obesity, many models supply diets ranging anywhere from 140 to 200% of the NRC requirements at varying stages of pregnancy (Swanson et al., 2008; Tong et al., 2009; Carr et al., 2012). One of the major limitations of this model is maternal age, as mature animals vary in outcomes between fetal growth restriction or overgrowth, making growth impacts difficult to determine (Tong et al., 2009). Fortunately, the model still provides insight into fetal programming caused by maternal obesity which can be seen in ruminant animals.
Similar to the previous models of fetal programming, the severity of insult varies based on the timing and duration of the insult. Wallace et al. (1999) discovered that early dietary interventions provided opportunity to recover fetal growth, but those insults persisting through later gestational stages resulted in permanent adaptations. It was also determined that reductions of maternal dietary intake from high to moderate throughout mid-gestation enhanced fetal growth, while increased dietary intake at the same time leads to reductions in both placental and fetal growth (Wallace et al., 1999). Continued overnourishment prompts fetal hypoxia and hypoglycemia due to impaired uterine arterial and umbilical venous blood flow (Wallace et al., 1999, 2002), reducing placental and fetal mass by ~45% (Wallace et al., 2002; Wallace, 2019). By mid-gestation, growth restricted fetuses also experience low insulin and IGF-1 concentrations and high lactate concentrations (Wallace et al., 2003), along with a greater fat deposition (Matsuzaki et al., 2004). On average, over nourished ewes with reduced placental mass experience a shorter gestational length and are at greater risk for abortion or stillbirth (Wallace, 2019). Fetuses that survive gestation and parturition after maternal overnourishment exhibit asymmetric growth, along with an increase in weight of major endocrine organs such as the pancreas and pituitary gland (Wallace, 2019). Offspring with moderately reduced birthweight often experience rapid catch-up growth, reaching average control size by weaning (Wallace et al., 2010, 2012), while those with severely reduced birth weights remain smaller through mid-adulthood (Wallace et al., 2018). Maternal overnutrition, while seemingly counterintuitive, decreases the quality and quantity of colostrum, specifically immunoglobulin G (IgG), causing a lack of passive immunity and further contributing to decreased survivability (Swanson et al., 2008). Along with changes to postnatal body composition, these offspring tend to have altered metabolic phenotypes including altered glucose metabolism and glucose intolerance (Wallace et al., 2012, 2014) throughout all life stages leading to an obese phenotype, particularly in females (Wallace et al., 2018). The obese phenotypes, as would be expected, result in greater adiposity and less muscling leading to decreased carcass merit.
Subsequent female offspring fertility is affected more by maternal obesity than males, evidenced by diminished ovarian reserve and development, with major reductions in primordial follicle number in fetuses from obese ewes (Da Silva et al., 2002, 2003). Reduced ovarian reserve may be attributed to disruption of meiotic germ cell activity along with altered estrogen and inhibin feedback from the fetal ovaries and placenta (Da Silva et al., 2002). Fortunately, female sheep reach puberty at similar ages to controls and exhibit average estrous cyclicity during the first breeding season (Da Silva et al., 2001). Contrarily, male reproduction is un-influenced when determined by number of Sertoli cells, seminiferous tubules, or pituitary gonadotroph expression in offspring of over nourished dams compared to their control counterparts (Da Silva et al., 2003). However, male lambs from overnourished dams were slower growing, had delayed age of puberty, smaller testicular volume, and reduced testosterone, which may influence sperm quality and quantity and reduce fertility (Da Silva et al., 2001; Wallace, 2019). Overall, this model has shown that excess maternal nutrition influences male and female reproductive capacity in some regard, and that these effects are evident with and without growth restriction.
As part of a the stress response to nutrient challenge, circulating cortisol concentrations increase in the dam and fetus (Phillips et al., 1998; Roussel et al., 2004). Increased cortisol concentrations are believed to contribute to rapid maturation of organ systems, which makes late-gestation administration of synthetic glucocorticoids a common clinical practice to improve neonatal outcomes in instances of pre-term labor in humans (Seckl, 2001; Kapoor et al., 2008). However, recent studies have begun to indicate a correlation between excessive increases in maternofetal cortisol concentrations and a neonate phenotype similar to previous models of adaptive fetal programming (Seckl, 2001; Long et al., 2012), such as reduced birthweight, aberrant metabolism, and poor body composition.
Studies administering synthetic glucocorticoids in the early third trimester have revealed associations between fetal exposure to glucocorticoids and reduced birth weight, metabolic dysfunction, and endocrine alterations (Long et al., 2012, 2013a,b). Under normal circumstances, passage of maternal glucocorticoids to the fetus is minimal due to the presence of the placental enzyme 11β- hydroxysteroid dehydrogenase (11β-HSD) type 2, catalyzing the conversion of maternal cortisol to ketone products, protecting the fetus from excess glucocorticoid exposure (Seckl et al., 1995; Kapoor et al., 2008). Synthetic glucocorticoids however cannot be metabolized by 11β-HSD 2, consequently allowing placental transfer and increasing fetal glucocorticoid exposure (Kapoor et al., 2008). Along with a significant decrease in birthweight, ovine models have indicated alterations in function of the fetal and postnatal hypothalamic-pituitary-adrenal (HPA) axis in response to elevated glucocorticoids (Sloboda et al., 2002, 2007; Long et al., 2013a). Fetal sheep with repeated exposure to glucocorticoids throughout gestation have shown increased circulating adrenocorticotropic hormone (ACTH; Long et al., 2013a) and cortisol concentrations through increased pituitary glucocorticoid receptors (Sloboda et al., 2000). Upon further investigation, it was observed that offspring exposed to excess glucocorticoids through maternal injection exhibit greater adrenal sensitivity in early life (until about 1 year of age) (Sloboda et al., 2007), but this decreases with age. Glucocorticoids also program hormones that regulate appetite and glucose metabolism in offspring. Lambs from ewes administered dexamethasone during the last third of gestation were absent of a postnatal leptin surge, possibly due to antagonistic elevated cortisol observed concurrently (Long et al., 2013b). This was paired with increased appetite that resulted in weight gain in favor of adipose deposition during an ad libitum feeding trial (Long et al., 2013b). Additionally, these animals were hyperglycemic and exhibited β-cell dysfunction when put through a glucose tolerance test (Long et al., 2012, 2013a) which is a finding in other models of developmental programming as well.
Glucocorticoids have the ability to act upon various tissue types including reproductive tissue via glucocorticoid receptors (Zambrano et al., 2014). Similar to the endocrine changes seen in the HPA axis, it is believed that glucocorticoids can also program changes to hormonal function of the HPG axis; however, most of these studies have been performed in rodents (Zambrano et al., 2014). Ovine studies have shown alterations to morphologic development of testes after prenatal glucocorticoid exposure (Pedrana et al., 2008), contributing to possible programming of reproductive development. Studies in female rats exposed to glucocorticoids prenatal have shown a decrease in follicle number (Ristic et al., 2008), while studies of human ovaries exposed to glucocorticoids exhibit a decrease in number of germ cells along with increased rates of apoptosis (Poulain et al., 2012). As increased glucocorticoids can be endogenous from maternofetal stress, induced exogenously as a treatment for impending pre-term birth, or a method of labor induction, these data encourage further investigation of the long-term impacts and potential methods to mediate them.
There is compelling human and rodent data to suggest the outcomes of an adverse in utero environment, persist into subsequent generations in what is described as intergenerational or transgenerational programming (reviewed by Aiken and Ozanne, 2014). Intergenerational programming refers to conditions in one generation that impact the development, growth, metabolism, and health in future generations, without secondary insult. It is important to note that since an adverse in utero environment impacts a fetus and the developing germ cells within the fetus, alterations must be sustained into the F3 generation and beyond to be considered transgenerational (Khatib, 2021). While evidence of intergenerational programming is available, the mechanism that allows transmission is poorly understood. Current hypotheses and areas of investigation include epigenetic mechanisms passed through the germline, programmed maternal physiological mechanisms, and persistent environmental influences; however, the answer is likely a combination of multiple mechanisms (Figure 1; Drake and Walker, 2004). Most of the transgenerational data has come from observational human studies or rodents, but, in recent years some intergenerational studies have extended to livestock species to characterize the phenotypic changes that occur between generations. Studies investigating generational impacts of developmental programming in ruminant livestock are limited (Table 1), in this section we recapitulate current findings that hold relevance to animal agriculture and may serve as areas to target for management practices that mediate multigenerational programming.
Figure 1. Current proposed hypotheses of the cascade of intergenerational programming in ruminant livestock. Initial maternofetal stress results in developmental programming in the first generation. While mechanisms are not yet clear, similar phenotypes are propagated into subsequent generations.
Environmental perturbations can change plane of nutrition rapidly for grazing livestock, making nutrient availability perhaps the most common stress livestock can encounter. Studies completed at the University of Wyoming using groups of sheep from genetically similar backgrounds but vastly different production systems (well-nourished university housed sheep vs. nutrient restricted “nomadic” sheep) provide perhaps the only evidence of transgenerational programming in ruminants over ~ 5 generations (Vonnahme et al., 2006). When both groups were subjected to a 50% nutrient restriction, nomadic ewes outperformed university ewes with maintenance of ideal BCS for a longer period of time, heavier fetuses, and no difference between fetal measurements. The ability of nomadic ewes to maintain normal fetal growth is credited to placentome conversion that maintains fetal nutrient delivery, suggesting adaptive programming to nutrient restriction that has been carried over generations (Vonnahme et al., 2006; Jobgen et al., 2008). Further investigation is needed to understand how many generations it takes for these adaptations to occur as nutrient restriction in the first generation generally exhibits poor fetal outcomes, as described in previous sections of this review. Indeed, other studies investigating impacts of granddam nutrition in early gestation found varying results in daughters and granddaughters lactation performance (Van Der Linden et al., 2009), birth weight (Kenyon et al., 2014), weaning weights (Paten et al., 2013), organ morphometrics (Martín et al., 2012), and reproductive performance (Blair et al., 2010) depending on timing and duration of insult. It is worth noting that in these studies ewes were nutrient restricted in early pregnancy then realimented for the remainder of gestation. Further research is needed to understand the impacts of restriction in mid-late gestation across generations.
Intergenerational programming has been observed in models of overnourished sheep as well, extending to the F2 generations. Sheep fed 150% of NRC requirements during gestation had daughters and granddaughters with elevated cortisol concentrations, hyperglycemia, insulin resistance, and increased adiposity even when provided a normal diet (Shasa et al., 2015). Follow-up studies found these effects appear to be sex specific as male lambs from overfed granddams had greater body mass compared to female lambs or controls. Furthermore, females from these overfed granddams had dysregulated insulin:glucose dynamics compared to male lambs or controls (Pankey et al., 2017) and these effects may carry into the F3 generation (Pankey et al., 2021). Alternatively, Van Der Linden et al. (2010) found that heavier dams increased lamb birth weight but did not impact female offspring reproductive performance. However, granddams fed to maintenance had granddaughters with heavier birth and weaning weights compared to granddams fed ad libitum regardless of dam bodyweight.
Pregnant ewes administered synthetic glucocorticoids toward the end of gestation have shown similar intergenerational outcomes. Lambs from treated ewes had reduced birthweight, were structurally smaller than their control counterparts, and had altered glucose utilization and HPA axis responsiveness (Long et al., 2012, 2013a). These findings carried over into the F2 progeny showing tissue specific programming in an untreated population. Focusing on the F2 generation, Long et al. (2013b) found that F2 lambs did not experience the neonatal leptin peak and had increased cortisol concentrations which were paired with increased appetite, body weight gain in favor of fat deposition, and impaired insulin response resulting from glucocorticoid treatment of their granddams.
The dairy industry has seen evidence of intergenerational programming in response to heat stress (Ouellet et al., 2021). Indeed, in a retrospective study, cows exposed to heat stress in late gestation had daughters that left the herd earlier, had reduced initial milk yield in their first three lactations, and had differences in milk components. Effects were also seen in the granddaughters of these cows as they displayed delayed age at first AI, reduced initial milk yield in their first three lactations, and differences in components of the milk (Laporta et al., 2020). Studies in Israel also found that heat stress during mid-gestation for F0 cows had negative effects out to the F3 generation for traits associated with production and calving (Weller et al., 2021). It is believed that this was possible due to preservation of epigenetic modification during the F1 progeny development (Klosin and Lehner, 2016; Weller et al., 2021), however, further research is warranted.
Animal models of adaptive fetal programming have become increasingly useful to understand the pathophysiologic and mechanistic changes that result in adaptive phenotypes. The bulk of this research within livestock focuses largely on the fetal adaptations, while the postnatal life of these offspring remains less understood. Future research to improve the wellbeing and productivity of food animals requires in depth evaluation of the long-term effects on production agriculture, including evaluation of growth patterns, efficiency, longevity, reproductive capabilities, and carcass traits. While maternal performance is a major driver of herd productivity and profitability there seems to be a general lack of information, outside direct maternal nutrition, on programming influences of reproductive efficiency in the first and subsequent generations. Current data begins to illustrate intergenerational programming events in ruminant livestock stemming from various maternal stressors. As these studies are limited, more research is necessary to improve understanding of how various environments and timepoints impact lifelong performance. Further, larger studies that provide knowledge better characterizing the relevance and extent of the impact are warranted, including following animals into the F3 generation and beyond. Investigations into current hypotheses of heritable phenotypes that increase understanding of environmental, epigenetic or programmed changes in subsequent generations are needed and may inform production practices. Development of expected outcomes based on environmental interactions could aid in identification of compromised animals faster and better interventional management.
AV and CC contributed to drafting and revision of manuscript. All authors contributed to the article and approved the submitted version.
This work was supported in part by the Colorado State University Agricultural Experiment Station.
The authors declare that the research was conducted in the absence of any commercial or financial relationships that could be construed as a potential conflict of interest.
All claims expressed in this article are solely those of the authors and do not necessarily represent those of their affiliated organizations, or those of the publisher, the editors and the reviewers. Any product that may be evaluated in this article, or claim that may be made by its manufacturer, is not guaranteed or endorsed by the publisher.
Ahmed, B. M. S., Younas, U., Asar, T. O., Montiero, A. P. A., Hayen, M. J., Tao, S., et al. (2021). Maternal heat stress reduces body and organ growth in calves: relationship to immune status. JDS Comm. 5, 295–299. doi: 10.3168/jdsc.2021-0098
Aiken, C. E., and Ozanne, S. E. (2014). Transgenerational developmental programming. Hum. Reprod. Update 20, 63–75. doi: 10.1093/humupd/dmt043
Akbarinejad, V., Gharagozlou, F., and Vojgani, M. (2017). Temporal effect of maternal heat stress during gestation on the fertility and anti-Müllerian hormone concentration of offspring in bovine. Theriogenology 99, 69–78. doi: 10.1016/j.theriogenology.2017.05.018
Alisi, A., Panera, N., Agostoni, C., and Nobili, V. (2011). Intrauterine growth retardation and nonalcoholic fatty liver disease in children. Int. J. Endocrinol. 2011, 269853. doi: 10.1155/2011/269853
Alves, J. R. A., de Andrade, T. A. A., de Medeiros Assis, D., Gurjão, T. A., de Melo, L. R. B., and de Souza, B. B. (2020). Productive and reproductive performance, behavior and physiology of cattle under heat stress conditions. J. Anim. Behav. Biometeorol. 5, 91–96. doi: 10.31893/2318-1265jabb.v5n3p91-96
Anderson, E. W. (1993). Prescription grazing to enhance rangeland watersheds. Rangelands Arch. 15, 31–34.
Anthony, R. V., Scheaffer, A. N., Wright, C. D., and Regnault, T. R. (2003). Ruminant models of prenatal growth restriction. Reprod. Suppl. 61, 183–194. Available online at: https://www.biosciproceedings.org/bp/0005/pdf/bp0005rdr14.pdf
Barry, J. S., Davidsen, M. L., Limesand, S. W., Galan, H. L., Friedman, J. E., Regnault, T. R., et al. (2006). Developmental changes in ovine myocardial glucose transporters and insulin signaling following hyperthermia-induced intrauterine fetal growth restriction. Exp. Biol. Med. 231, 566–575. doi: 10.1177/153537020623100511
Bartelds, B., Knoester, H., Smid, G. B., Takens, J., Visser, G. H., Penninga, L., et al. (2000). Perinatal changes in myocardial metabolism in lambs. Circulation 102, 926–931. doi: 10.1161/01.cir.102.8.926
Beede, K. A., Limesand, S. W., Petersen, J. L., and Yates, D. T. (2019). Real supermodels wear wool: summarizing the impact of the pregnant sheep as an animal model for adaptive fetal programming. Animal Front. 9, 34–43. doi: 10.1093/af/vfz018
Bell, A., Mcbride, B., Slepetis, R., Early, R., and Currie, W. (1989). Chronic heat stress and prenatal development in sheep: I. Conceptus growth and maternal plasma hormones and metabolites. J. Anim. Sci. 67, 3289–3299. doi: 10.2527/jas1989.67123289x
Bell, A. W., and Greenwood, P. L. (2016). Prenatal origins of postnatal variation in growth, development and productivity of ruminants. Anim. Prod. Sci. 56, 1217–1232. doi: 10.1071/AN15408
Bertucci, M. C., Loose, J. M., Wallace, E. M., Jenkin, G., and Miller, S. L. (2011). Anti-inflammatory therapy in an ovine model of fetal hypoxia induced by single umbilical artery ligation. Reprod. Fertil. Dev. 23, 346–352. doi: 10.1071/RD10110
Blair, H. T., Jenkinson, C. M., Peterson, S. W., Kenyon, P. R., Van Der Linden, D. S., Davenport, L. C., et al. (2010). Dam and granddam feeding during pregnancy in sheep affects milk supply in offspring and reproductive performance in grand-offspring. J. Anim. Sci. 88, E40–E50. doi: 10.2527/jas.2009-2523
Bohnert, D. W., and Stephenson, M. B. (2016). Supplementation and sustainable grazing systems. J. An. Sci. 94, 15–25. doi: 10.2527/jas.2016-0520
Brown, L. D., Rozance, P. J., Bruce, J. L., Friedman, J. E., Hay, W. W. Jr., and Wesolowski, S.R. (2015). Limited capacity for glucose oxidation in fetal sheep with intrauterine growth restriction. Am. J. Physiol. Regul. Integr. Comp. Physiol. 309, R920–928. doi: 10.1152/ajpregu.00197.2015
Cadaret, C. N., Merrick, E. M., Barnes, T. L., Beede, K. A., Posont, R. J., Petersen, J. L., et al. (2019a). Sustained maternal inflammation during the early third-trimester yields intrauterine growth restriction, impaired skeletal muscle glucose metabolism, and diminished β-cell function in fetal sheep. J. Anim. Sci. 97, 4822–4833. doi: 10.1093/jas/skz321
Cadaret, C. N., Posont, R. J., Beede, K. A., Riley, H. E., Loy, J. D., and Yates, D. T. (2019b). Maternal inflammation at midgestation impairs subsequent fetal myoblast function and skeletal muscle growth in rats, resulting in intrauterine growth restriction at term. Transl. Anim. Sci. 3, 867–876. doi: 10.1093/tas/txz037
Carr, D. J., Aitken, R. P., Milne, J. S., David, A. L., and Wallace, J. M. (2012). Fetoplacental biometry and umbilical artery Doppler velocimetry in the overnourished adolescent model of fetal growth restriction. Am. J. Obstet. Gynecol. 207, 141.e146–e115.doi: 10.1016/j.ajog.2012.05.008
Chavatte-Palmer, P., Dupont, C., Debus, N., and Camous, S. (2014). Nutritional programming and the reproductive function of the offspring. Anim. Prod. Sci. 54, 1166–1176. doi: 10.1071/AN14470
Chen, X., Fahy, A. L., Green, A. S., Anderson, M. J., Rhoads, R. P., and Limesand, S. W. (2010). β2-Adrenergic receptor desensitization in perirenal adipose tissue in fetuses and lambs with placental insufficiency-induced intrauterine growth restriction. J. Physiol. 588, 3539–3549. doi: 10.1113/jphysiol.2010.192310
Cox, P., and Marton, T. (2009). Pathological assessment of intrauterine growth restriction. Best Pract. Res. Clin. Obstet. Gynaecol. 23, 751–764. doi: 10.1016/j.bpobgyn.2009.06.006
Cripps, R. L., Green, L. R., Thompson, J., Martin-Gronert, M. S., Monk, M., Sheldon, I. M., et al. (2008). The effect of maternal body condition score before and during pregnancy on the glucose tolerance of adult sheep offspring. Reprod. Sci. 15, 448–456. doi: 10.1177/1933719107312161
Da Silva, P., Aitken, R., Rhind, S., Racey, P., and Wallace, J. (2003). Effect of maternal overnutrition during pregnancy on pituitary gonadotrophin gene expression and gonadal morphology in female and male foetal sheep at day 103 of gestation. Placenta 24, 248–257. doi: 10.1053/plac.2002.0897
Da Silva, P., Aitken, R. P., Rhind, S. M., Racey, P. A., and Wallace, J. M. (2001). Influence of placentally mediated fetal growth restriction on the onset of puberty in male and female lambs. Reproduction 122, 375–383. doi: 10.1530/rep.0.1220375
Da Silva, P., Aitken, R. P., Rhind, S. M., Racey, P. A., and Wallace, J. M. (2002). Impact of maternal nutrition during pregnancy on pituitary gonadotrophin gene expression and ovarian development in growth-restricted and normally grown late gestation sheep fetuses. Reproduction 123, 769–777. doi: 10.1530/rep.0.1230769
Dado-Senn, B., Vega Acosta, L., Torres Rivera, M., Field, S. L., Marrero, M. G., Davidson, B. D., et al. (2020). Pre- and postnatal heat stress abatement affects dairy calf thermoregulation and performance. J. Dairy Sci. 103, 4822–4837. doi: 10.3168/jds.2019-17926
Dahl, G. E., Skibiel, A. L., and Laporta, J. (2019). In utero heat stress programs reduced performance and health in calves. Vet. Clin. North Am. Food Anim. Pract. 35, 343–353. doi: 10.1016/j.cvfa.2019.02.005
Davidson, B. D., Dad-Senn, B., Ouellet, V., and Laporta, J. (2021). Effect of late-gestation heat stress in nullparous heifers on postanatal growth, passive transfer of immunoglobulin G, and thermoregulation of their calves. JDS Comm. 2, 165–169. doi: 10.3168/jdsc.2020-0069
De Blasio, M. J., Gatford, K. L., Robinson, J. S., and Owens, J. A. (2007). Placental restriction of fetal growth reduces size at birth and alters postnatal growth, feeding activity, and adiposity in the young lamb. Am. J. Physiol. Regul. Integr. Comp. Physiol. 292, R875–886. doi: 10.1152/ajpregu.00430.2006
Drake, A. J., and Walker, B. R. (2004). The intergenerational effects of fetal programming: non-genomic mechanisms for the inheritance of low birth weight and cardiovascular risk. J. Endocrinol. 180, 1–16. doi: 10.1677/joe.0.1800001
Dwyer, C. M., Conington, J., Corbiere, F., Holmoy, I. H., Muri, K., Nowak, R., et al. (2016). Invited review: improving neonatal survival in small ruminants: science into practice. Animal. 10, 449–459. doi: 10.1017/S1751731115001974
Flinn, T., Kleemann, D. O., Swinbourne, A. M., Kelly, J. M., Weaver, A. C., Walker, S. K., et al. (2020). Neonatal lamb mortality: major risk factor and the potential ameliorative role of melatonin. J. Animal Sci. Biotechnol. 11, 107. doi: 10.1186/s40104-020-00510-w
Ford, S. P., Hess, B. W., Schwope, M. M., Nijland, M. J., Gilbert, J. S., Vonnahme, K. A., et al. (2007). Maternal undernutrition during early to mid-gestation in the ewe results in altered growth, adiposity, and glucose tolerance in male offspring. J. Anim. Sci. 85, 1285–1294. doi: 10.2527/jas.2005-624
Frost, R. A., Lang, C. H., and Gelato, M. C. (1997). Transient exposure of human myoblasts to tumor necrosis factor-alpha inhibits serum and insulin-like growth factor-I stimulated protein synthesis. Endocrinology 138, 4153–4159. doi: 10.1210/endo.138.10.5450
Funston, R. N., Larson, D. M., and Vonnahme, K. A. (2010). Effects of maternal nutrition on conceptus growth and offspring performance: implications for beef cattle production. J. Anim. Sci. 88, E205–215. doi: 10.2527/jas.2009-2351
Galan, H. L., Hussey, M. J., Barbera, A., Ferrazzi, E., Chung, M., Hobbins, J. C., et al. (1999). Relationship of fetal growth to duration of heat stress in an ovine model of placental insufficiency. Am. J. Obstet. Gynecol. 180, 1278–1282. doi: 10.1016/s0002-9378(99)70629-0
Gibbs, R. L., Cadaret, C. N., Swanson, R. M., Beede, K. A., Posont, R. J., Schmidt, T. B., et al. (2019). Body composition estimated by bioelectrical impedance analyses is diminished by prenatal stress in neonatal lambs and by heat stress in feedlot wethers. Transl. Anim. Sci. 3, 1691–1695. doi: 10.1093/tas/txz059
Gifford, C. A., Holland, B. P., Mills, R. L., Maxwell, C. L., Farney, J. K., Terrill, S. J., et al. (2012). Growth and Development Symposium: impacts of inflammation on cattle growth and carcass merit. J. Anim. Sci. 90, 1438–1451. doi: 10.2527/jas.2011-4846
Gluckman, P. D., and Hanson, M. A. (2004). Developmental origins of disease paradigm: a mechanistic and evolutionary perspective. Pediat. Res. 56, 311–317. doi: 10.1203/01.PDR.0000135998.08025.FB
Goldenberg, R. L., and Culhane, J. F. (2007). Low birth weight in the United States. Am. J. Clin. Nutr. 85, 584S−590S. doi: 10.1093/ajcn/85.2.584S
Gonzalez-Recio, O., Urgarte, E., and Bach, A. (2012). Trans-generational effect of maternal lactation during pregnancy: a Holstein cow model. PLoS ONE 7, e51816. doi: 10.1371/journal.pone.0051816
Gootwine, E., Spencer, T. E., and Bazer, F. W. (2007). Litter-size-dependent intrauterine growth restriction in sheep. Animal 1, 547–564. doi: 10.1017/S1751731107691897
Greenwood, P. L., and Cafe, L. M. (2007). Prenatal and pre-weaning growth and nutrition of cattle: long-term consequences for beef production. Animal 1, 1283–1296. doi: 10.1017/S175173110700050X
Hales, C. N., and Barker, D. J. (1992). Type 2 (non-insulin-dependent) diabetes mellitus: the thrifty phenotype hypothesis. Diabetologia 35, 595–601. doi: 10.1007/BF00400248
Hales, C. N., and Barker, D. J. (2001). The thrifty phenotype hypothesis. Br. Med. Bull. 60, 5–20. doi: 10.1093/bmb/60.1.5
Hansen, P. J. (2011). The immunology of early pregnancy in farm animals. Reprod. Domest. Anim. 46(Suppl. 3), 18–30. doi: 10.1111/j.1439-0531.2011.01850.x
Hay, W. W. Jr., Brown, L. D., Rozance, P. J., Wesolowski, S. R., and Limesand, S. W. (2016). Challenges in nourishing the intrauterine growth-restricted foetus - lessons learned from studies in the intrauterine growth-restricted foetal sheep. Acta Paediatr. 105, 881–889. doi: 10.1111/apa.13413
Heasman, L., Clarke, L., Stephenson, T. J., and Symonds, M. E. (1999). The influence of maternal nutrient restriction in early to mid-pregnancy on placental and fetal development in sheep. Proc. Nutr. Soc. 58, 283–288. doi: 10.1017/s0029665199000397
Jobgen, W. S., Ford, S. P., Jobgen, S. C., Feng, C. P., Hess, B. W., Nathanielsz, P. W., et al. (2008). Baggs ewes adapt to maternal undernutrition and maintain conceptus growth by maintaining fetal plasma concentrations of amino acids. J. Anim. Sci. 86, 820–826. doi: 10.2527/jas.2007-0624
Kapoor, A., Petropoulos, S., and Matthews, S. G. (2008). Fetal programming of hypothalamic-pituitary-adrenal (HPA) axis function and behavior by synthetic glucocorticoids. Brain Res. Rev. 57, 586–595. doi: 10.1016/j.brainresrev.2007.06.013
Kenyon, P. R., and Blair, H. T. (2014). Foetal programming in sheep – effects on production. Small Rum. Res. 118, 16–30. doi: 10.1016/j.smallrumres.2013.12.021
Kenyon, P. R., Corner-Thomas, R. A., Peterson, S. W., Pain, S. J., and Blair, H. T. (2014). Pregnancy nutrition does not influence lamb liveweight in developmentally programmed ewes. Anim. Prod. Sci. 54, 1465–1470. doi: 10.1071/an14217
Khatib, H. (2021). Transgenerational epigenetic inheritance in farm animals: how substantial is the evidence? Livest. Sci. 250, 104557. doi: 10.1016/j.livsci.2021.104557
Klosin, A., and Lehner, B. (2016). Mechanisms, timescales and principles of trans-generational epigenetic inheritance in animals. Curr. Opin. Genet. Dev. 36, 41–49. doi: 10.1016/j.gde.2016.04.001
Kotsampasi, B., Balaskas, C., Papadomichelakis, G., and Chadio, S. E. (2009a). Reduced Sertoli cell number and altered pituitary responsiveness in male lambs undernourished in utero. Anim. Reprod. Sci. 114, 135–147. doi: 10.1016/j.anireprosci.2008.08.017
Kotsampasi, B., Chadio, S., Papadomichelakis, G., Deligeorgis, S., Kalogiannis, D., Menegatos, I., et al. (2009b). Effects of maternal undernutrition on the hypothalamic-pituitary-gonadal axis function in female sheep offspring. Reprod. Domest. Anim. 44, 677–684. doi: 10.1111/j.1439-0531.2007.01046.x
Laporta, J., Ferreira, F. C., Ouellet, V., Dado-Senn, B., Almeida, A. K., De Vries, A., et al. (2020). Late-gestation heat stress impairs daughter and granddaughter lifetime performance. J. Dairy Sci. 203, 7555–7568. doi: 10.3168/jds.2020-18154
Larson, D. M., Martin, J. L., Adams, D. C., and Funston, R. N. (2009). Winter grazing system and supplementation during late gestation influence performance of beef cows and steer progeny. J. Anim. Sci. 87, 1147–1155. doi: 10.2527/jas.2008-1323
Lemley, C. O., Meyer, A. M., Camacho, L. E., Neville, T. L., Newman, D. J., Caton, J. S., et al. (2012). Melatonin supplementation alters uteroplacental hemodynamics and fetal development in an ovine model of intrauterine growth restriction. Am. J. Physiol. Regul. Integr. Comp. Physiol. 302, R454–467. doi: 10.1152/ajpregu.00407.2011
Leos, R. A., Anderson, M. J., Chen, X., Pugmire, J., Anderson, K. A., and Limesand, S. W. (2010). Chronic exposure to elevated norepinephrine suppresses insulin secretion in fetal sheep with placental insufficiency and intrauterine growth restriction. Am. J. Physiol. Endocrinol. Metab. 298, E770–778. doi: 10.1152/ajpendo.00494.2009
Limesand, S. W., Camacho, L. E., Kelly, A. C., and Antolic, A. T. (2018). Impact of thermal stress on placental function and fetal physiology. Anim. Reprod. 15, 886–898. doi: 10.21451/1984-3143-ar2018-0056
Limesand, S. W., Rozance, P. J., Smith, D., and Hay, W. W. Jr. (2007). Increased insulin sensitivity and maintenance of glucose utilization rates in fetal sheep with placental insufficiency and intrauterine growth restriction. Am. J. Physiol. Endocrinol. Metab. 293, E1716–1725. doi: 10.1152/ajpendo.00459.2007
Limesand, S. W., Rozance, P. J., Zerbe, G. O., Hutton, J. C., and Hay, W. W. Jr. (2006). Attenuated insulin release and storage in fetal sheep pancreatic islets with intrauterine growth restriction. Endocrinology 147, 1488–1497. doi: 10.1210/en.2005-0900
Long, J. M., Trubenbach, L. A., Hobbs, K. C., Poletti, A. E., Steinhauser, C. B., Pryor, J. H., et al. (2021). Maternal nutrient restriction in late pregnancy programs postnatal metabolism and pituitary development in beef heifers. PLoS ONE 16, e0249924. doi: 10.1371/journal.pone.0249924
Long, N. M., Ford, S. P., and Nathanielsz, P. W. (2013a). Multigenerational effects of fetal dexamethasone exposure on the hypothalamic-pituitary-adrenal axis of first- and second-generation female offspring. Am. J. Obstet. Gynecol. 208, 217.e211–218. doi: 10.1016/j.ajog.2012.12.014
Long, N. M., Shasa, D. R., Ford, S. P., and Nathanielsz, P. W. (2012). Growth and insulin dynamics in two generations of female offspring of mothers receiving a single course of synthetic glucocorticoids. Am. J. Obstet. Gynecol. 207, 203.e201–208. doi: 10.1016/j.ajog.2012.06.024
Long, N. M., Smith, D. T., Ford, S. P., and Nathanielsz, P. W. (2013b). Elevated glucocorticoids during ovine pregnancy increase appetite and produce glucose dysregulation and adiposity in their granddaughters in response to ad libitum feeding at 1 year of age. Am. J. Obstet. Gynecol. 209, 353.e351–359. doi: 10.1016/j.ajog.2013.05.051
Macko, A. R., Yates, D. T., Chen, X., Green, A. S., Kelly, A. C., Brown, L. D., et al. (2013). Elevated plasma norepinephrine inhibits insulin secretion, but adrenergic blockade reveals enhanced beta-cell responsiveness in an ovine model of placental insufficiency at 0.7 of gestation. J. Dev. Orig. Health Dis. 4, 402–410. doi: 10.1017/S2040174413000093
Martin, J. L., Vonnahme, K. A., Adams, D. C., Lardy, G. P., and Funston, R. N. (2007). Effects of dam nutrition on growth and reproductive performance of heifer calves. J. Anim. Sci. 85, 841–847. doi: 10.2527/jas.2006-337
Martín, N. P., Kenyon, P. R., Morel, P. C. H., Pain, S. J., Jenkinson, C. M. C., Hutton, P. G., et al. (2012). Ewe nutrition in early and mid- to late pregnancy has few effects on fetal development. Anim. Prod. Sci. 52. 533–539. doi: 10.1071/an11246
Matsuzaki, M., Milne, J., Aitken, R., Redmer, D., and Wallace, J. (2004). Overnourishing pregnant adolescent ewes stimulates perirenal fat deposition in their growth restricted foetuses. J. Anim. Feed Sci. 13, 519–522. doi: 10.22358/jafs/74022/2004
Monteiro, A. P. A., Tao, S., Thompson, I. M. T., and Dahl, G. E. (2016). In utero heat stress decreases calf survival and performance through the first lactation. J. Dairy Sci. 99, 8443–8450. doi: 10.3168/jds.2016-11072
Morrison, J. L. (2008). Sheep models of intrauterine growth restriction: fetal adaptations and consequences. Clin. Exp. Pharmacol. Physiol. 35, 730–743. doi: 10.1111/j.1440-1681.2008.04975.x
Mossa, F., Carter, F., Walsh, S. W., Kenny, D. A., Smith, G. W., Ireland, J. L., et al. (2013). Maternal undernutrition in cows impairs ovarian and cardiovascular systems in their offspring. Biol. Reprod. 88, 92. doi: 10.1095/biolreprod.112.107235
National Research Council (1985). Nutrient Requirements of Sheep, 6th Edn. Washington, D.C. National Academies Press.
Ouellet, V., Boucher, A., Dahl, G. E., and Laporta, J. (2021). Consequences of maternal heat stress at different stages of embryonic and fetal development in dairy cow's progeny. Animal Front. 11, 48–56. doi: 10.1093/af/vfab059
Ouellet, V., Laporta, J., and Dahl, G. E. (2020). Late gestation heat stress in dairy cows: effects on dam and daughter. Theriogenology 150,471–479. doi: 10.1016/j.theriogenology.2020.03.011
Painter, R. C., Roseboom, T. J., and Bleker, O. P. (2005). Prenatal exposure to the Dutch famine and disease in later life: an overview. Reprod. Toxicol. 20, 345–352. doi: 10.1016/j.reprotox.2005.04.005
Pankey, C. L., Odhiambo, J. F., Smith, A. M., and Ford, S. P. (2021). Effects of maternal obesity in an ovine model on metabolic outcomes in F2 adults and F3 neonates. Domest. Anim. Endocrinol. 76, 106628. doi: 10.1016/j.domaniend.2021.106628
Pankey, C. L., Walton, M. W., Odhiambo, J. F., Smith, A. M., Ghnenis, A. B., Nathanielsz, P. W., et al. (2017). Intergenerational impact of maternal overnutrition and obesity throughout pregnancy in sheep on metabolic syndrome in grandsons and granddaughters. Domest. Anim. Endocrinol. 60, 67–74. doi: 10.1016/j.domaniend.2017.04.002
Paten, A. M., Kenyon, P. R., Lopez-Villalobos, N., Peterson, S. W., Jenkinson, C. M., Pain, S. J., et al. (2013). Lactation Biology Symposium: maternal nutrition during early and mid-to-late pregnancy: Comparative effects on milk production of twin-born ewe progeny during their first lactation. J. Anim. Sci. 91, 676–684. doi: 10.2527/jas.2012-5752
Pedrana, G., Sloboda, D. M., Perez, W., Newnham, J. P., Bielli, A., and Martin, G. B. (2008). Effects of pre-natal glucocorticoids on testicular development in sheep. Anat. Histol. Embryol. 37, 352–358. doi: 10.1111/j.1439-0264.2008.00853.x
Phillips, D. I., Barker, D. J., Fall, C. H., Seckl, J. R., Whorwood, C. B., Wood, P. J., et al. (1998). Elevated plasma cortisol concentrations: a link between low birth weight and the insulin resistance syndrome? J. Clin. Endocrinol. Metab. 83, 757–760. doi: 10.1210/jcem.83.3.4634
Posont, R. J., Cadaret, C. N., Beede, K. A., Beard, J. K., Swanson, R. M., Gibbs, R. L., et al. (2019). Maternal inflammation at 0.7 gestation in ewes leads to intrauterine growth restriction and impaired glucose metabolism in offspring at 30 d of age. Transl. Anim. Sci. 3, 1673–1677. doi: 10.1093/tas/txz055
Poudel, R., Mcmillen, I. C., Dunn, S. L., Zhang, S., and Morrison, J. L. (2015). Impact of chronic hypoxemia on blood flow to the brain, heart, and adrenal gland in the late-gestation IUGR sheep fetus. Am. J. Physiol. Regul. Integr. Comp. Physiol. 308, R151–162. doi: 10.1152/ajpregu.00036.2014
Poulain, M., Frydman, N., Duquenne, C., N'tumba-Byn, T., Benachi, A., Habert, R., et al. (2012). Dexamethasone induces germ cell apoptosis in the human fetal ovary. J. Clin. Endocrinol. Metab. 97, E1890–1897. doi: 10.1210/jc.2012-1681
Rae, M. T., Kyle, C. E., Miller, D. W., Hammond, A. J., Brooks, A. N., and Rhind, S. M. (2002). The effects of undernutrition, in utero, on reproductive function in adult male and female sheep. Anim. Reprod. Sci. 72, 63–71. doi: 10.1016/s0378-4320(02)00068-4
Rae, M. T., Palassio, S., Kyle, C. E., Brooks, A. N., Lea, R. G., Miller, D. W., et al. (2001). Effect of maternal undernutrition during pregnancy on early ovarian development and subsequent follicular development in sheep fetuses. Reproduction 122, 915–922. doi: 10.1530/rep.0.1220915
Redmer, D. A., Wallace, J. M., and Reynolds, L. P. (2004). Effect of nutrient intake during pregnancy on fetal and placental growth and vascular development. Domest. Anim. Endocrinol. 27, 199–217. doi: 10.1016/j.domaniend.2004.06.006
Regnault, T. R., De Vrijer, B., Galan, H. L., Wilkening, R. B., Battaglia, F. C., and Meschia, G. (2013). Umbilical uptakes and transplacental concentration ratios of amino acids in severe fetal growth restriction. Pediatr. Res. 73, 602–611. doi: 10.1038/pr.2013.30
Reynolds, L. P., Borowicz, P. P., Caton, J. S., Vonnahme, K. A., Luther, J. S., Hammer, C. J., et al. (2010). Developmental programming: the concept, large animal models, and the key role of uteroplacental vascular development. J. Anim. Sci. 88, E61–72. doi: 10.2527/jas.2009-2359
Reynolds, L. P., and Vonnahme, K. A. (2017). Livestock as models for developmental programming. Animal Front. 7, 12–17. doi: 10.2527/af.2017-0123
Ristic, N., Nestorovic, N., Manojlovic-Stojanoski, M., Filipovic, B., Sosic-Jurjevic, B., Milosevic, V., et al. (2008). Maternal dexamethasone treatment reduces ovarian follicle number in neonatal rat offspring. J. Microsc. 232, 549–557. doi: 10.1111/j.1365-2818.2008.02117.x
Roussel, S., Hemsworth, P. H., Boissy, A., and Duvaux-Ponter, C. (2004). Effects of repeated stress during pregnancy in ewes on the behavioural and physiological responses to stressful events and birth weight of their offspring. Appl. Anim. Behav. Sci. 85, 259–276. doi: 10.1016/j.applanim.2003.11.006
Rozance, P. J., Zastoupil, L., Wesolowski, S. R., Goldstrohm, D. A., Strahan, B., Cree-Green, M., et al. (2018). Skeletal muscle protein accretion rates and hindlimb growth are reduced in late gestation intrauterine growth-restricted fetal sheep. J. Physiol. 596, 67–82. doi: 10.1113/JP275230
Seckl, J. R. (2001). Glucocorticoid programming of the fetus; adult phenotypes and molecular mechanisms. Mol. Cell. Endocrinol. 185, 61–71. doi: 10.1016/s0303-7207(01)00633-5
Seckl, J. R., Benediktsson, R., Lindsay, R. S., and Brown, R. W. (1995). Placental 11β-hydroxysteroid dehydrogenase and the programming of hypertension. J. Steroid Biochem. Molec. Biol. 55, 447–455. doi: 10.1016/0960-0760(95)00193-x
Shalom-Paz, E., Weill, S., Ginzberg, Y., Khatib, N., Anabusi, S., Klorin, G., et al. (2017). IUGR induced by maternal chronic inflammation: long-term effect on offspring's ovaries in rat model-a preliminary report. J. Endocrinol. Invest. 40, 1125–1131. doi: 10.1007/s40618-017-0681-3
Shasa, D. R., Odhiambo, J. F., Long, N. M., Tuersunjiang, N., Nathanielsz, P. W., and Ford, S. P. (2015). Multigenerational impact of maternal overnutrition/obesity in the sheep on the neonatal leptin surge in granddaughters. Int. J. Obes. 39, 695–701. doi: 10.1038/ijo.2014.190
Skibiel, A. L., Dado-Senn, B., Fabris, T. F., Dahl, G. E., and Laporta, L. (2018b). In utero exposure to thermal stress has long-term effects on mammary gland microstructure and function in dairy cattle. PLoS ONE 13, e0206046. doi: 10.1371/journal.pone.0206046
Skibiel, A. L., Penagaricano, F., Amorin, R, Ahmend, B. M., Dahl, G. E., and Laporta, J. (2018a). In utero heat stress altesr the offspring epigenome. Sci. Rep. 8, 14609. doi: 10.1038/s41598-018-32975-1
Sloboda, D. M., Moss, T. J., Gurrin, L. C., Newnham, J. P., and Challis, J. R. (2002). The effect of prenatal betamethasone administration on postnatal ovine hypothalamic-pituitary-adrenal function. J. Endocrinol. 172, 71–81. doi: 10.1677/joe.0.1720071
Sloboda, D. M., Moss, T. J., Li, S., Doherty, D., Nitsos, I., Challis, J. R., et al. (2007). Prenatal betamethasone exposure results in pituitary-adrenal hyporesponsiveness in adult sheep. Am. J. Physiol. Endocrinol. Metab. 292, E61–70. doi: 10.1152/ajpendo.00270.2006
Sloboda, D. M., Newnham, J. P., and Challis, J. R. G. (2000). Effects of repeated maternal betamethasone administration on growth and hypothalamic-pituitary-adrenal function of the ovine fetus at term. J. Endocrinol. 165, 79–91. doi: 10.1677/joe.0.1650079
Sullivan, T. M., Micke, G. C., Greer, R. M., Irving-Rodgers, H. F., Rodgers, R. J., and Perry, V. E. (2009). Dietary manipulation of Bos indicus x heifers during gestation affects the reproductive development of their heifer calves. Reprod. Fertil. Dev. 21, 773–784. doi: 10.1071/RD09004
Swanson, R. M., Tait, R. G., Galles, B. M., Duffy, E. M., Schmidt, T. B., Petersen, J. L., et al. (2020). Heat stress-induced deficits in growth, metabolic efficiency, and cardiovascular function coincided with chronic systemic inflammation and hypercatecholaminemia in ractopamine-supplemented feedlot lambs. J. Anim. Sci. 98, skaa168. doi: 10.1093/jas/skaa168
Swanson, T. J., Hammer, C. J., Luther, J. S., Carlson, D. B., Taylor, J. B., Redmer, D. A., et al. (2008). Effects of gestational plane of nutrition and selenium supplementation on mammary development and colostrum quality in pregnant ewe lambs. J. Anim. Sci. 86, 2415–2423. doi: 10.2527/jas.2008-0996
Tao, S., Monteiro, A. P., Thompson, I. M., Hayen, M. J., and Dahl, G. E. (2012). Effect of late-gestation maternal heat stress on growth and immune function of dairy calves. J. Dairy Sci. 95, 7128–7136. doi: 10.3168/jds.2012-5697
Thorn, S. R., Regnault, T. R., Brown, L. D., Rozance, P. J., Keng, J., Roper, M., et al. (2009). Intrauterine growth restriction increases fetal hepatic gluconeogenic capacity and reduces messenger ribonucleic acid translation initiation and nutrient sensing in fetal liver and skeletal muscle. Endocrinology 150, 3021–3030. doi: 10.1210/en.2008-1789
Thureen, P. J., Trembler, K. A., Meschia, G., Makowski, E. L., and Wilkening, R. B. (1992). Placental glucose transport in heat-induced fetal growth retardation. Am. J. Physiol. 263, R578–585. doi: 10.1152/ajpregu.1992.263.3.R578
Tong, J. F., Yan, X., Zhu, M. J., Ford, S. P., Nathanielsz, P. W., and Du, M. (2009). Maternal obesity downregulates myogenesis and beta-catenin signaling in fetal skeletal muscle. Am. J. Physiol. Endocrinol. Metab. 296, E917–924. doi: 10.1152/ajpendo.90924.2008
Van Der Linden, D. S., Kenyon, P. R., Blair, H. T., Lopez-Villalobos, N., Jenkinson, C. M., Peterson, S. W., et al. (2009). Effects of ewe size and nutrition on fetal mammary gland development and lactational performance of offspring at their first lactation. J. Anim. Sci. 87, 3944–3954. doi: 10.2527/jas.2009-2125
Van Der Linden, D. S., Kenyon, P. R., Lopez-Villalobos, N., Jenkinson, C. M. C., Peterson, S. W., and Blair, H. T. (2010). Effects of ewe size and nutrition during pregnancy on performance of 2-year-old female offspring. J. Agric. Sci. 148, 465–475. doi: 10.1017/s0021859610000274
Van Eetvelde, M., Kama, M. M., Hostens, M., Vandaele, L., Fiems, L. O., and Opsomer, G. (2016). Evidence for placental compensation in cattle. Animal. 10, 1342–1350. doi: 10.1017/S1751731116000318
Van Wettere, W., Kind, K. L., Gatford, K. L., Swinbourne, A. M., Leu, S. T., Hayman, P. T., et al. (2021). Review of the impact of heat stress on reproductive performance of sheep. J. Anim. Sci. Biotechnol. 12, 26. doi: 10.1186/s40104-020-00537-z
Vonnahme, K. A. (2012). How the maternal environment impacts fetal and placental development: implications for livestock production. Anim. Reprod. 9, 789–797.
Vonnahme, K. A., Hess, B. W., Hansen, T. R., Mccormick, R. J., Rule, D. C., Moss, G. E., et al. (2003). Maternal undernutrition from early- to mid-gestation leads to growth retardation, cardiac ventricular hypertrophy, and increased liver weight in the fetal sheep. Biol. Reprod. 69, 133–140. doi: 10.1095/biolreprod.102.012120
Vonnahme, K. A., Hess, B. W., Nijland, M. J., Nathanielsz, P. W., and Ford, S. P. (2006). Placentomal differentiation may compensate for maternal nutrient restriction in ewes adapted to harsh range conditions. J. Anim. Sci. 84, 3451–3459. doi: 10.2527/jas.2006-132
Vonnahme, K. A., Zhu, M. J., Borowicz, P. P., Geary, T. W., Hess, B. W., Reynolds, L. P., et al. (2007). Effect of early gestational undernutrition on angiogenic factor expression and vascularity in the bovine placentome. J. Anim. Sci. 85, 2464–2472. doi: 10.2527/jas.2006-805
Wallace, J. M. (2019). Competition for nutrients in pregnant adolescents: consequences for maternal, conceptus and offspring endocrine systems. J. Endocrinol. 242, T1–T19. doi: 10.1530/JOE-18-0670
Wallace, J. M., Bourke, D. A., Aitken, R. P., and Cruickshank, M. A. (1999). Switching maternal dietary intake at the end of the first trimester has profound effects on placental development and fetal growth in adolescent ewes carrying singleton fetuses. Biol. Reprod. 61, 101–110. doi: 10.1095/biolreprod61.1.101
Wallace, J. M., Bourke, D. A., Aitken, R. P., Leitch, N., and Hay, W. W. Jr. (2002). Blood flows and nutrient uptakes in growth-restricted pregnancies induced by overnourishing adolescent sheep. Am. J. Physiol. Regul. Integr. Comp. Physiol. 282, R1027–1036. doi: 10.1152/ajpregu.00465.2001
Wallace, J. M., Bourke, D. A., Aitken, R. P., Milne, J. S., and Hay, W. W. Jr. (2003). Placental glucose transport in growth-restricted pregnancies induced by overnourishing adolescent sheep. J. Physiol. 547, 85–94. doi: 10.1113/jphysiol.2002.023333
Wallace, J. M., Milne, J. S., Adam, C. L., and Aitken, R. P. (2012). Adverse metabolic phenotype in low-birth-weight lambs and its modification by postnatal nutrition. Br. J. Nutr. 107, 510–522. doi: 10.1017/S0007114511003175
Wallace, J. M., Milne, J. S., and Aitken, R. P. (2010). Effect of weight and adiposity at conception and wide variations in gestational dietary intake on pregnancy outcome and early postnatal performance in young adolescent sheep. Biol. Reprod. 82, 320–330. doi: 10.1095/biolreprod.109.080069
Wallace, J. M., Milne, J. S., Aitken, R. P., and Adam, C. L. (2014). Impact of embryo donor adiposity, birthweight and gender on early postnatal growth, glucose metabolism and body composition in the young lamb. Reprod. Fertil. Dev 26, 665–681. doi: 10.1071/RD13090
Wallace, J. M., Milne, J. S., Aitken, R. P., Horgan, G. W., and Adam, C. L. (2018). Ovine prenatal growth restriction impacts glucose metabolism and body composition throughout life in both sexes. Reproduction 156, 103–119. doi: 10.1530/REP-18-0048
Wallace, J. M., Regnault, T. R., Limesand, S. W., Hay, W. W. Jr., and Anthony, R. V. (2005). Investigating the causes of low birth weight in contrasting ovine paradigms. J. Physiol. 565, 19–26. doi: 10.1113/jphysiol.2004.082032
Weller, J. I., Ezra, E., and Gershoni, M. (2021). Broad phenotypic impact of the effects of transgenerational heat stress in dairy cattle: a study of four consecutive generations. Genet. Sel. Evol. 53. doi: 10.1186/s12711-021-00666-7
Wu, G., Bazer, F., Cudd, T. A., Meininger, C. J., and Thomas, E. S. (2004). Recent advances in nutritional sciences-maternal nutrition and fetal development. J. Nutr. 139, 2169–2172. Available online at: http://jn.nutrition.org/content/134/9/2169.full.pdf
Wu, G., Bazer, F. W., Wallace, J. M., and Spencer, T. E. (2006). Board-invited review: intrauterine growth retardation: implications for the animal sciences. J. Anim. Sci. 84, 2316–2337. doi: 10.2527/jas.2006-156
Yates, D. T., Cadaret, C. N., Beede, K. A., Riley, H. E., Macko, A. R., Anderson, M. J., et al. (2016). Intrauterine growth-restricted sheep fetuses exhibit smaller hindlimb muscle fibers and lower proportions of insulin-sensitive Type I fibers near term. Am. J. Physiol. Regul. Integr. Comp. Physiol. 310, R1020–1029. doi: 10.1152/ajpregu.00528.2015
Yates, D. T., Green, A. S., and Limesand, S. W. (2011). Catecholamines mediate multiple fetal adaptations during placental insufficiency that contribute to intrauterine growth restriction: lessons from hyperthermic sheep. J. Pregnancy 2011, 740408. doi: 10.1155/2011/740408
Yates, D. T., Petersen, J. L., Schmidt, T. B., Cadaret, C. N., Barnes, T. L., Posont, R. J., et al. (2018). ASAS-SSR Triennial reproduction symposium: looking back and moving forward-how reproductive physiology has evolved: fetal origins of impaired muscle growth and metabolic dysfunction: lessons from the heat-stressed pregnant ewe. J. Anim. Sci. 7, 2987–3002. doi: 10.1093/jas/sky148
Zambrano, E., Guzman, C., Rodriguez-Gonzalez, G. L., Durand-Carbajal, M., and Nathanielsz, P. W. (2014). Fetal programming of sexual development and reproductive function. Mol. Cell. Endocrinol. 382, 538–549. doi: 10.1016/j.mce.2013.09.008
Keywords: in utero, intergenerational, nutrient restriction, postnatal, ruminant
Citation: Vautier AN and Cadaret CN (2022) Long-Term Consequences of Adaptive Fetal Programming in Ruminant Livestock. Front. Anim. Sci. 3:778440. doi: 10.3389/fanim.2022.778440
Received: 16 September 2021; Accepted: 29 March 2022;
Published: 25 April 2022.
Edited by:
Caleb Lemley, Mississippi State University, United StatesReviewed by:
Pedro Fontes, University of Georgia, United StatesCopyright © 2022 Vautier and Cadaret. This is an open-access article distributed under the terms of the Creative Commons Attribution License (CC BY). The use, distribution or reproduction in other forums is permitted, provided the original author(s) and the copyright owner(s) are credited and that the original publication in this journal is cited, in accordance with accepted academic practice. No use, distribution or reproduction is permitted which does not comply with these terms.
*Correspondence: Caitlin N. Cadaret, Y2FpdGxpbi5jYWRhcmV0QGNvbG9zdGF0ZS5lZHU=
Disclaimer: All claims expressed in this article are solely those of the authors and do not necessarily represent those of their affiliated organizations, or those of the publisher, the editors and the reviewers. Any product that may be evaluated in this article or claim that may be made by its manufacturer is not guaranteed or endorsed by the publisher.
Research integrity at Frontiers
Learn more about the work of our research integrity team to safeguard the quality of each article we publish.